DOI:
10.1039/C7QM00558J
(Research Article)
Mater. Chem. Front., 2018,
2, 609-614
Orbital-specific electronic interaction in crystalline films of iron phthalocyanine grown on Au(111) probed by angle-resolved photoemission spectroscopy
Received
6th December 2017
, Accepted 24th January 2018
First published on 26th January 2018
Abstract
The orbital-specific interfacial and intermolecular interactions in crystalline films of iron phthalocyanine (FePc) grown on an Au(111) surface were studied by means of angle-resolved photoemission spectroscopy (ARPES) with synchrotron radiation. The temperature-dependent ARPES measurement on the FePc monolayer reveals the formation of the molecule–metal hybrid state together with the Kondo resonance state below the Fermi level. These interface-specific states are derived from the electronic coupling of the Fe 3d orbital in the molecule with the Au(111) surface electron. For the flat-lying FePc multilayer on Au(111), the photon-energy-dependent ARPES spectra reveal the intermolecular valence-band dispersion for the C 2p-derived state, while the Fe 3d-derived state does not show such dispersion, indicating the localized Fe 3d spin state. These orbital-specific interactions play crucial roles in the unique electronic and magnetic properties of FePc.
Introduction
Metal phthalocyanine (MPc, M = metal) molecules are well-known archetypal organic semiconductors and show unique physical and chemical properties depending on central metals and end groups in the molecule and their polymorphs, which can be promising materials for next-generation (opto)electronics. Since MPc molecules with 3d transition metals (e.g. FePc, shown in the inset of Fig. 1) have attracted much attention for potential applications in molecular spintronic devices, various spectroscopic and microscopic experiments have been performed for MPc thin films and interfaces1–9 in comparison with gaseous molecules.10,11 In particular, it was pointed out that the FePc molecules on an Au(111) surface exhibit two Kondo resonances at the temperature of T = 2.6 ± 1.4 K and 110–150 K, depending on the adsorption configuration.3 The Kondo resonance is in general derived from the screening of the magnetic moment of the impurity spin by the conduction electrons as the result of the many-body entanglement.12 Therefore, the FePc–Au(111) hybrid state due to the orbital-specific interaction, if present, can be regarded as a probe to understand the Kondo resonance.
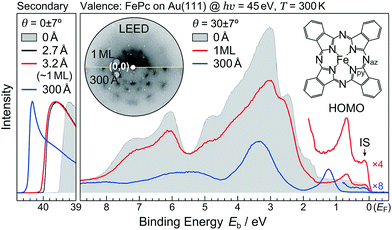 |
| Fig. 1 Electronic structure at 300 K; the Au(111) surface (gray shadow), the FePc monolayer on Au(111) (1 ML, red curve), and the FePc multilayer on Au(111) (300 Å, blue curve), measured by ARPES (hν = 45 eV, θ = 23°–37° integrated). The secondary electron cutoff region are shown in the left panel. The molecular structure of FePc and the combined LEED image (E = 60 eV) for the FePc monolayer and multilayer films on Au(111) at 20 K are shown in the inset. | |
In the present work, in order to understand the orbital-specific electronic interaction in two- and three-dimensional crystalline films of FePc on Au(111), we performed the angle-resolved photoemission spectroscopy (ARPES) experiments using synchrotron radiation. At the FePc/Au(111) interface, we observed evolution of the charge transfer (CT)-derived peak at the Fe site, together with the Kondo resonance at the temperature of T ≤ 160 K. On the other hand, we observed the intermolecular electronic band dispersion in the FePc multilayer on Au(111), which shows delocalized and localized characters for the C 2p- and Fe 3d-derived bands, respectively, and is related to magnetic properties of FePc thin films on Au(111).
