DOI:
10.1039/C7QM00416H
(Review Article)
Mater. Chem. Front., 2018,
2, 219-234
Hybridization of metal–organic frameworks and task-specific ionic liquids: fundamentals and challenges
Received
11th September 2017
, Accepted 19th October 2017
First published on 20th October 2017
Abstract
Metal–organic frameworks (MOFs) and task-specific ionic liquids (TSILs) have been active as a high-profile class of versatile materials. However, there was no relatively close intersection between MOFs and TSILs until the concept of hybridization of MOFs and TSILs was proposed in the most recent years. At present, it has been well witnessed that the development of MOF and TSIL hybrid materials (MTHMs) is attracting significant attention. In this regard, it is high time to address the recent advances made in the fast-growing field of MTHMs and provide a critical assessment of this subject matter. In this review, as an originator of MTHMs, we have presented a logical and easy-to-follow story about the hybridization of MOFs and TSILs: from their origins, to the motivation behind hybridization, to a strategy to carry out the hybridization, and finally to the consequences of hybridization based on a few illustrative examples. Finally, the specific bottlenecks, challenges, and outlooks towards this subject matter have been proposed according to the state-of-the-art progress as well as our first-hand experiences and personal perspectives. Hopefully, the knowledge and learning points gained from this review will deepen the understanding of MTHMs and appeal to more multidisciplinary communities coming forward with their valuable contributions in the coming decades.
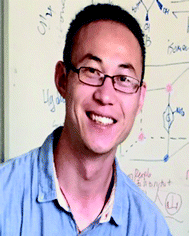
Qun-xing Luo
| Dr Qun-xing Luo obtained his PhD degree in Physical Chemistry from Dalian University of Technology (DUT), China, in 2016. To fulfil his PhD research requirements, he spent a year as Joint-Supervision student at the University of California, Santa Barbara (UCSB), USA. After receiving his PhD, he joined Shannxi Normal University (SNNU) as a Tenure-Track Assistant Professor. At present, his research interests focus on the synthesis and reengineering of porous materials as well as their applications in catalysis, separation and process intensification related to clean energy. |
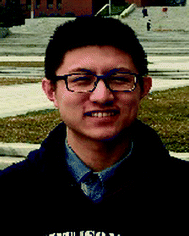
Bo-wen An
| Bowen An is a senior undergraduate in Dalian University of Technology (DUT), China, who is set to receive his Bachelor's degree in Applied Chemistry in July 2018. He is conducting an innovative experimental programme for undergraduates under the supervision of Prof. Min Ji. He has done some work on the synthesis and characterization of MOFs. At present, he is preparing to pursue a doctoral study with interest in the development of porous and nanostructured materials for applications in catalysis and energy storage. |
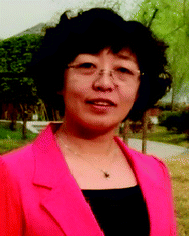
Min Ji
| Prof. Min Ji obtained her PhD in Physical Chemistry from Jilin University, China, in 1996 and worked there until 1998. Afterwards, she joined Dalian University of Technology (DUT) as an associate professor. In 2012–2013, she worked as a postdoctoral researcher with Prof. Sang-Eon Park at the Korean Research Institute of Chemical Technology, and was awarded a Brainpool Fellowship. She is currently a full professor at the School of Chemistry, DUT. Her main research activities are focused on the catalytic conversion of CO2, the design and synthesis of immobilized Lewis acids and IL catalysts and their applications in the synthesis of fine chemicals. |
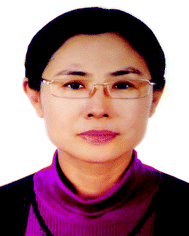
Jie Zhang
| Prof. Jie Zhang received her PhD degree in Jilin University in 1996 and then worked there. After her postdoctoral research at ChangChun Institute of Applied Chemistry, CAS, and at Tokyo Metropolitan University as a JSPS fellow, she joined Fujian Institute of Research on the Structure of Matter, CAS. In 2014, she moved to Beijing Institute of Technology as a Professor of Chemistry. Her research interests focus on developing molecular recognition and photoresponsive materials based on small conjugated molecules. |
1. Introduction
The term hybridization in nature is commonplace, where the original intention is to create a new offspring resulting from two discriminatively biological species through artificial introgression.1–3 It is generally accepted that the resulting hybrid species can display a few unprecedented consequences due to the combination of advantages of at least two or more individuals. As a result, these hybrid processes might render an amplification effect (1 + 1 > 2, as shown in Fig. 1) for a new hybrid species because of the synergistic effects and/or mutual promotion rather than a simple mathematic adduct. During the past few decades, this sort of hybrid concept has been gradually introduced into material science.4–6 One of the most important reasons for this trend is that this concept can afford access to design and re-engineering of a kind of advanced composite materials with versatile functionalities and extraordinary properties.
 |
| Fig. 1 Schematic of the amplification effect for hybrid processes. | |
In general, these hybrid materials, with a well-defined composition and structure, are composed of host and guest moieties, which are blended at the molecular level. Moreover, these host and guest materials can be either single inorganic or organic components as well as binary inorganic–organic components.7 It is well established that some typically inorganic materials (e.g. simple substances, metal/non-metal oxides, zeolites, and ceramics) can be rationally designed and controllably synthesized. Similarly, majority of organic materials can also be well designed and predicated at the atomic or molecular level. More importantly, these pristine materials with unique structures and properties have displayed potential in a wide range of task-specific application fields such as mechanical engineering,8,9 and thermal,10 electrical,11 optical,12 and chemical behaviours.13,14 However, “there are lees to every wine”. Therefore, one particular interest is to combine two different materials and create a sort of new organic–inorganic hybrid material to reinforce the individual advantages and remedy the disadvantages.
