DOI:
10.1039/C7QM00556C
(Research Article)
Mater. Chem. Front., 2018,
2, 402-409
Smart soaps: stimulus responsive soap–hydrogel bead composites for controlled dissolution and release of actives†
Received
4th December 2017
, Accepted 5th January 2018
First published on 16th January 2018
Abstract
We designed pressure responsive soap–hydrogel bead composites by incorporating agar hydrogel beads of different size distributions within a molten soap matrix at various volume fractions. Upon cooling, the combined suspension of hydrogel beads into the molten soap was set into a composite of soap matrix. We demonstrate pressure driven syneresis of water from the soap–hydrogel bead composites upon compression. This allowed a release of active components embedded in the hydrogel beads upon application of pressure on these “smart” soap composites. We found that the dissolution rate of these composites generally increases with the volume percentage of hydrogel beads. We achieved a composite dissolution rate approximately 2.8 times higher than the soap control sample without hydrogel beads. However, the composite dissolution rate was independent of the size of the embedded hydrogel beads. We studied the release rates of active components encapsulated within the hydrogel beads used to prepare the composites. It was found that the release rate can be controlled in three different ways: varying the hydrogel beads size, using different concentrations of the gelling polymer used to make the hydrogel and also by co-encapsulating an oppositely charged polyelectrolyte along with the active encapsulated species. We found that the composites compressional strength decreased with an increasing volume percentage of hydrogel beads incorporated within the soap composite. Young's modulus showed a maximum when 7.5% by volume of hydrogel beads were used for composite preparation. These fast-dissolving soap–hydrogel composites contain significantly less raw materials and would reduce the pollution of waste water with surface active components. We envisage that soap–hydrogel bead composites could improve the sustainability of the soap-producing industry and could find their application within the hotel business, where they could reduce costs and the waste of millions of partially used soap bars discarded on a daily basis.
Introduction
The use of soap products is considered essential for modern life when it comes to personal hygiene and disease prevention. For example, one of the leading causes of child death around the world is diarrhoeal disease, with estimates that more than 2.2 million lives are lost each year due to these infections.1 Handwashing is a preventative measure that may substantially reduce the chances of contracting diarrhoeal diseases. It has been shown that handwashing with soap and water reduces bacteria present on hands to 8% which is almost 3 times lower than handwashing with water alone.2 Therefore, this necessitates cheap and easy worldwide access to soap products. The Global Soap Project organisation has estimated that 2.6 million bars of soap are discarded each day by the hotel industry in the U.S. alone. This occurs due to hotel guests using only a fraction of their soap bars before they leave, followed by the hotels discarding these partially used bars to replace them with fresh ones for their next guests. Not only is this wasteful, it also incurs unnecessary costs. Furthermore, discarding such large amounts of soap is detrimental to the environment. Many surface active components are harmful to aquatic life, pollute water and can endanger human health.3,4 The development of methods to decrease the production costs of soap is a crucial step towards making it more readily accessible worldwide; which in turn could reduce mortality rates due to certain bacterial infections, especially in developing countries.
Hydrogels are 3D hydrophilic, polymeric networks that can be considerably swollen with water. They are largely biocompatible and are aqueous-based which has made them useful in various areas such as porogenic materials,5 drug delivery and wound dressing,6 tissue engineering7 and food structuring.8–10 Porous materials fabricated from hydrogel templating of gypsum composites have been studied as sound and heat insulating materials.11,12
Here, we have explored the incorporation of hydrogel beads within soap as a method to reduce costs, increase sustainability of the sourced materials and decrease pollution of waste waters with surface active materials. We have used agar as gelling agent, which is derived from natural sources and consists of both agarose and agaropectin, to produce the hydrogels. It is insoluble in cold water, however it hydrates at temperatures close to the boiling point of water. At such temperatures, the polymer chains adopt a random coil conformation. Upon cooling, the agarose chains form double helixes which then self-assemble into a three-dimensional network with water within.13 Once set, the hydrogel would not melt at temperatures below 85 °C,14 which exceeds the melting point of many conventional soaps.
Upon blending of the hydrogel, slurries of hydrogel beads were obtained. We have mixed these slurries of hydrogel beads with molten soap at temperature below the melting point of the hydrogel but above the soap melting point to obtain soap–hydrogel composites with controlled composition. The produced soap–hydrogel composites showed an increase in their dissolution rate depending on the hydrogel bead content. The presence of hydrogel beads within the composites also allows for control over a range of their properties. One can change the size of the hydrogel beads or the concentration of the gelling polymer to control the release rate of active species encapsulated within the beads. The release rate of encapsulated species can also be controlled by encapsulating different amounts of oppositely charged polyelectrolyte which is expected to delay their diffusion out of the beads during the composite dissolution. Investigation of the mechanical properties of the produced composites showed that their compressional strength decreased with increasing volume percentage of hydrogel beads used in the composite preparation. The Young's modulus of the composites, however, displayed a maximum when small amounts of hydrogel beads were incorporated within the soap matrix. An unusual behaviour of the composites was observed when they underwent compression; syneresis of water occurred. This suggests that they could be useful for designing a washing action in areas where clean water is not readily available. We also expect that this effect can be beneficial for better consumer perception when washing with such a composite soap bar.