Results and discussion
Molecule–metal hybrid state at the FePc/Au(111) interface
First, we discuss the thickness-dependent electronic structure of FePc thin films on Au(111). Fig. 1 shows the ARPES spectra (hν = 45 eV) and the corresponding low-energy electron diffraction (LEED) image, measured for the FePc thin films grown on Au(111). In the secondary electron cutoff region, which corresponds to the vacuum level (VL) position, the 2.7 Å deposition introduces the rapid decrease in VL from the clean Au(111) due to the formation of the interface dipole layer.13 Shift in VL for 3.2 Å is almost the same to that in 2.7 Å, indicating the saturated monolayer (1 ML) formation. The corresponding LEED image of the FePc monolayer exhibits two-kinds of diffraction spots; one is the
surface reconstruction pattern of Au(111) around the (0,0) spot, and the other is the weak diffraction pattern due to the molecular unit cell. The latter is the typical LEED pattern of the flat-lying MPc monolayer on Au(111).14 For the multilayer, the
pattern disappears and the molecule-derived LEED pattern is getting intense from the monolater's LEED pattern, indicating the ordered growth of the FePc multilayer on Au(111).
The ARPES spectrum of the FePc monolayer shows the highest-occupied molecular orbital (HOMO)-derived peak at the binding energy (Eb) of 0.68 eV. The HOMO-derived peak is shifted to the higher-Eb side as the thickness increases, and is almost converged at Eb = 1.23 eV when the film thickness reaches 300 Å. The thickness dependent shift in the HOMO is almost parallel to that in the VL, indicating that the ionization potential is almost constant in the present system; therefore, the observed shift in the HOMO is ascribed to the photohole screening by surrounding mediums such as the substrate and neighboring molecules.15 Note that, the interface-specific electronic state is observed just below the Fermi level (EF, Eb = 0 eV), labelled “IS” in Fig. 1, but is not observable in the FePc multilayer, which only shows the weak substrate's Fermi edge because of the dewetting effect on Au(111).16
The detailed θ-dependent ARPES spectra for the FePc monolayers on Au(111) and the highly-oriented pyrolytic graphite (HOPG) at 20 K are shown in Fig. 2(a). At the weakly physisorbed FePc/HOPG interface, the C 2p- and Fe 3d-derived peaks appear at Eb = 1.20 eV and 1.49 eV, respectively. The intensity of Fe 3d is stronger than that of C 2p at the surface-normal emission (θ = 0°). With increasing θ, the intensity of C 2p is getting stronger, and the peak intensity ratio between C 2p and Fe 3d is reversed at θ ≥ 20°. This photoelectron intensity angular distribution (PIAD) is reflected the different spatial electron distribution between C 2p- and Fe 3d-derived molecular orbitals. No additional adsorption-induced peaks can be seen just below EF at the FePc/HOPG interface.
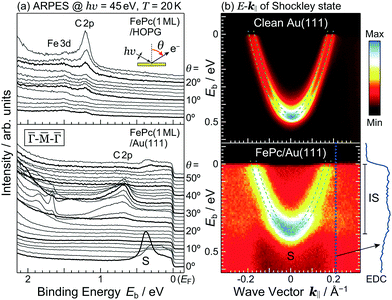 |
| Fig. 2 (a) θ-Dependent ARPES spectra (hν = 45 eV) for the FePc/HOPG and FePc/Au(111) interfaces at 20 K, shown with 2° step. (b) E–k‖ map of the Shockley state for the Au(111) surface and the FePc/Au(111) interface at 20 K. The green dashed lines represent the parabolic fitting to the observed dispersion. EDC at k‖ = 0.21 Å−1 is shown in the right-hand side. | |
At the FePc/Au(111) interface, the dispersive Au(111) surface states exist at Eb = 0–0.37 eV (Shockley state, labelled S), 1.65–2.15 eV (Tamm state), and 0–2.15 eV (sp band). The C 2p-derived peak is localized at Eb = 0.68 eV, and overlaps with the sp band at θ = 20°–30°. The C 2p PIAD shows an intensity maximum at θ = 32°, which is converted to the lateral wave-vector component (k‖) of ca. 1.7 Å−1 using the following equation.
| 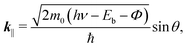 | (1) |
where
m0 is the free-electron mass and
Φ is the work function. Remarkably, no clear Fe 3d-derived peaks appear just below the C 2p-derived peak, indicating the presence of the non-negligible interfacial interaction at the Fe site with Au(111). Indeed, the interface state appears as a tiny signal just below
EF. In contrast to the C 2p-derived peak, the interface state is observable even around
θ = 0° (
k‖ = 0 Å
−1), together with the Shockley state as discussed below.