During the past few decades, organic–inorganic hybrid materials (OIHMs) have attracted significant attention15 and have been involved in many widespread applications such as in semiconductors,16 electrochemistry,17 medicine,18 optics,19 adsorption and separation membranes,20 as well as heterogeneous catalysis.21 In addition to the early interests in hybrid materials by blending two sorts of independent materials, significant recent effort has been focused on the design and development of single compounds containing both inorganic and organic components. Typically, metal–organic frameworks (MOFs), a kind of organic–inorganic hybrid materials, are constructed by assembling metal ions/clusters with organic ligands using coordination bonds to create open frameworks with permanent porosity. Compared with those of the traditionally porous materials, the chemical compositions and framework structures of MOFs can be multiply tailored based on the well-defined molecular building blocks via reticular synthesis and/or crystal engineering.22–25 In addition, ionic liquids (ILs), another kind of organic–inorganic hybrid materials, are usually composed of organic cations and inorganic anions.26 In particular, a variety of functionalized groups can also be incorporated into ILs by altering the combination of their cations and anions, and this forms the so-called task-specific ionic liquids (TSILs).27,28
In this context, it is well-recognized that both TSILs and MOFs are very versatile materials and show promising potential for extensive applications. However, there was still no relatively close intersection between MOFs and TSILs until the immobilization of TSILs onto MOFs through coordination bonds was proposed in the most recent years.29 After this, this concept towards the hybridization of MOFs and TSILs gradually attracted extensive attention from multidisciplinary communities, especially with a focus on a variety of applications such as in gas adsorption and separation,30–45 hybrid membranes,46–48 chromatography,49 liquid-phase selective adsorption of sulfide,50–52 heterogeneous catalysis,29,41,53–67 ionic conduction,68–73 and templates for the synthesis of functional materials.74 Moreover, these abovementioned aspects about MOF and TSIL hybrid materials (MTHMs) have been summarized in the literature.75–79 Herein, as an originator of MTHMs, we aimed to contribute a critical review on the fast-growing field of MTHMs on the basis of the state-of-the-art progress as well as our first-hand experiences and personal perspectives. In this review, we have strived to tell a logical and easy-to-follow story about MTHMs through discussing a few illustrative examples, which may be useful for anyone who is interested in MTHMs.
2. Why do we propose hybrid materials of MOFs and TSILs?
2.1 Objective appraisal of MOFs
In the field of porous solids, from zeolites, mesoporous silica, to MOFs and porous organic frameworks (POFs), MOFs are recognized as “apple of the eye” in the most recent years.80 This high evaluation can actually be attributed to the following facts: (I) they are crystalline compounds consisting of infinite constitutional units built up of inorganic nodes and organic linkers (Fig. 2); (II) the distinctive characteristics of ultrahigh surface area and porosity and uniform size of nanopores situated within the range of that of many molecules of interest make them the desirable catalysts and supports; (III) the well-defined metal sites within the frameworks have a high content and are homogeneously distributed; this allows them to serve as a kind of mild catalytically active centers; (IV) the organic ligands can be readily pre- and post-functionalized to incorporate a sort of task-specific group; and (V) the nature of the chemical composition in MOFs endows them with structural transformability and flexibility. As a result, MOFs as a porous matrix or scaffold, by virtue of their intrinsic features, can usually be employed to introduce a variety of guest functional molecules by an in situ synthesis or post-synthetic modification (PSM) method81–87 to develop new functional materials. However, a majority of MOFs themselves still have some fatal weaknesses such as poor thermal and chemical stability and a limited number of active or functional sites; this results in their stringent constitution for chemical modification and special requirements in specific applications. In this regard, it is a challenging task to design and synthesize robust MOFs and understand the host–guest interactions and mutual effects between the MOFs and guest species.
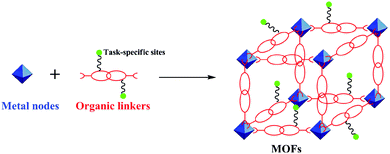 |
| Fig. 2 Schematic of the construction of metal–organic framework materials. | |
2.2 Objective appraisal of TSILs
Ionic liquids, a kind of organic molten salt and free-flowing liquid at room temperature, are composed entirely of cations and anions. In general, the common cations are organic imidazolium, pyridinium, pyrrolidinium, ammonium, and phosphonium, and the anions are inorganic acid radicals or complex ions, as illustrated in Fig. 3. The nature of ion pairs (cations and anions) with an asymmetric structure gives rise to a weak intermolecular interaction, contributing to a set of fascinating and unique physicochemical properties such as a negligible vapour pressure, non-flammability, high thermal and chemical stability, favourable conductivity, wide electrochemical window, good capability of dissolving various compounds, low toxicity, and acceptable biocompatibility.26,88–92 On the other hand, the synthesis of ILs is highly flexible, endowing an ability to tailor the component of ions and tune the physicochemical properties and functionalities. Therefore, the chemical and structural diversities of TSILs make them a sort of potential modifier to decorate various host materials and improve their properties. In view of these characteristics, significant interest has been shown in the application of TSILs in the green synthesis of organic compounds,91,93,94 catalysis,95–99 adsorption and separation,100 extraction,101,102 lubrication,103 electrochemistry,104–108 ionothermal syntheses of materials,109–112etc.
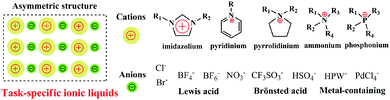 |
| Fig. 3 Main cations and anions of task-specific ionic liquids. | |
Actually, use of TSILs also presents several practical issues in real operation and application due to the intrinsic viscosity and low diffusion coefficient of TSILs, as well as high cost of their synthesis. In this regard, it is generally accepted that heterogenization of TSILs is a valid strategy to circumvent the challenging operation in viscous media and reduce the dosage of TSILs. To date, this concept has been implemented on the surface of robust supports (e.g. silica, polymer, and carbon material) by impregnation or covalent attachment.113–118 Moreover, it has been observed that the combination of TSILs and solid supports generates several positive responses, such as synergistic effects, favourable solubility of composites, surface wettability, straightforward separation, and recycling, in real applications. In this regard, use of other types of solid materials as supports to explore the heterogenization of TSILs, especially focusing on the microenvironment effect of the support, is highly necessary and desired. It is thus expected that these newborn composite materials would not only remedy the shortcomings of TSILs in application processes, but also open a new window for TSIL chemistry.
2.3 Superiority of MTHMs
As the saying goes, “enhance strengths and circumvent weaknesses” or, more widely speaking “win–win”. In the cases of MOFs and TSILs materials, they are no exception. Undoubtedly, these unique aspects of MOFs endow them with the potential to serve as host materials or supports. Similarly, TSILs are entitled to be a sort of versatile guest molecules since they possess a set of unusual physicochemical properties. In view of the respective merits and demerits of MOFs and TSILs, their hybridization materials may not only intensify the material strengths and avoid the weaknesses, but also evoke several new superiorities as follows:
(1) The functionality and diversity of MOFs can be further enriched by the incorporation of TSILs, and this would potentially contribute to a wider range of applications.