We foresee the use of such soap–hydrogel composites in the hotel industry, where millions of barely used soap bars are discarded each day. The reduced amount of surface active species present in them would lead to a decrease in pollution of waste water. Finally, the decreased cost due to a reduction in raw materials required for production could make them attractive for businesses and more affordable for developing countries.
Experimental
Materials
Agar (food grade) was purchased from Special Ingredients Ltd. A soap base (main components were sodium stearate, glycerol and water) was purchased from a local shop. Berberine hydrochloride (BRB, 98%) was purchased from Sigma-Aldrich. Methylene blue (MB) was obtained from Lancaster Synthesis Ltd. Poly(sodium 4-styrenesulfonate) (PSS) with a weight average molecular weight (Mw) of ca. 70
000 g mol−1 was purchased from Sigma-Aldrich. Deionised water was obtained by a MilliQ purification system (Millipore) and used in all experiments.
Production of hydrogel beads
Agar hydrogels (2.0% or 8.0% w/v) were prepared by addition of the agar powder to water in a sealable bottle. The bottle was sealed, autoclaved (Classic prestige medical autoclave, 121 °C, 105 kPa) to allow hydration of the agar and then homogenised whilst hot. This was repeated twice to ensure complete dissolution of the agar. The hydrogel was then left to set at room temperature and placed in a fridge (4 °C) overnight. To produce ‘large’ hydrogel beads, the hydrogel was transferred to a blender (Tefal food processor Minipro with 500 W power and three stacked blades) and blended for 10 seconds to produce a slurry of beads with an average diameter of 600 ± 300 μm. To produce ‘small’ hydrogel beads, the hydrogel was blended for 300 seconds (see video S1 in ESI†). The resulting slurry contained beads with an average diameter of 120 ± 60 μm.
Preparation of soap–hydrogel bead composites
Soap base was cut into pieces and heated in a thermostatic bath at 75 °C until it melted. The molten soap was mixed with controlled volume percentages of the slurry of hydrogel beads (either small or large).
Five different volume percentages of slurry of hydrogel beads were used (7.5%, 15%, 25%, 35% and 50%) as well as a control sample of soap alone. The samples prepared for the dissolution studies were then degassed under vacuum in a desiccator and poured into moulds. After setting in a fridge (4 °C) for 1 hour, the samples were removed from the moulds for testing (see video S2 in ESI†). A schematic illustrating this procedure is shown in Fig. 1. For the composites prepared for the release kinetics studies, molten soap base and slurries of hydrogel beads were mixed gently with a spatula for 15 seconds to limit the formation of air bubbles. After mixing, aliquots were poured into pre-cooled moulds (4 °C), ensuring rapid setting of the composites. This limits the leaking of the aqueous phase from the gel beads into the liquid soap. The samples were set at 4 °C for 1 hour before use.
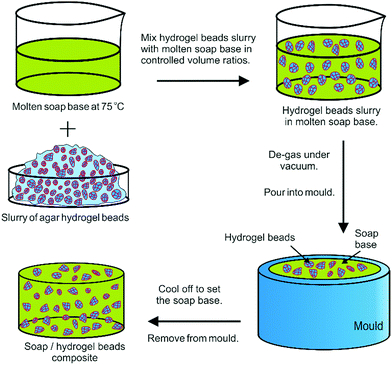 |
| Fig. 1 Schematics of the formulation of soap–hydrogel beads composites. Slurries of hydrogel beads are mixed with molten soap base in controlled volume ratios. The mixture was de-gassed to remove trapped air bubbles, poured into moulds and then transferred to a fridge (4 °C) for 1 hour to set before further use. | |
Dissolution kinetics of soap–hydrogel bead composites
We investigated how varying the volume percentage of hydrogel beads incorporated within the soap composites affected their dissolution rate compared to the control sample of soap alone. Cylindrical samples of the same dimensions, initial surface area and weight of approximately 15 g each were placed into a sample then submerged in water (750 cm3, 30.0 ± 0.2 °C). The holder consisted of a metal wire basket designed in-house to allow maximum exposure of the sample to warm water (see video S4 in ESI†). Dissolution was encouraged via agitation with a magnetic stirrer bar (35 mm length, 6 mm width) rotating on a magnetic stirrer at 600 rpm. The conductance of the solution as a function of time passed since the sample was added to water was measured using a Jenson 4510 Bench Conductivity Meter. Care was taken to have the sample and the probe in the same position in each measurement. The conductance was compared with a calibration curve to elucidate the dissolution rate over a period of 120 seconds.