The Shockley state shows a parabolic dispersion in the E–k‖ map [Fig. 2(b)], obtained using eqn (1), which is well-reproduced by two free-electron-like parabolas,
| 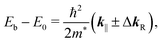 | (2) |
with the parameters given in
Table 1. The Shockley state at the FePc/Au(111) interface shows a weak modification with the upshift by Δ
E0 = 85 meV from that at the clean Au(111) surface, which is in general ascribed to the Pauli repulsion of surface electron systems. As demonstrated previously,
17,18 the adsorption-induced shift in the Shockley state enables us to determine the interfacial adsorption energy, 9.0 meV Å
−2 (=0.106 Δ
E0) at the FePc/Au(111) interface, which suggests a quite weak physisorptive interaction at the Pc-ring site.
18 Note that the Shockley-state modification during the 1 ML formation depends on the actual coverage.
19 In the present work, the modification is almost saturated as judged from the VL shift in
Fig. 1. On the other hand, in addition to the Shockley state, the interface state simultaneously appears at
Eb = 0–0.4 eV as judged from the energy distribution curve (EDC) analysis at
k‖ = 0.21 Å
−1, which is not observable at the FePc multilayer or the other MPc/Au(111) interfaces.
18 Considering the orbital-specific PIAD discussed in
Fig. 2(a), the most plausible contribution to the FePc/Au(111) interface state is the Fe 3d-derived orbital.
Table 1 Fitting parameters in eqn (2) for the Shockley state of Au(111) and FePc/Au(111) at 20 K in Fig. 2(b) (effective mass m*, surface state energy E0, and Rashba momentum offset ΔkR)
Sample |
m*/m0 |
E
0 (meV) |
ΔkR (Å−1) |
Au(111) |
0.27 |
465 |
0.013 |
FePc/Au(111) |
0.28 |
380 |
0.013 |
In order to understand the FePc/Au(111) interface state in more detail, we performed the temperature dependence of He Iα ARPES (hν = 21.218 eV) along θ = 55° (k‖ ≈ 1.7 Å−1). As shown in Fig. 3(a), the substrate's Fermi edge at Eb = 0 meV is sharpened with decreasing the temperature, which can be subtracted by using the Fermi–Dirac distribution function. In Fig. 3(b), the background-subtracted ARPES spectra exhibit the temperature-dependent evolution of the interface state. At T = 240 K, the interface state appears very weakly at Eb = 80–400 meV. The intensity of the interface state is increased at T = 180 K, and is almost saturated at T ≤ 160 K. Increase in the intensity of the interface state can be explained by the efficient CT interaction from Au(111) to Fe 3d orbitals due to the shorter FePc–Au(111) interfacial bonding distance at the lower temperature. Remarkably, an additional interface state appears just below EF (Eb ≈ 30 meV) at T ≤ 160 K. The additional interface state just below EF can be ascribed to the Kondo resonance since the observed energy and temperature range correspond well with those for the high-temperature Kondo resonance at the FePc/Au(111) interface;3 therefore, the complex electronic interaction at the FePc/Au(111) interface is mainly dominated by the Fe 3d orbitals in the FePc molecule coupled with the Au(111) surface electron systems. Although we could not apply the lineshape analysis to the Kondo-resonance peak because of its tiny signal intensity, the ARPES spectra show the evidence for the linewidth narrowing with decreasing the temperature, which is apparent at T = 60–100 K, as a result of the electron–phonon interaction.6
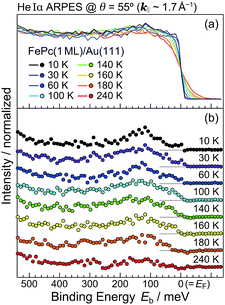 |
| Fig. 3 Temperature-dependent He Iα ARPES spectra at θ = 55° (hν = 21.218 eV, k‖ = 1.7 Å−1) for the FePc/Au(111) interface. (a) Raw ARPES spectra. (b) Background-subtracted ARPES spectra using the Fermi–Dirac distribution function. | |
Intermolecular interaction in the FePc crystalline film