(2) Several practical issues towards the recovery, separation, purification, and recycling of TSILs in handling procedures may be addressed. Moreover, it is expected to reduce the dosage and save the cost of TSILs.
(3) The presence of, to some extent, analogous chemical properties (π-electron and aromaticity) of organic moieties and the flexible framework within MOFs and TSILs, as well as the electrostatic field, is conducive to constructing the favourable interactions between MOFs and TSILs.
(4) The hybridization of MOFs and TSILs and the alliance between them can potentially trigger an unprecedented performance due to the integration of the respective advantages.
3. Fundamental insight into MTHMs based on theoretical simulations
The initial report on MOF and TSIL composites, to the best of our knowledge, was proposed by Jiang's group (National University of Singapore) based on theoretical calculations and simulations.40,44,45 After this, especially in recent years, a few computational studies on the composite systems of MOFs and TSILs have been reported in succession.31,36–39,43,119 Specifically, these reports were committed to studying the microscopic properties, structure, and dynamics of ionic liquids inside the MOF nanopores, the location and dispersion of ionic liquids, as well as the host–guest interactions. Furthermore, the effects of the microscopic behaviours on the performance of gas adsorption and separation were investigated. Hereinafter, these microscopic behaviours have been discussed one by one.
3.1 Location of TSILs
As is known, MOFs are highly porous materials and possess large and empty nanospaces in their microstructures. Ionic liquids are a sort of electrolyte, consisting of anions and cations. Therefore, it is interesting to probe the location of ionic liquids in the MOF nanopores. Jiang and co-workers conducted a study on the IRMOF-1-supported 1-n-butyl-3-methylimidazolium hexafluorophosphate ([BMIM][PF6]) ionic liquid by molecular computation.45 On the basis of the calculation of radial distribution functions g(r), it was found that these IL molecules were packed in a more ordered state inside the nanopores due to the confinement effect. In addition, it was revealed that the bulky [BMIM] cations tended to reside in the open pores of IRMOF-1, whereas the small [PF6] anions preferred to locate in the corner of metal clusters of IRMOF-1, as shown in Fig. 4.
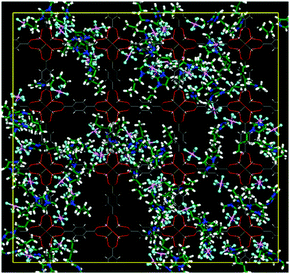 |
| Fig. 4 [BMIM][PF6]/IRMOF-1 composite at a weight ratio WIL/IRMOF-1 of 0.4. N, blue; C in [BMIM]+, green; P, pink; F, cyan; Zn, orange; O, red; C in IRMOF-1, gray; and H, white (reproduced with permission from ref. 45. Copyright 2011, American Chemical Society). | |
On the basis of the abovementioned results, a set of ionic liquids with the identical cation ([BMIM]+), but four different anions, i.e. tetrafluoroborate ([BF4]−), hexafluorophosphate ([PF6]−), thiocyanate ([SCN]−), and bis(trifluoromethylsulfonyl) imide ([Tf2N]−), was employed to investigate the effects of the anions.40 It was found that the small anions [PF6]−, [BF4]−, and [SCN]− preferred to locate near the metal clusters, particularly the quasi-spherical [PF6]− and [BF4]−. In contrast, the bulky and chain-like [BMIM]+ and [Tf2N]− resided near the phenyl rings of MOFs. Furthermore, the ionic liquid ([BMIM][SCN]) supported on hydrophobic ZIF-71 and hydrophilic Na-rho-ZMOF with the same topology and a similar pore size were investigated by atomistic simulation.44 It turned out that [SCN]− anion preferred to locate near the metal clusters of ZIF-71 and Na+ ion of Na-rho-ZMOF, whereas the bulky and chain-like [BMIM]+ cation resided in the open cages of MOFs, as shown in Fig. 5.
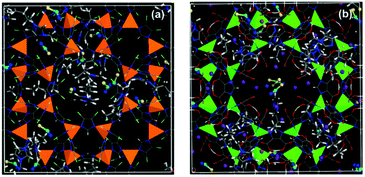 |
| Fig. 5 (a) [BMIM][SCN]/ZIF-71 and (b) [BMIM][SCN]/ZMOF membranes at a weight percentage WIL/MOF of 0.15. C of [BMIM]+ and framework: grey; C of [SCN]−: cyan; S: yellow; N: blue; Zn: orange; Cl: light green; In: green; Na+: purple; O: red; and H: white. The metal clusters are shown as polyhedra (reproduced with permission from ref. 44. Copyright 2013, American Chemical Society). | |
Similarly, Zhong and co-workers conducted a study on four types of ILs combined from the identical cation [BMIM]+, but different anions ([Cl]−, [Tf2N]−, [PF6]−, and [BF4]−) supported on MOFs (Cu-TDPAT).39 It was found that the anions were preferentially located around the metal sites of MOFs. Moreover, the distance between the anions and metal sites was in line with the sizes of the anions ([Cl]− < [BF4]− < [PF6]− < [Tf2N]−). Specifically, the quasi-spherical structures of the former three anions were closer to the metal centres than that of [Tf2N]−, with a chain-like structure. In addition, several observations based on simulated structures of the ILs/Cu-TDPAT composites indicated that ILs with a larger size (e.g. [BMIM][Tf2N], [BMIM][PF6], and [BMIM][BF4]) appeared in three types of Cu-TDPAT cages or in the intersection regions between these cages, whereas the ionic liquid ([BMIM][Cl]) with a smaller size dominantly occupied the tetrahedral cages with the smallest pore size.
3.2 Interaction between MOFs and TSILs
Unlike the traditional liquids (e.g. water or organic solvent), the cations and anions of ionic liquids serve independent roles in determining their interaction behaviours with MOF materials since both of them bear a variety of interaction sites or functional groups (Fig. 6a). On the other hand, several accessible interaction sites or groups can also be found in MOF materials (Fig. 6b). As a result, there are more complicated interaction systems such as Coulombic forces (electrostatic attraction or repulsion), polarization, van der Waals interactions, π–π interactions, and hydrogen-bonding.42,88,120 Therefore, elucidation and understanding of the specific interactions between MOFs and TSILs would assist in optimizing the performance of their hybrid materials; however, it is still a big challenge.