Release rates of actives from soap–hydrogel bead composites
Berberine (BRB) has recently been investigated as a natural antimicrobial agent.23,24 BRB solution (0.15% w/v) was gelled with agar (either 2.0% w/v or 8.0% w/v) using the same method as described previously. Here we also used MB as a model for a cationic active due to the easy way of monitoring its release based on UV/vis absorbance. The MB aqueous solution was mixed with PSS solution so that the overall concentrations were 0.01 M MB and either 0.1% or 0.25% w/v PSS, respectively. This solution was gelled using agar (2.0% w/v). The resulting hydrogels were then blended for either 10 seconds or 300 seconds to produce slurries of large or small beads. Then, the soap–hydrogel composites were prepared with 50% volume percentage of hydrogel beads. Composite samples of approximate initial weight of 15 g each were immersed in water (200 cm3, 30.0 ± 0.2 °C) whilst being agitated with a magnetic stirrer bar at 600 rpm. Aliquots from the surrounding solution were taken at certain time intervals and their UV-visible spectra measured to obtain the release rate with the utmost care to take them from the same position each time (see video S4 in ESI†). The effect of the hydrogel bead size, agar concentration in the hydrogel and the concentration of an oppositely charged polyelectrolyte (PSS) on the release rate of encapsulated compounds was investigated.
Mechanical properties
The samples were placed onto a Lloyds LS100 testing apparatus equipped with a 100 kN load cell and a preload of 10 N was applied. They were compressed at a loading rate of 4 mm min−1 and their compressional strength was taken as the force applied at point of structural failure, normalised with the cross-sectional area of the sample. Young's modulus was obtained from the gradient of the linear elastic region of the stress/strain plots. Composites produced with 5%, 7.5% and 12% by volume of hydrogel beads were also tested.
Results and discussion
Hydrogel bead size distributions
We controlled the size of the hydrogel beads by varying the blending time of the hydrogel. The hydrogel beads were then dispersed in water, viewed under optical microscopy and their size distributions measured using Image J software. It was found that after blending the hydrogel for 10 seconds, the beads produced had an average size of 600 ± 300 μm, whereas after blending for 300 seconds, beads with an average size of 120 ± 60 μm were obtained. The cumulative distributions of the average bead diameters can be seen in Fig. 2. Hydrogel beads of irregular shape were produced due to the preparation method used, therefore the average of the length measured through the widest section of each bead was determined.
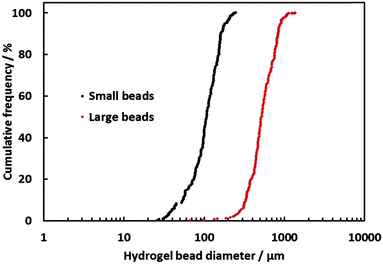 |
| Fig. 2 Percentage cumulative distribution of agar hydrogel (2.0% w/v) bead sizes for small beads produced by blending for 300 seconds and for large beads produced by blending for 10 seconds. | |
Dissolution rate of soap–hydrogel composites
An investigation into the kinetics of dissolution of the soap–hydrogel composites was performed by submerging the sample into a fixed volume of water whilst stirring with a magnetic stirrer (see video S4 in ESI†).
The solution conductance was recorded after 120 seconds and used to calculate the initial dissolution rate by comparing with a calibration curve (Fig. 3). The initial dissolution rates at the test conditions were 0.00093 ± 0.00005 g s−1 for the pure soap base and up to 0.0026 ± 0.0001 g s−1 for the soap–hydrogel composites. Fig. 3C shows that the solution conductance is proportional to the soap concentration. It can be seen that the composites produced with greater volume percentage of hydrogel slurry had increased dissolutions rates and therefore would be faster acting soaps. When the volume of hydrogel beads slurry incorporated within the soap matrix was 50%, the dissolution rate increased by approximately 2.8 times compared with the control sample (soap base). This is possibly due to the hydrogel beads being removed from the surface of the composites due to the drag forces acting upon them. After the detachment of the hydrogel beads, the surface area of the soap exposed to water will increase which in turn will increase the dissolution rate. A schematic to show this process is shown in Fig. 4. In addition, the soap near the hydrogel beads could be partially hydrated within the composite and could dissolve faster.