As discussed using the LEED data (Fig. 1), the FePc molecules on Au(111) form the ordered growth, which introduces the ferromagnetism.8 Prior to discussion of the intermolecular interaction in the FePc crystalline film, the molecular orientation of the FePc crystalline film on Au(111) at 20 K was quantitatively characterized by the incident-angle (α) dependence of N K-edge X-ray absorption spectroscopy (XAS). The XAS spectra in Fig. 4 show sharp peaks at hν = 398.1 eV, 400.2 eV, and 402.1 eV as labelled A, B, and C, respectively. Peak A is ascribed to the 1s → LUMO transition at azaporphyrin N (Naz) site, and peaks B and C are ascribed to the 1s → π* transitions at Naz and pyrrole N (Npy) sites.20 Peaks A–C are weakest at the normal incidence (α = 0°) and become stronger with increasing α. Broad 1s → σ* features appear after the isosbestic point at hν = 405 eV, showing the opposite behavior. These evidences are explained by the interaction between the electric field vector (E) of incident p-polarized X-rays and the molecular orbitals based on the dipole transition. The XAS intensity is expressed as, | 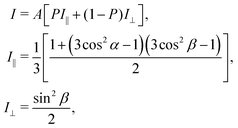 | (3) |
where A, P, and β are constants for the absolute intensity, the polarization degree of the incident X-ray (0 ≤ P ≤ 1), and a molecular tilt angle with respect to the substrate surface, respectively.21 From the comparison between the angular distribution of the XAS peaks A–C and the theoretical curves using eqn (3) with P = 0.90, we conclude that FePc molecules on Au(111) form a flat-lying orientation with β = 0°–10°, as is consistent with the LEED data. On the other hand, peak X at hν = 398.8 eV shows a slightly different α dependence due to the hybridization between the N 2p and Fe 3d orbitals as has been observed for other MPc thin films such as MnPc,22 which gives different transition dipole moments for the incident X-rays.
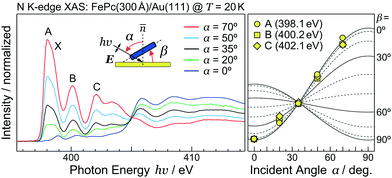 |
| Fig. 4 Incident angle (α) dependence of the N K-edge XAS spectra for the FePc multilayer on Au(111) at 20 K. The XAS intensity plots with the theoretical curves with various molecular tilt angles (β) are shown. | |
In the case of the flat-lying ordered multilayer of π-conjugated molecules, the intermolecular electronic coupling exists along the surface-normal direction, and can be detected by the hν-dependent normal-emission ARPES, which scans the perpendicular wave-vector component (k⊥).
| 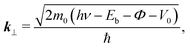 | (4) |
where
V0 is the inner potential.
Fig. 5(a) shows the
hν dependence of the normal-emission ARPES spectra for the FePc crystalline film at 20 K. As pointed out in
Fig. 1, the observed ARPES spectra involve the weak substrate's Fermi edge at
Eb = 0 eV, which seems to be stronger than that observed at 300 K and
θ = 23°–37° due to the temperature-induced sharpening and the different surface/bulk sensitivity depending on
θ. We used the Fermi edge signal for the
hν calibration. As shown in the inset of
Fig. 5(a), the topmost peak around
Eb = 1.5 eV exhibits two components with the energy separation by
ca. 0.4 eV. Judging from
Fig. 2(a) and the gas-phase FePc photoemission,
10 the observed low- and high-
Eb components can be ascribed to the C 2p and Fe 3d orbitals, respectively. The intensity of C 2p and Fe 3d is dependent on
hν. The C 2p peak is stronger than the Fe 3d peak at
hν ≤ 54 eV, and their relative intensity is flipped at
hν ≥ 58 eV due to the different photoionization cross section between C 2p and Fe 3d orbitals. Note that, the
hν-dependent intensity ratio between C 2p and Fe 3d in the FePc crystalline film is slightly different from that in the gaseous FePc,
10 originating from the presence of the intermolecular orbital interaction.