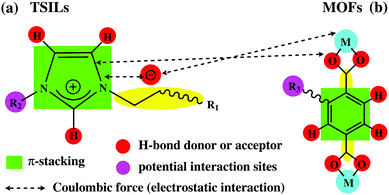 |
| Fig. 6 Schematic of the potential types of interaction between MOFs and TSILs. | |
At present, it is generally accepted that there is a stronger interaction between anions and metal clusters of MOFs due to Coulombic forces.40,44,45 Moreover, it has been found that the interaction between MOFs and the anions of TSILs is dependent on the size of anions. In general, a smaller size results in a stronger interaction such as for [Cl]−, [BF4]−, and [PF6]−. In contrast, [Tf2N]− with a larger size has a weaker interaction with MOFs, and in turn, it interacts strongly with the cation ([BMIM]+).39 Very recently, Kim and co-workers conducted both DFT calculations and an experimental study on a composite system of Cu-BTC and an ionic liquid, 1-ethyl-3-methylimidazolium ethyl sulfate ([EMIM][ETS]).119 Interestingly, it was found that the interaction of the ionic liquid with Cu ions of MOFs gave rise to a perturbation of the symmetry of the MOF structure. In addition, it was observed that two different types of ion pairs were formed inside the pores of MOFs. Among these, the first ion pair represents an enhanced interionic interaction via strengthening of hydrogen-bonding and change in the polarizability of the C2–H bonds between cations and anions, whereas the second ion-pair configuration shows a weakened interaction between the ions of the ionic liquid. Moreover, it has been shown that the imidazole ring of an ionic liquid can directly interact with either the MOFs or the counterpart anions. As a result, it is speculated that the transfer and/or redistribution of electron density are responsible for the molecular interactions of MOFs and ILs.
3.3 Dispersion and dynamics behaviour of TSILs
In principle, the dispersion and dynamics behaviour of TSILs in MOFs are strongly dependent on their location and interaction with MOFs. It has been well established that the anions of an ionic liquid prefer to locate around metal clusters, whereas the cations reside in the vicinity of the organic ligands of MOFs. Moreover, an ionic liquid with a bulky size is more inclined to be distributed in bigger cages or in the pore-intersection regions of MOFs. As a result, it is reasonable to understand that the preferential distribution of TSILs in MOFs and the strong interaction between MOFs and TSILs can facilitate the dispersion and suppress the aggregation of TSILs, but at the expense of mobility. Very recently, Yang et al. conducted a systematic computational study by molecular dynamics (MD) simulation to investigate the dispersion behaviour of an ionic liquid in MOFs and covalent organic frameworks (COFs).37 Specifically, a series of MOFs and COFs with diverse pore structures and chemical properties were employed as supports. The ionic liquid used was 1-n-butyl-3-methylimidazolium thiocyanate [BMIM][SCN]. They employed coordination number to characterize the aggregation degree of the ionic liquid in the pores of the MOFs and COFs, as shown in Fig. 7. The results revealed that the molecules of the ionic liquid were more inclined to aggregate in the two-dimensional (2D) COFs and one-dimensional (1D) MOFs. On the contrary, they were more favourable to dispersion in either MOF or COF materials with a three-dimensional (3D) pore structure. Moreover, it was shown that compared to the case of COFs (without a metal cluster and pure organic framework), the stronger Coulombic interactions can contribute to a better dispersion behaviour of the ionic liquid in the pores of the MOFs.
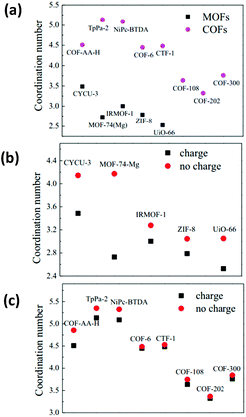 |
| Fig. 7 (a) The coordination number of [BMIM[]SCN] in the MOFs and COFs; (b) the coordination number of the ILs in the IL/MOFs; and (c) IL/COFs composites obtained with (denoted as charge) and without (denoted as no charge) taking into account the framework Coulombic contribution of the support materials for ILs dispersion (reproduced with permission from ref. 37. Copyright 2015, Elsevier Ltd). | |
4. General strategy for the hybridization of MOFs and TSILs based on experimental studies
Actually, the hybridization of MOFs and TSILs can usually be defined as the domain of heterogenization of the TSILs. In this context, heterogenization, according to type of the interaction (intermolecular forces and chemical bonds) between MOFs and ionic liquids, is specifically classified by the supported ionic liquids, immobilization of the ionic liquids, and encapsulation of the ionic liquids. In this section, three typical strategies towards the hybridization of MOFs and TSILs, or the so-called heterogenization of TSILs, have been explicitly introduced and discussed.
4.1 MOF-supported ionic liquids (intermolecular force)
The concept of supported ionic liquids (SILs) was first proposed and applied in catalysis by Mehnert's group in 2002.115,121 However, they initially had no intention to make a distinction between supported and immobilized ionic liquids. However, herein, to make a clear-cut distinction, we redefined the concept of supported ionic liquids, referring in particular to the systems without chemical bonds and with intermolecular forces (e.g. electrostatic interactions, van der Waals interactions, π–π interactions, and hydrogen-bonding) that stabilize the ionic liquids. Considering this reference, the visibility of SILs on traditional solid materials (e.g. silica, carbon, and polymers) has been confirmed in many cases.121–125 However, only a few studies have been reported on MOF-supported ionic liquid systems with intermolecular interactions although there are a number of potential types of interactions between MOFs and TSILs, as abovementioned in Fig. 6. Typically, mesoporous metal–organic frameworks, MIL-101 (Cr), are usually used to support ionic liquids, including 1-butyl-3-methylimidazolium chloride,50 1-butyl-3-methylimidazolium acetate ([Bmim][OAc]),51 and N-methyl-2-pyrrolidonium methyl sulfonate,53 by an impregnation method. In addition, a metal–organic framework UiO-66-supported ionic liquid (1-methylimidazolium-3-propylsulfonate hydrosulfate, PSMIMHSO4) has been reported.59 However, these studies fail to elucidate the specific interactions between MOFs and TSILs although these MTHMs systems demonstrate stability and reusability to a certain extent. As a consequence, these half-done works need to be further consummated in follow-up work.