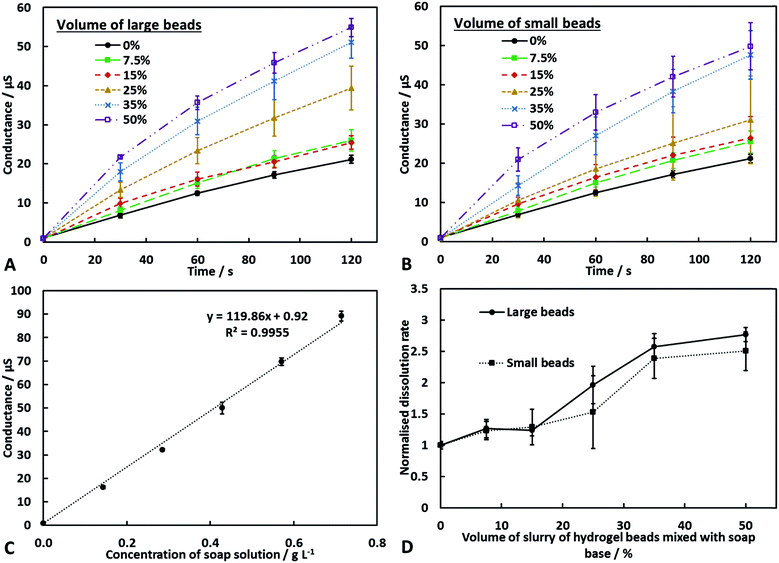 |
| Fig. 3 (A and B) The change in conductance over time when dissolving soap and soap hydrogel composites in water (750 cm3, 30 ± 0.2 °C). (C) The conductance versus the concentration of soap solution prepared under the same conditions. (D) The dissolution rate of the soap hydrogel composites after normalising with the dissolution rate of a control sample of soap alone. Each data point represents an average of three separate samples and the error bars are the standard deviation. | |
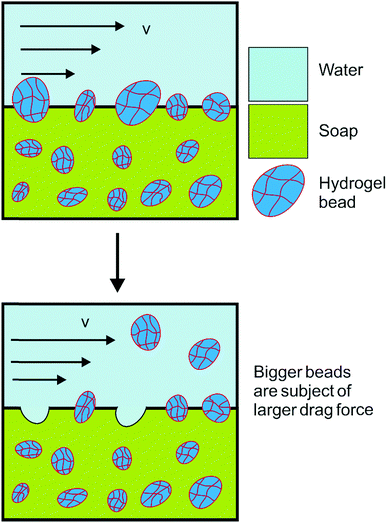 |
| Fig. 4 Schematics explaining the increase of the sample dissolution rate with increasing volume percentage of slurry of hydrogel beads incorporated within the soap–hydrogel composite. As the flow detaches hydrogel beads from the surface of the dissolving soap–hydrogel composites, the sample surface area increases which increases further the dissolution rate. | |
Role of hydrogel bead size on the release rate of berberine (BRB) from soap–hydrogel composites
We have investigated the effect of the average size of the hydrogel beads used on the release rate of BRB encapsulated within the beads. Soap hydrogel beads composites containing 50% by volume of large or small hydrogel beads with berberine encapsulated within them were prepared and investigated (see video S4 in ESI†). Upon dissolution of the composites, aliquots of the dissolution medium were taken and their UV-visible spectra was measured to determine the amount of BRB released. The results are shown in Fig. 5. The soap–hydrogel composites produced using small hydrogel beads showed up to approximately a twice as fast release rate of BRB after 240 seconds, when compared to the soap composites produced with large hydrogel beads. This can be attributed to a larger surface area to volume ratio of the small beads compared to large beads and decreased diffusion path lengths and increased BRB concentration gradients when using small beads.15–21
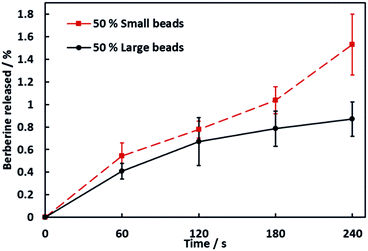 |
| Fig. 5 Release rate of BRB when dissolving soap hydrogel composites in water (200 cm3). Samples initial weight was approximately 15 g each. 50% by volume of agar (2.0% w/v) hydrogel beads were incorporated in the composites and the initial concentration of BRB in the hydrogel beads was 0.15% w/v. Each data point is an average of three results and the error bars are the standard deviation. | |
Role of the agar concentration on the release rate of BRB
We investigated how the concentration of the agar used to prepare the hydrogel beads affected the release rate of actives from the soap–hydrogel composites. This was done by encapsulating BRB within hydrogel beads of different size distributions and different concentrations of agar and using them to prepare the soap–hydrogel composites.