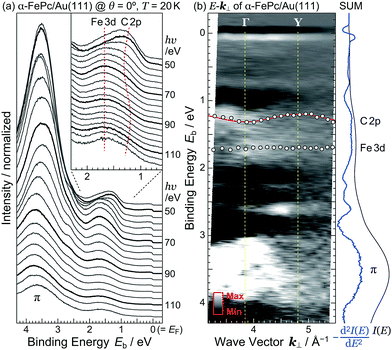 |
| Fig. 5 Intermolecular valence-band dispersion in the α-FePc crystalline film on Au(111) at 20 K. (a) hν-Dependent normal-emission ARPES spectra, shown with hν step of 4 eV. The HOMO-derived peak is magnified in the inset. (b) E–k⊥ map obtained from the second derivatives of the hν-dependent ARPES spectra [−d2I(E)/dE2], where open circles represent the peak position of C 2p and Fe 3d bands. The tight-binding fitting to the C 2p band is indicated by the red curve. The sum curves for the raw ARPES [I(E)] and −d2I(E)/dE2 spectra are shown on the right. | |
Judging from the lineshape of the HOMO-derived peak, the C 2p peak shows a dispersive behavior with hν, but the Fe 3d peak does not. The C 2p peak appears at Eb = 1.25 eV at hν = 50 eV. With increasing hν, the C 2p peak shifts to the higher-Eb side, and turns back at hν = 62 eV. Such an inflection point is also found around hν = 94 eV. The HOMO-derived peak onset also shows the dispersive behavior with hν. If the observed change in the ARPES spectra originates from the hν-dependent photoionization cross section, the HOMO-derived peak onset should appear at the constant Eb position.10 Since the C 2p peak and its onset shows the periodic shift, the most plausible origin of the dispersive behavior is not photoionization cross section but the delocalized band formation.
Fig. 5(b) shows the E–k⊥ map for the FePc crystalline film on Au(111) at 20 K, which is obtained from the second derivative of ARPES [−d2I(E)/dE2] after converting to k⊥ from hν using eqn (3). For the k⊥ conversion, we used V0 = −1.0 eV, to get good agreement in the dispersion periodicity between C 2p and π bands. The dispersion periodicity is well explained by π/a⊥ = 0.964 Å−1, where a⊥ = 3.26 Å is the intermolecular distance. Because of the low-temperature measurement in ARPES, the determined a⊥ = 3.26 Å at 20 K is 4.1% shorter than that observed for the α-phase FePc crystal, as determined from the room-temperature X-ray diffraction (a⊥ ≈ 3.4 Å).23 The observed dispersion for the C 2p peak can be thus ascribed to the delocalized band formation due to the intermolecular π–π interaction in the α-FePc crystalline film as observed for the other MPc crystalline films.24,25 From the Γ to Y point, the C 2p band is dispersed to the lower-Eb side by 110 ± 5 meV in total. Assuming the free-electron-like final states, the one-dimensional tight-binding model with only nearest neighbor interactions is given as,
| E(k⊥) =E0b − 2t⊥ cos(a⊥k⊥), | (5) |
where
E0b is the dispersion center and
t⊥ is the transfer integral. A least-squares fitting to the C 2p band dispersion using
eqn (5), as shown by a red solid curve in the
E–
k⊥ map, gives
E0b = 1.257 eV and
t⊥ = 28 meV. Using the dispersion parameters of
a⊥ and
t⊥, the hole effective mass (
mh*) of the C 2p-derived band is determined by 12.8
m0, giving the lower limit of the hole mobility (
μh) of 23.4 cm
2 V
−1 s
−1 for the α-FePc crystal at 20 K by using
μh > 20(
m0/
mh*) (300/
T).
26 On the other hand, the Fe 3d band show non-dispersive behavior, indicating the localization of the Fe 3d state at
Eb = 1.7 eV as the origin of the ferromagnetism in the α-FePc crystal.
Conclusion
We have studied the orbital-specific electronic interaction in the monolayer and multilayer films of FePc on Au(111) by means of ARPES, wherein the molecular orientation and the ordered structure were characterized by XAS and LEED. The temperature-dependent ARPES measurement on the FePc monolayer reveals the formation of the interface-specific state derived from the site-specific electronic coupling at the central-metal (Fe) site in the FePc molecule with the Au(111) surface electron system. The orbital-specific CT interaction induces not only the filling of Fe 3d-derived unoccupied orbitals but also the Kondo resonance.