In addition to this conventional post-impregnation method, MOF-supported ionic liquids can be obtained by ionothermal synthesis, where the precursors of MOFs are dissolved in ionic liquids or along with other solvents. Upon crystallization, the frameworks of the as-synthesized MOF materials are charged. As a result, a certain amount of ionic liquids as charge-compensating elements remain in the structure of the MOFs to maintain electrical neutrality. In this regard, the first successful example involves the synthesis of Cu(bpp)BF4 [bpp = 1,3-bis(4-pyridyl)propane] using BMImBF4 as a solvent.126 Subsequently, other kinds of ionic liquids and MOFs have been demonstrated.127–129 Herein, we recommend a couple of review articles on ionothermal synthesis of MOFs to expand specialized interests.111,130
4.2 Immobilization of ionic liquids on MOFs (chemical bonds)
The immobilization of ionic liquids via the formation of chemical bonds can be implemented by different ways. According to the ions involved in the formation of chemical bonds, this method can be classified as cation or anion immobilization. Given the nature of MOFs, i.e. since they consist of inorganic metal ions and organic ligands, covalent and coordination bonds can be formed between MOFs and TSILs, respectively. In addition, ionic bonds are probably formed because the anions with negative charge in TSILs can interact with the metal centres in the MOFs with a positive charge. It was, therefore, concluded that all the immobilization cases can be systematically categorized, as illustrated in Fig. 8. Notably, TSILs immobilized on MOFs showed a high dispersion and uniform distribution by chemical bonds because the anchoring sites within the MOFs are well defined and periodically distributed; this allows them to bear the homogeneous task-specific sites and favourable microenvironment for mass-transfer and diffusion.
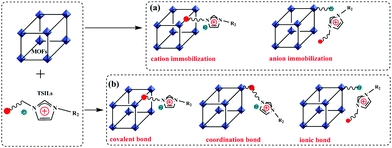 |
| Fig. 8 Classification for the immobilization of TSILs on MOFs, (a) based on cations and anions and (b) based on the type of chemical bond. | |
Pioneering studies on the immobilization of TSILs on MOFs have been reported by our group.29,54–56 Previously, we have proposed that TSILs can be immobilized inside the nanocavities of MOFs via coordination bonds. As the first example, a tandem post-synthetic modification strategy was used to construct a Brönsted acidic ionic liquid confined inside well-defined MIL-101 nanocages (Fig. 9), where the electron-rich N-heterocyclic compounds as the chemical bridge played a key role in the in situ synthesis of ionic liquids and formation of stable N–Cr coordination bonds.29 Subsequently, a facile method for the synthesis of HKUST-1 (or the so-called CuBTC)-immobilized amino-functionalized basic ionic liquid by coordination bonds with Cu–NH2 was demonstrated, as illustrated in Fig. 10.54,55 In addition, the 1-carboxyethyl-3-methyl imidazole Pd-containing chloride ionic liquid was immobilized on CuBTC through Cu–O coordination bonds.56 It is also worth mentioning that both the metal–organic framework MIL-101 and CuBTC materials used in these studies have metal coordinatively unsaturated sites (CUSs) that can serve as anchoring centres to form chemical bonds.131,132
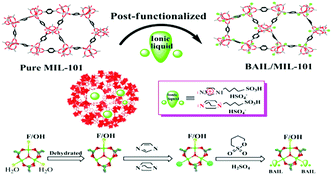 |
| Fig. 9 The generic tandem procedure for synthesizing BAIL confined in MIL-101 nanocages by post-synthetic modification (reproduced with permission from ref. 29. Copyright 2013, The Royal Society of Chemistry). | |
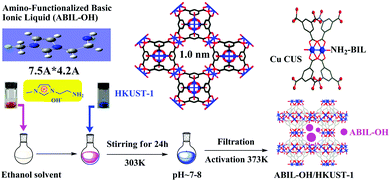 |
| Fig. 10 Schematic for the synthesis of the ABIL-OH/HKUST-1 catalyst (reproduced with permission from ref. 55. Copyright 2014, Elsevier B.V.). | |
Afterwards, a few analogical studies about the immobilization of TSILs on MOFs have been successively reported. For example, the ethanedithiol compound was selected as a chemical bridge to be grafted first on CUS of CuBTC material, and then, another thiol group was used to react with a vinyl-containing ionic liquid to prepare a TSILs/CuBTC hybrid material.64 Abbasi and co-workers reported the immobilization of a dual amino-functionalized ionic liquid on MIL-100 (Fe) material, where the precursor obtained was further used to support heteropolyacid H3PW4O24.66 Similarly, the immobilization of phosphotungstic acid into the nanocages of a dual amino-functionalized ionic liquid (DAIL)-modified MIL-101 (Cr) was also demonstrated by Voort's group.61 Therein, one amino group played a role in the formation of Cr–NH2 coordination bonds, and another amino group was used to interact with phosphotungstic acid, as illustrated in Fig. 11. In addition, it could be noted that the Pd nanoparticles stabilized via the TSILs (1,1,3,3-tetramethylguanidinium trifluoroacetate, TMGT) microphase could be immobilized on the CuBTC material.62 The results indicate that the nitrogen atom of TSILs gives rise to the mutual interaction between TSIL and Pd nanoparticles as well as between TSILs and MOFs (N–Cu coordination bonds).
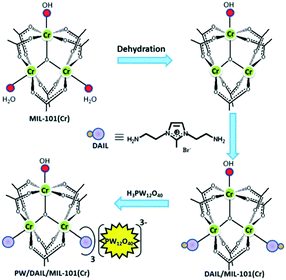 |
| Fig. 11 Schematic for the preparation of PW/DAIL/MIL-101 (Cr) (reproduced with permission from ref. 61. Copyright 2017, The Royal Society of Chemistry). | |
Besides the abovementioned cases, the manners of the covalent bond and electrovalent bond are also under investigation to immobilize ionic liquids. As a representative example, amino-functionalized MIL-101 (MIL-101-NH2) was used as a support to immobilize TSILs (N(n-Bu)3 or P(n-Bu)3) via the formation of covalent bonds between the ionic liquids and –NH2 groups of the organic ligands.65 Interestingly, different from the post-synthetic approach, a direct synthesis of UiO-67-immobilized TSIL hybrid material has been reported,41 as shown in Fig. 12. Specifically, an organic ligand modified using an imidazolium-based ionic liquid was first synthesized, and then, it was further used to prepare an UiO-67-TSIL hybrid material via solvothermal crystallization. In addition, a pyridinium-based ionic liquid immobilized on a zeolitic imidazolate framework (ZIF-90) material by covalent post-functionalization has been demonstrated by Park's group.58 In the case of immobilization through ionic bonds, only one demonstration on a Brönsted acid ionic liquid immobilized on amino-functionalized magnetic metal–organic framework composites (Fe3O4@NH2-MIL-88B) could be found.67 Therein, the abundant amino groups of the support could interact with the sulfoacid groups of TSILs by interionic interactions.