Aliquots of the dissolution medium were taken during dissolution process and their UV-visible spectra measured and used to determine the release rate of BRB. The results are shown in Fig. 6A and B for both large and small beads, increasing the concentration of agar used to prepare the hydrogel beads causes a decrease in the release rate of BRB from the soap–hydrogel composites. This is likely due to the higher concentration of agar forming a denser polymer network with reduced porosity and increased stiffness which hinders the diffusion transport of BRB molecules encapsulated within the hydrogel beads22 (see Fig. S1 in the ESI† for the morphology of the agar gel structure of freeze-dried samples).
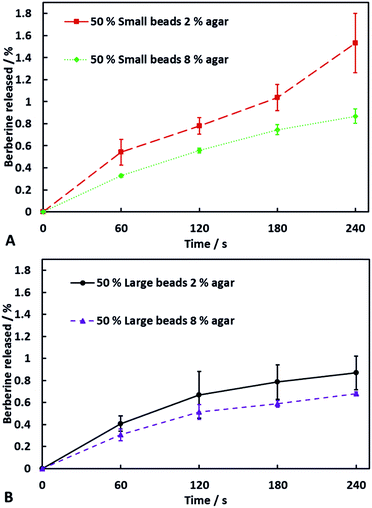 |
| Fig. 6 The release rate of BRB from soap–hydrogel composites (initial weight 15 g) when dissolving in water (200 cm3). The composites were produced using either small (A) or large (B) hydrogel beads prepared with different concentrations of agar (2.0% or 8.0% w/v). 50% by volume of hydrogel beads were incorporated within the composites and the initial concentration of BRB in the hydrogel beads was 0.15% w/v. Each data point is an average of three measurements and the error bars represent the standard deviation. | |
One could relate the data for the antimicrobial action of the released BRB to its concentration in the solution as recently reported in ref. 24 for E.coli.
Effect of polyelectrolyte co-encapsulation on the release rate of encapsulated actives from the soap–hydrogel composites
Another method we used to control the release rate of molecules encapsulated within our soap–hydrogel composites was to encapsulate a polyelectrolyte that has an opposite charge to the encapsulated active ingredient. We utilised PSS, a negatively charged polyelectrolyte, at two different concentrations; one being not in excess and the other being in excess of the cationic active, MB. From Fig. 7A and B, one can see how the release rates depend on the concentration of PSS encapsulated within the hydrogel beads. When small beads were used to prepare the composites, the initial amounts of MB released were independent of the PSS concentration. For times greater than 30 seconds, the composites containing excess PSS showed a slower release rate than those with PSS not in excess. For the composites produced using large hydrogel beads, the effect was seen instantly. The possible reasons for the reduction in the release rate of MB when PSS was in excess are two-fold: firstly, the presence of the polyelectrolyte reduces the free volume within the hydrogel beads, hindering transport of the MB molecules and secondly, there would be ionic attractions between negatively charged PSS and positively charged MB thus decreasing the concentration of free MB and its release rate.
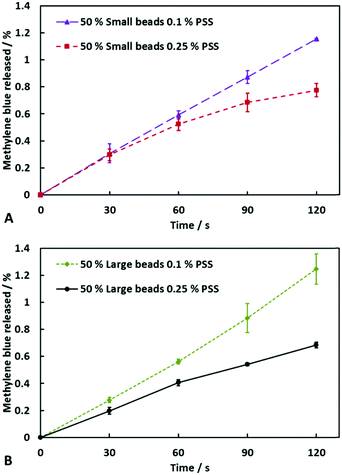 |
| Fig. 7 The release rate of MB from soap–hydrogel composites (initial weight 15 g each) when dissolving in water (200 cm3). The composites were produced using either small (A) or large (B) hydrogel beads prepared with agar (2.0% w/v). 50% by volume of hydrogel beads loaded with 0.01 M MB and PSS (0.1% or 0.25% w/v) were incorporated within the composites. Each data point is an average of three results and the error bars represent the standard deviation. | |
Fitting the actives release profiles from soap–agar hydrogel composites by different kinetic models
We have considered three different drug release models (first order model, Higuchi model and Hixson–Crowell model – see ref. 25–27) which were used to fit the release profile of BRB or MB from the soap–hydrogel composites.
(a) First order model: ln(100 − Mt) = k1t + ln(100).
Here Mt is the cumulative percentage of released drug at time t and k1 is the first order release constant. In this case, the amount of drug released at time t is proportional to the residual drug inside the drug carrier.
(b) Higuchi model: Mt = kHt1/2.
Here kH is the Higuchi release constant. This model assumes that the drug released from an insoluble matrix and the rate is proportional to the square root of time, t1/2. The Huguchi model assumes a Fickian diffusion.