The hν-dependent ARPES (E–k⊥) measurement on the FePc multilayer reveals the orbital-specific intermolecular interaction. The weakly delocalized C 2p band due to the intermolecular π–π interaction gives the hole transport properties mh* = 12.8m0 and μh > 23.4 cm2 V−1 s−1 at 20 K. On the other hand, the Fe 3d band does not show dispersion, indicating the localized character of the spin state. The interplay of the orbital-specific electronic interactions is crucial in the coexistence of high-μh and ferromagnetic characters of FePc crystals.
Experimental
ARPES and XAS experiments
The ARPES and XAS experiments were performed on a highly brilliant in-vacuum undulator beamline of BL6U, which covers hν = 40–700 eV, of the UVSOR-III Synchrotron in the Institute for Molecular Science. The ARPES/XAS endstation at BL6U consists of the measurement, preparation, and load-lock chambers. The ARPES spectra were acquired from the energy-vs.-angle image by using a micro-channel-plate (MCP) detector of a hemispherical electron energy analyzer (MB Scientific A-1). The total energy resolution was 20 meV and the angular resolution was 0.2° in the ARPES measurements. On the other hand, the XAS spectra were measured by using the sample-current method with the energy resolution of better than 0.1 eV.
The temperature-dependent He Iα ARPES experiment (hν = 21.218 eV) was performed using the laboratory-based ARPES system, equipped with the deflector-type hemispherical analyser and the monochromatized UV source with a μ-spot capillary (Scienta-Omicron DA30 and VUV5k, respectively). The sample temperature was controlled by the liquid He flow cryostat and the indirect resistive heating systems.
Preparation of the FePc thin films on Au(111)
The Au(111) single crystal (5 N purity) and the HOPG (ZYA grade) were used for the substrate. The clean Au(111) substrate was obtained by repeated cycles of the Ar+ sputtering and the subsequent annealing at 700 K. The HOPG substrate was cleaved in air just before loading into the load-lock chamber and cleaned by heating at 700 K for 5 hours in the preparation chamber. The cleanliness of these substrates was confirmed by the core-level and valence-band ARPES spectra and MCP-LEED.
The well-ordered FePc monolayer was prepared by the vacuum deposition of highly purified powders onto the clean substrate kept at the room temperature. After the 1 ML equivalent deposition, the sample was annealed at 475 K to improve the lateral ordering. The thick FePc multilayer was prepared on the well-ordered FePc monolayer. During the deposition for the FePc multilayer, the substrate temperature was kept at 355 K. The deposition rate (<1 Å min−1) and amount were measured using a calibrated quartz crystal microbalance. The lateral ordered structure of FePc crystalline films was confirmed by MCP-LEED.
Conflicts of interest
There are no conflicts to declare.
Acknowledgements
The authors would like to thank the staff of the UVSOR-III Synchrotron for their kind supports in the present ARPES experiment. This work is supported by Grant-in-Aid for Scientific Research on Innovative Areas (π-System Figuration: Control of Electron and Structural Dynamism for Innovative Functions) JP17H05167 and Grant-in-Aid for Scientific Research (C) JP17K05767 from Japan Society for the Promotion of Science (JSPS) and Grant for Basic Science Research Projects from the Sumitomo Foundation 150301.
References
- A. Zhao, Q. Li, L. Chen, H. Xiang, W. Wang, S. Pan, B. Wang, X. Xiao, J. Yang, J. G. Hou and Q. Zhu, Science, 2005, 309, 1542 CrossRef CAS PubMed.
- N. Tsukahara, S. Shiraki, S. Itou, N. Ohta, N. Takagi and M. Kawai, Phys. Rev. Lett., 2011, 106, 187201 CrossRef PubMed.
- E. Minamitani, N. Tsukahara, D. Matsunaka, Y. Kim, N. Takagi and M. Kawai, Phys. Rev. Lett., 2012, 109, 086602 CrossRef PubMed.
- N. Tsukahara, E. Minamitani, Y. Kim, M. Kawai and N. Takagi, J. Chem. Phys., 2014, 141, 054702 CrossRef PubMed.