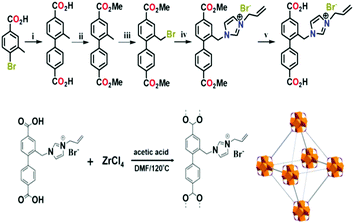 |
| Fig. 12 Schematic for the synthesis of an UiO-67-TSIL hybrid material (reproduced with permission from ref. 41. Copyright 2017, American Chemical Society). | |
4.3 Encapsulation of ionic liquids in MOFs (geometrical confinement)
The concept of encapsulation is by no means new and has been widely applied in a variety of research fields.133–135 As is known, one of the greatest strengths of MOFs is their fascinating textural properties such as ultrahigh porosity, extra-large surface areas, and open and accessible nanospace. These characteristics make them a sort of promising host materials to encapsulate guest species. Actually, there have been many reports on the encapsulation of functional molecules in MOFs.80,136–139 However, the encapsulation of TSILs in MOFs is still in its infancy at present. In addition, in a few cases, it is difficult to define the encapsulation and impregnation of TSILs. To be more explicit, herein, only real encapsulation (whereby all the moieties of the TSILs must be located within the pores or cavities of MOFs, and without leaching) has been discussed.
In general, the geometrical size and configuration of MOF materials and TSIL molecules are important parameters to determine the encapsulation efficiency and stability. As a result, the traditional impregnation method fails to realize real encapsulation because of leaching and an outer residual of TSILs. In this respect, the ship-in-bottle strategy is usually deemed as an ideal candidate because the reactants with a smaller size can be allowed to diffuse into the nanopores of the MOFs, whereas the target product synthesized usually has a larger size as compared to the pore of the MOFs. The resulting encapsulated guest species can be trapped in the nanopores by geometric and steric confinement effects. As a representative example, Jhung and co-workers reported that ionic liquids could be synthesized in the nanocages of MIL-101 via a ship-in-bottle technique,52 as shown in Fig. 13a. Specifically, N-methylimidazole and 1-bromobutane as initial reactants were introduced into the nanocages of MIL-101 in sequence and reacted under given reaction conditions. Similarly, a sort of poly(ionic liquid) was synthesized via assembly in the nanocages of MIL-101 and robustly confined within the pore lattice (Fig. 13b).47 In addition, the impregnation–reaction–encapsulation process was used to encapsulate a dicationic acid ionic liquid in the nanocages of MIL-100 (Fe).57
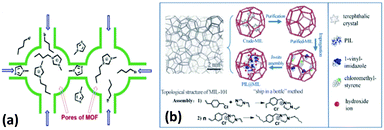 |
| Fig. 13
In situ synthesis of ionic liquids in the nanocages of MIL-101, (a) Jhung's work from ref. 52, (b) Jiang's work from ref. 47 (reproduced with permission from ref. 47 and 52. Copyright 2016, The Royal Society of Chemistry). | |
Apart from the abovementioned ship-in-bottle technique, so-called post-encapsulation, another typical method involves in situ encapsulation, namely the assembly of MOFs around TSILs. This approach refers to the utilization of TSILs as a soft template to synthesize MOFs by self-assembly. During the period of synthesis, a certain amount of TSILs are encapsulated in the nanospace of the as-synthesized MOF materials simultaneously. This process is illustrated in Fig. 14. Unfortunately, the technique is usually fastidious or may be altogether thwarted because the majority of organic ligands are labile under extreme conditions of ions; thus, only a limited number of MOFs can be synthesized. Actually, to some extent, a few cases of ionothermal synthesis have been roughly regarded as in situ encapsulation, but the outer and surface-adsorbed TSILs must be thoroughly removed. Overall, this protocol towards the in situ encapsulation of TSILs in MOFs is still at the conceptual stage and needs a realistic breakthrough in experimental strategy.
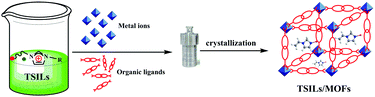 |
| Fig. 14 Schematic of the in situ encapsulation of TSILs via the ionothermal synthesis of metal–organic frameworks. | |
5. Overview towards the application of MTHMs
As abovementioned, it is well known that MOFs and TSILs have many applications. To the best of our knowledge, however, only a limited number of applications can be migrated to MTHMs and have been reported to date. In the following section, we have provided a closer observation of a few specific applications of MTHMs in gas adsorption and separation, heterogeneous catalysis, adsorptive desulfurization, and electrochemistry. In particular, several interesting phenomena, such as the confinement effect, molecular size- and shape-selective catalysis, and synergistic catalysis, observed in heterogeneous catalysis have been highlighted. In addition, a couple of emerging but infant applications (e.g. in extraction and chromatography and as precursors or templates for the synthesis of novel functional materials) have also been enumerated.
5.1 Gas adsorption and separation
In view of the enhanced properties of MTHMs resulting from the combination of the respective merits of MOFs and TSILs, one of the most extensive applications is in gas adsorption and separation. Therein, the most popular system, CO2 adsorption and separation from the binary mixed gases (e.g. CO2/N2, CO2/CH4, and CO2/H2) and CH4 adsorption and separation from the binary mixed gases (e.g. CH4/N2 and CH4/H2), has been across-the-board discussed in the review publications.30,75,76,78,140 In addition, the selective separation of C2H2 from the mixture of C2H2/C2H4 and the selective removal of H2S from a H2S/CH4 mixture have also been reported.39,51 To avoid repetitive discussion, herein, we do not intend to review the former, whereas the latter applications as representative have been thoroughly introduced.