(c) Hixson–Crowell model: 1001/3 − Mt1/3 = kCt.
Here kC is the Hixson–Crowell release constant. In this case, the cubic root of the percentage of unreleased drug is proportional to t, and the drug carrier is eroded (dissolved) proportionally with time. We have fitted the experimental data for the release profiles of BRB and MB in Fig. 5–7 to these three kinetic models and Table 1 presents the results for their correlation coefficients. It can be seen from Table 1 that the data do not conform simply to the Higuchi model and that the erosion of the soap matrix also contributes to the overall BRB and MB release kinetics (see Fig. S2–S5 in ESI† for the fits). One would expect the Higuchi model to work best as the agar gel bead matrix does not dissolve or swell. However the beads start leaching drug only after the soap around them dissolves so it might be more complicated than Higuchi model in our composites. It is not a surprise that both Higuchi and Hixon–Crowell models are relevant due to the partitioning of some of the active from 2% agar gel beads to molten soap base during the sample preparation. Also, at higher concentration of agar (8%), the correlation coefficient is lower for Hixon–Crowell as the partitioning of BRB is slower from higher concentration of agar (and less syneresis) during the sample preparation. One may also suggest that the presence of PSS has a much bigger effect on the release rate compared to the hydrogel bead sizes. The role of PSS added in the hydrogel is even more interesting and the Hixon–Crowell model works better there which indicates that the rate of release is probably controlled not by the diffusion through the agar hydrogel matrix but by the rate of dissociation of the BRB from the PSS. One would expect that more sophisticated kinetic model is needed to incorporate the complexity of the drug release in this case. We will address this in future publications on this topic.
Table 1 Correlation coefficient of linear regression of fitting BRB and MB release profiles with different kinetic models. The soap–hydrogel composites tested all contained 50% by volume of hydrogel beads of different compositions
Composition of beads within the soap hydrogel composite |
1st order model, R2 |
Higuchi model, R2 |
Hixon–Crowell model, R2 |
2% agar large beads |
0.8524 |
0.9928 |
0.9712 |
2% agar small beads |
0.9712 |
0.9284 |
0.9716 |
|
8% agar large beads |
0.8678 |
0.9926 |
0.8706 |
8% agar small beads |
0.9521 |
0.9776 |
0.9533 |
|
2% agar large beads 0.1% PSS |
0.9955 |
0.8644 |
0.9953 |
2% agar small beads 0.1% PSS |
0.9994 |
0.9102 |
0.9995 |
|
2% agar large beads 0.25% PSS |
0.9873 |
0.9387 |
0.9880 |
2% agar small beads 0.25% PSS |
0.9370 |
0.9795 |
0.9385 |
Mechanical properties of soap–hydrogel composites
The effect of hydrogel bead size and composition of the soap–hydrogel composites on the mechanical properties was investigated by testing at least three samples of each type at room temperature. The samples were subjected to compression and the force at structural failure was used to calculate the compressional strength, whereas the linear elastic region of the stress/strain curve was used to determine the Young modulus. The results are shown in Fig. 8A and B. It was found that an increase in the volume percentage of hydrogel beads incorporated within the composites decreases their compressional strengths. There was no significant effect of changing the size of the hydrogel beads up to 50% volume percentage of beads. The Young modulus, however, shows unexpected values that are greater than the soap control sample when the composites contained 5% or 7.5% by volume of hydrogel beads (small or large). At higher volume percentages of beads, the Young modulus is less than the soap control sample and decreases with increasing the volume percentage of hydrogel beads.
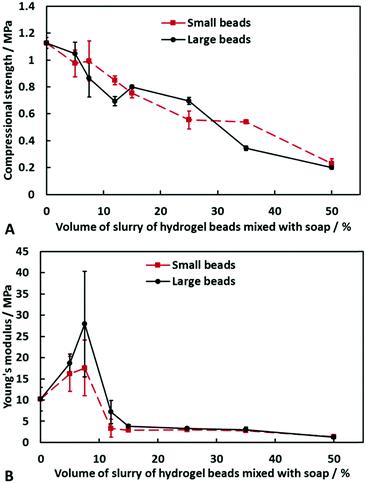 |
| Fig. 8 The compressional strength (A) and Young's modulus (B) of soap and soap–hydrogel beads composites as a function of the volume of hydrogel beads (either small or large) incorporated within the composites. | |
One possible reason for the initial increase in the Young modulus is that upon compression, the aqueous phase within the hydrogel beads has nowhere to go and so reinforces the composite. The large error bars could be due to the random distribution and arrangement of the beads within the composite. At higher volume percentage of beads, however, due to their partial formation of a network within the composite, water can be released from the beads upon compression and redistributed along the beads network which can dissipate energy and does not correspond to elastic deformation. An argument in favour of this explanation was revealed during the compression tests, where we observed syneresis of water from the composites produced with beads of either size. This effect is shown in Fig. 9 and furthermore, it gives scope for the production of these composites with a rather unusual rheological response compared to classic soap bars (see video S3 in ESI†).