- Y. Wang, X. Zheng and J. Yang, Phys. Rev. B, 2016, 93, 125114 CrossRef.
- J. Ziroff, S. Hame, M. Kochler, A. Bendounan, A. Schöll and F. Reinert, Phys. Rev. B: Condens. Matter Mater. Phys., 2012, 85, 161404(R) CrossRef.
- S. Ahmadi, M. N. Shariati, S. Yu and M. Göthelid, J. Phys. Chem., 2012, 137, 084705 CrossRef PubMed.
- J. Bartolomé, F. Bartolomé, L. M. García, G. Filoti, T. Gredig, C. N. Colesniuc, I. K. Schuller and J. C. Cezar, Phys. Rev. B: Condens. Matter Mater. Phys., 2010, 81, 195405 CrossRef.
- S. Stepanow, P. S. Miedema, A. Mugarza, G. Ceballos, P. Moras, J. C. Cezar, C. Carbone, F. M. F. de Groot and P. Gambardella, Phys. Rev. B: Condens. Matter Mater. Phys., 2011, 83, 220401(R) CrossRef.
- B. Brena, C. Puglia, M. de Simone, M. Coreno, K. Tarafder, V. Feyer, R. Banerjee, E. Göthelid, B. Sanyal, P. M. Oppeneer and O. Eriksson, J. Chem. Phys., 2011, 134, 074312 CrossRef PubMed.
- I. Bidermane, J. Lüder, R. Totani, C. Grazioli, M. de Simone, M. Coreno, A. Kivimäki, J. Åhlund, L. Lozzi, B. Brena and C. Puglia, Phys. Status Solidi B, 2015, 252, 1259 CrossRef CAS.
- J. Kondo, Prog. Theor. Phys., 1964, 32, 37 CrossRef CAS.
- H. Ishii, K. Sugiyama, E. Ito and K. Seki, Adv. Mater., 1999, 11, 605 CrossRef CAS.
- B. Stadtmüller, I. Kröger, F. Reinert and C. Kumpf, Phys. Rev. B: Condens. Matter Mater. Phys., 2011, 83, 085416 CrossRef.
- H. Peisert, M. Knupfer, T. Schwieger, J. M. Auerhammer, M. S. Golden and J. Fink, J. Appl. Phys., 2002, 91, 4872 CrossRef CAS.
- G. Beernink, T. Strunskus, G. Witte and C. Wöll, Appl. Phys. Lett., 2004, 85, 398 CrossRef CAS.
- J. Ziroff, P. Gold, A. Bendounan, F. Forster and F. Reinert, Surf. Sci., 2009, 603, 354 CrossRef CAS.
- H. Yamane and N. Kosugi, J. Phys. Chem. C, 2016, 120, 24307 CAS.
- E. Annese, J. Fujii, I. Vobornik and G. Rossi, J. Phys. Chem. C, 2011, 115, 17409 CAS.
- S. Kera, M. B. Casu, A. Schöll, T. Schmidt, D. Batchelor, E. Rühl and E. Umbach, J. Chem. Phys., 2006, 125, 014705 CrossRef CAS PubMed.
-
J. Stöhr, NEXAFS Spectroscopy, Springer-Verlag, 1992 Search PubMed.
- F. Petraki, H. Peisert, F. Latteyer, U. Aygül, A. Vollmer and T. Chassé, J. Phys. Chem. C, 2011, 115, 21334 CAS.
- M. Evangelisti, J. Bartolomé, L. J. de Jongh and G. Filoti, Phys. Rev. B: Condens. Matter Mater. Phys., 2002, 66, 144410 CrossRef.
- H. Yamane and N. Kosugi, Phys. Rev. Lett., 2013, 111, 086602 CrossRef PubMed.
- H. Yamane and N. Kosugi, J. Chem. Phys., 2014, 141, 224701 CrossRef PubMed.
- N. Ueno and S. Kera, Prog. Surf. Sci., 2008, 83, 490 CrossRef CAS.
Footnote |
† Present address: RIKEN SPring-8 Center, Sayo, Hyogo 679-5148, Japan. E-mail: yamane@spring8.or.jp |
|
This journal is © the Partner Organisations 2018 |
Click here to see how this site uses Cookies. View our privacy policy here.