Zhong and co-workers conducted a computational study and found that introduction of ionic liquids, in particular ILs with smallest size ([Cl]−), into metal–organic framework Cu-TDPAT could significantly enhance the adsorption affinity of H2S as compared to the case of pristine MOFs. Moreover, they found that increase in the loading of ionic liquid could generally increase the adsorption selectivity of H2S/CH4 within the pressure range examined herein.39 In the case of the adsorptive separation of acetylene and ethylene, the adsorbent reported was a hybrid material of 1-butyl-3-methylimidazolium acetate ([Bmim][OAc]) and MIL-101. Compared to that of the parent MIL-101, the adsorption selectivity of C2H2/C2H4 over this composite adsorbent could be dramatically increased from 3.0 to 30.51 Moreover, the breakthrough curve (Fig. 15) and regeneration experiment results showed that this kind of hybrid adsorbent not only met the requirements for the industrial removal of low levels of acetylene from ethylene, but was also very stable during the adsorption–desorption process.
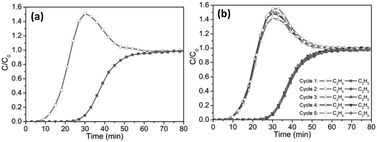 |
| Fig. 15 Breakthrough curve (a) for C2H2 (solid) and C2H4 (hollow) binary mixture separation and regeneration experiment result (b) for the 1.5-[Bmim][OAc]@MIL-101 composites. (Reproduced with permission from ref. 51. Copyright 2016, American Institute of Chemical Engineers.) | |
5.2 Heterogeneous catalysis
To date, both MOFs and TSILs have attracted wide attention in the field of heterogeneous catalysis due to several distinctive properties in their individual parts.97,99,115,116,141,142 Due to these pioneering efforts, it is a great honour that the application of MOF and TSIL hybrid materials in heterogeneous catalysis has been proposed first by our group. Herein, we have mainly discussed a few interesting phenomena, such as the confinement effect, molecular size- and shape-selective catalysis, and synergistic catalysis, in heterogeneous catalysis observed using MTHMs as catalysts.
For instance, in the acetalization of benzaldehyde with glycol as a probe reaction with a Brönsted acidic ionic liquid (BAIL) confined inside well-defined MIL-101 nanocages as a catalyst (BAIL/MIL-101), it was observed that there was an enhanced catalytic performance over the BAIL/MIL-101 catalyst as compared to the case of its homogeneous counterpart (BAIL) and pure MIL-101.29 Due to the combination of the advantageous merits of the ionic liquid and the desirable microenvironment of MIL-101, it was expected that the BAIL/MIL-101 catalyst could be used as a kind of nanoreactor, as shown in Fig. 16. In addition, a molecular size- and shape-selective catalyst by introducing an amino-functionalized basic ionic liquid (ABIL-OH) into the nanocavities of HKUST-1 was demonstrated in Knoevenagel condensation (Fig. 17).54,55 It was found that the diffusion kinetics of the reactants might be the controlling step and might play a more important role than the nature of the substituents in the confined microenvironment. Finally, we also reported that the PdCl2-ILs/CuBTC catalyst could enhance the catalytic activity in the selective oxidation of cyclohexene with molecular oxygen as an oxidant and TBHP as an initiator due to Pd–Cu synergistic catalysis (Fig. 18).56 On the other hand, it is noteworthy that the formation of coordination bonds between MOFs and TSILs play an important role in determining the stability and reusability of these catalysts.
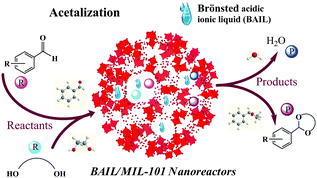 |
| Fig. 16 Brönsted acidic ionic liquid (BAIL) confined inside well-defined MIL-101 nanocages as a nanoreactor for the acetalization reaction. | |
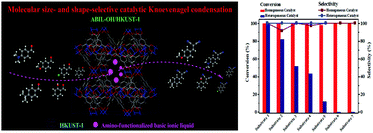 |
| Fig. 17 Molecular size- and shape-selective Knoevenagel condensation over a microporous HKUST-1 immobilized amino-functionalized basic ionic liquid catalyst (reproduced with permission from ref. 55. Copyright 2014, Elsevier B.V.). | |
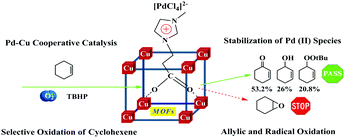 |
| Fig. 18 Selective oxidation of cyclohexene over a PdCl2-ILs/CuBTC catalyst (reproduced with permission from ref. 56. Copyright 2016, The Royal Society of Chemistry). | |
Afterwards, a variety of catalyst systems with task-specific functionalities, such as Brönsted/Lewis acidic ILs,29,53,57,59,64,67 basic ILs,54,55 acidic–basic bifunctional ILs,41,58,65,143 polyoxometalate ILs,61,66,144 as well as metal nanoparticle-62 and metal salt-containing ILs,56 involved in the corresponding acidic/basic or redox reactions (e.g. the cycloaddition of CO2 with epoxides, the esterification reaction, oxidative desulfurization, the oxidation of benzyl alcohol, Friedel–Crafts acylation, and selective hydrogenation), have been reported in the last few years. Herein, we present a summary of these research results, as listed in Table 1. Upon conducting a general survey of these literature reports, it was found that there was a common ground that these catalysts derived from the hybridization of MOFs and TSILs could significantly enhance the catalytic performance or trigger a sort of unique catalytic behaviour and maintain favourable stability and recyclability in various catalytic reactions. As a result, it was expected that MTHMs could afford a new protocol to develop MOF-based and supported TSIL heterogeneous catalysts.