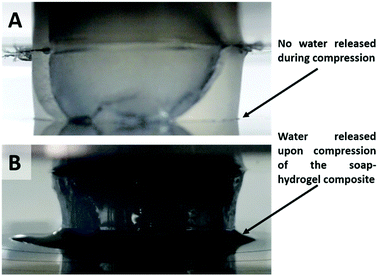 |
| Fig. 9 Digital camera images of a soap (A) and a soap–hydrogel composite produced with 50% by volume of small hydrogel beads (B) during compression. Note that the released water can be seen at the base of the composite. This effect is also seen when large hydrogel beads are used. | |
Conclusions
We have formulated novel pressure-responsive soap–hydrogel composites where hydrogel beads are encased within the soap matrix. These “smart” soap composites have a reduced cost, could reduce pollution with surface active materials and require less raw materials which improves the sustainability. The soap–hydrogel bead composites were stable for months in a standard soap packaging. We utilised agar, which forms a non-ionic hydrogel with a high melting point, for the preparation of these composites. Upon chopping the hydrogel to beads of a desired size with a blender, controlled volume percentages of hydrogel bead slurry were mixed with molten soap. This mixture was then poured into a mould and allowed to harden before use. We found that the soap dissolution rate increased with the volume percentage of hydrogel beads incorporated in the composite. The dissolution rate of composites with 50% by volume of hydrogel beads was 2.8 times faster than the soap control sample. We attribute this to an increase in the surface area of the soap bar exposed to water as hydrogel beads are detached from the surface of the composite.
The release rate of species encapsulated within the hydrogel beads used in the composites was also investigated. It was found that the release rate of berberine could be increased almost two-fold by changing the size of hydrogel beads used from 600 ± 300 μm to 120 ± 60 μm. The increase in surface area to volume ratio of smaller hydrogel beads, along with decreased diffusion path lengths and increased concentration gradients of berberine when using small hydrogel beads seems a plausible explanation for the observed difference in the release rates. We were able to control the release rate of the encapsulated active by changing the agar concentration in the hydrogel. As the concentration of agar increased, the active release rate decreased due to the formation of a denser polymer network, hindering the transport of the diffusing molecules. Another method to control the release rate of actives was to co-encapsulate an oppositely charged polyelectrolyte to attract the active species. The release rate of the encapsulated active decreased as the concentration of the polyelectrolyte increased which could be attributed to ionic attraction between the polyelectrolyte and the diffusing active molecules, as well as to the polyelectrolyte decreasing the free volume within the hydrogel beads, thus hindering the molecular transport. The mechanical properties of the composites were investigated and found that the compressional strength decreased linearly with an increase in the volume percentage of hydrogel beads in the composite irrespective of the hydrogel bead size. The composites also showed a compression driven syneresis of water, suggesting that they could exercise washing action without running water. The Young modulus showed a significant increase when 5% or 7.5% by volume of hydrogel beads were incorporated within the composite. However, at higher volume percentages of beads, it decreased to values below the soap control sample.
We envisage that such “smart” soap–hydrogel beads composites could find application for more sustainable solution within the hotel industry, where millions of partially used soap bars are discarded on a daily basis. The reduced cost of the smaller amount of soap base required for these materials combined with the possibility to encapsulate actives in the hydrogel beads content and the control of their release would make these composites appealing in personal care products.
Conflicts of interest
There are no conflicts to declare.
Acknowledgements
B. R. T. acknowledges the financial support from EPSRC/Unilever CASE studentship for his PhD study.
References
- C. Boschi-Pinto, L. Velebit and K. Shibuya, Bull. W. H. O., 2008, 86, 710–717 CrossRef PubMed.
- M. Burton, E. Cobb, P. Donachie, G. Judah, V. Curtis and W.-P. Schmidt, Int. J. Environ. Res. Public Health, 2011, 8, 97–104 CrossRef PubMed.
- T. Ivanković and J. Hrenović, Arch. Ind. Hyg. Toxicol., 2010, 61, 95–110 Search PubMed.
- C. L. Yuan, Z. Z. Xu, M. X. Fan, H. Y. Liu, Y. H. Xie and T. Zhu, J. Chem. Pharm. Res., 2014, 6, 2233–2237 Search PubMed.
- M. Rutkevičius, S. K. Munusami, Z. Watson, A. D. Field, M. Salt, S. D. Stoyanov, J. Petkov, G. H. Mehl and V. N. Paunov, Mater. Res. Bull., 2012, 47, 980–986 CrossRef.
- M. Constantin, S.-M. Bucatariu, F. Doroftei and G. Fundueanu, Carbohydr. Polym., 2017, 157, 493–502 CrossRef CAS PubMed.
- L. Zhang, K. Li, W. Xiao, L. Zheng, Y. Xiao, H. Fan and X. Zhang, Carbohydr. Polym., 2011, 84, 118–125 CrossRef CAS.
- M. Rutkevičius, G. H. Mehl, J. T. Petkov, S. D. Stoyanov and V. N. Paunov, J. Mater. Chem. B, 2015, 3, 82–89 RSC.
- B. R. Thompson, T. S. Horozov, S. D. Stoyanov and V. N. Paunov, Food Funct., 2017, 70, 448–455 Search PubMed.
- B. R. Thompson, T. S. Horozov, S. D. Stoyanov and V. N. Paunov, RSC Adv., 2017, 7, 45535–45544 RSC.
- B. R. Thompson, B. L. Taylor, Q. Qin, S. D. Stoyanov, T. S. Horozov and V. N. Paunov, Mater. Chem. Front., 2017, 1, 2627–2637 RSC.
- B. R. Thompson, T. S. Horozov, S. D. Stoyanov and V. N. Paunov, Mater. Des., 2018, 137, 384–393 CrossRef CAS.
- S. Arnott, A. Fulmer, W. E. Scott, I. C. M. Dea, R. Moorhouse and D. A. Rees, J. Mol. Biol., 1974, 90, 269–284 CrossRef CAS PubMed.
-
R. Armisén and F. Gaiatas, 4 – Agar, in Handbook of Hydrocolloids, ed. G. O. Phillips, P. A. Williams, Woodhead Publishing Limited, Cambridge, 2nd edn, 2009, ch. 4, pp. 82–107 Search PubMed.
- C. Berkland, K. Kim and D. W. Pack, Pharm. Res., 2003, 20, 1055–1062 CrossRef CAS.
- J. Siepmann and A. Göpferich, Adv. Drug Delivery Rev., 2001, 48, 229–247 CrossRef CAS PubMed.
- C. Berkland, E. Pollauf, C. Raman, R. Silverman, K. Kim and D. W. Pack, J. Pharm. Sci., 2007, 96, 1176–1191 CrossRef CAS PubMed.
- C. Berkland, K. Kim and D. W. Pack, J. Controlled Release, 2001, 73, 59–74 CrossRef CAS PubMed.
- C. Berkland, M. J. Kipper, B. Narasimhan, K. Kim and D. W. Pack, J. Controlled Release, 2004, 94, 129–141 CrossRef CAS PubMed.
- M. Dunne, O. I. Corrigan and Z. Ramtoola, Biomaterials, 2000, 21, 1659–1668 CrossRef CAS PubMed.
- D. Klose, F. Siepmann, K. Elkharraz, S. Krenzlin and J. Siepmann, Int. J. Pharm., 2006, 314, 198–206 CrossRef CAS PubMed.
- R. V. Kulkarni, V. Sreedhar, S. Mutalik, C. M. Setty and B. Sa, Int. J. Biol. Macromol., 2010, 47, 520–527 CrossRef CAS PubMed.
- M. Chu, M.-B. Zhang, Y.-C. Liu, J.-R. Kang, Z.-Y. Chu, K.-L. Yin, L.-Y. Ding, R. Ding, R.-X. Xiao, Y.-N. Yin, X.-Y. Liu and Y.-D. Wang, Sci. Rep., 2016, 6, 24748 CrossRef CAS PubMed.
- M. J. Al-Awady, A. Fauchet, G. M. Greenway and V. N. Paunov, J. Mater. Chem. B, 2017, 5, 7885–7897 RSC.
- R. Gouda, H. Baishya and Z. Qing, J. Dev. Drugs, 2017, 6, 1000171 Search PubMed.
- J. Siepmanna and N. A. Peppas, Int. J. Pharm., 2011, 418, 6–12 CrossRef PubMed.
- K. H. Ramteke, P. A. Dighe, A. R. Kharat and S. V. Patil, Scholars Acad. J. Pharm., 2014, 3, 388–396 Search PubMed.
Footnotes |
† Electronic supplementary information (ESI) available. See DOI: 10.1039/c7qm00556c |
‡ Present affiliation: Department of Chemical and Biomolecular Engineering, North Carolina State University, Raleigh, NC, USA. |
|
This journal is © the Partner Organisations 2018 |