Table 1 Summary towards the catalytic applications of MTHMs in different reactions and the derived catalytic behaviours
MOFs |
TSILs |
Catalytic reaction |
Catalytic behaviour |
Reference |
MIL-101 (Cr) |
Brönsted acidic quaternary ammonium salt |
Acetalization of benzaldehyde with glycol |
Enhanced catalytic performance due to confinement effect |
29
|
MIL-101 (Cr) |
Dual amino-functionalized phosphotungstic acid ionic liquid |
Selective oxidation of benzyl alcohol |
Enhanced catalytic performance |
61
|
MIL-101 (Cr) |
Quaternary ammonium salt and quaternary phosphorus salt (N(n-Bu)3 and P(n-Bu)3) |
Cycloaddition of CO2 and epoxide |
High activity under mild condition due to synergistic catalysis (Cr3+ and Br−) |
65
|
MIL-101 (Cr) |
N-Methyl-2-pyrrolidonium methyl sulfonate ([NMP]+CH3SO3−) |
Esterification of acetic acid with amyl alcohol and the Friedel–Crafts acylation of anisole |
Favourable catalytic performance and reusability |
53
|
MIL-100 (Fe) |
Heteropolyanion-based ionic liquid |
Esterification of oleic acid with ethanol |
High activity due to the coexistence of Lewis and Brönsted acid sites |
63
|
MIL-100 (Fe) |
1,4-Bis[3-(propyl-3-sulfonate)imidazolium] butane hydrogen sulphate |
Esterification reaction of oleic acid and methanol |
Catalytic activity is comparable with homogeneous IL counterparts |
57
|
MIL-100 (Fe) |
Peroxopolyoxotungstate ionic liquid |
Oxidation of benzyl alcohol |
Enhanced catalytic performance |
66
|
HKUST-1 |
1,1,3,3-Tetramethylguanidinium trifluoroacetate and Pd nanoparticles |
Selective hydrogenation of phenylacetylene |
Catalytic activity is higher than that of other Pd-supported catalyst |
62
|
HKUST-1 |
Amino-functionalized basic ionic liquid |
Knoevenagel condensation |
Molecular size- and shape-selective catalysis |
54 and 55
|
HKUST-1 |
Thiol-functionalized ionic liquid ([HVIm-(CH2)3SO3H]HSO4) |
Esterification of oleic acid with short-chain alcohols |
Catalytic activity is roughly equal to that of the same kind of catalyst |
64
|
HKUST-1 |
1-Carboxyethyl-3-methyl imidazole chloride salt and PdCl2 |
Selective oxidation of cyclohexene |
Enhanced catalytic activity due to Pd–Cu cooperative catalysis |
56
|
NH2-MIL-88B (Fe) |
1,4-Butanediyl-3,3′-bis(3-sulfopropyl) imidazolium dihydrogensulfate |
Esterification of oleic acid and ethanol |
Fine catalytic performance due to the combination of ILs and the support |
67
|
UiO-66 |
1-Methylimidazolium-3-propylsulfonate hydrosulfate (PSMIMHSO4) |
Oxidative desulfurization |
Sulfur removal is better than that of pure UiO-66 |
59
|
UiO-67 |
Imidazolium-based bromine |
Cycloaddition of epoxides and CO2 |
Favourable catalytic performance under atmospheric pressure |
41
|
ZIF-90 |
Pyridinium-based iodate |
Cycloaddition of propylene oxide and CO2 |
Enhanced catalytic activity due to synergistic catalysis (Zn2+ and I−) |
58
|
MOF-5 |
Quaternary ammonium salts (Me4NCl, Me4NBr, Et4NBr, n-Pr4NBr, n-Bu4NBr) |
Cycloaddition of epoxides and CO2 |
High yield at mild conditions due to the synergetic effect of MOF-5 and ionic liquid |
143
|
5.3 Adsorptive desulfurization
In recent years, the increasingly serious deterioration of air quality resulting from the utilization of fossil fuels and stringent environmental regulations are inexorably driving us towards a world of clean fuel. As a result, ultra-deep desulfurization from transportation fuels has become a significantly important research subject.145–147 Among these, selective adsorption desulfurization using adsorbents is considered as an efficient method to remove sulfides from transportation fuels.148–150 To date, MOFs and TSILs as adsorbents have been reported for the removal of sulfur compounds from diesel fuel, gasoline, jet fuel, and model fuels.151–157 However, only a couple of studies on adsorption desulfurization using MTHMs can be found in the current literature. For instance, Jhung and co-workers reported that 1-butyl-3-methylimidazolium chloride ionic liquid supported on MIL-101 showed a remarkable improvement in adsorption capacity (ca. 71%) as compared to the pure MIL-101 material.50 Moreover, they reported that an IL@MIL-101 composite prepared via a ship-in-bottle technique showed excellent stability and reusability in the liquid-phase adsorption of benzothiophene from liquid fuel.52 More importantly, it can be concluded from their contributions that an adequate porosity/pore size and specific adsorption sites (acid or base) are key parameters for the efficient removal of sulphur compounds.
5.4 Electrochemical behaviour
The electrode and electrolyte materials play a crucial role in electrochemistry.158–161 Both MOFs and TSILs have been studied extensively in electrochemical devices due to their unique properties (e.g. high electrochemical stability and ionic conductivity).104,105,162–166 As a result, the electrochemical applications of MTHMs are emerging as a topic of research, which involves applications in charge storage,71 ionic conduction and phase behaviour,69,70,72,73 proton conduction,68 as well as the electrochemical reduction reaction.167 For example, a new type of proton-conducting material, EIMS-HTFSA@MIL-101 (EIMS = 1-(1-ethyl-3-imidazolium)propane-3-sulfonate; HTFSA = N,N-bis(trifluoromethanesulfonyl)amide), can be prepared by impregnating the binary ionic liquid into MIL-101,68 as shown in Fig. 19. This composite material showed potential application as a safe electrolyte in proton conduction above 100 °C.
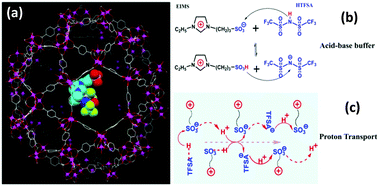 |
| Fig. 19 (a) Model structure of EIMS-HTFSA@MIL-101, (b) schematic of the binary ionic liquid EIMS-HTFSA and a Brönsted acid–base buffer, and (c) schematic of H+ hopping within the matrix of EIMS-HTFSA (reproduced with permission from ref. 68. Copyright 2017, Wiley-VCH Verlag GmbH & Co. KGaA, Weinheim). | |
In the case of ionic conduction, Kitagawa's group made a major contribution. Typically, they reported a hybrid system consisting of an ionic liquid (1-ethyl-3-methylimidazolium bis(trifluoromethylsulfonyl)amide, EMI-TFSA) and microporous ZIF-8 material. It was found that EMI-TFSA@ZIF-8 had no phase transition, and it showed higher ionic conductivity than bulky EMI-TFSA below 250 K (Fig. 20).73 Moreover, it was found that doping a lithium ion into EMI-TFSA could affect the ionic conductivity because of the exchange of Li+ and the solvating TFSA− in the micropores of ZIF-8.70 Similarly, UiO-67 and 1-ethyl-3-methylimidazolium chloride (EMIMCl) hybrid material prepared by capillary action through specific grinding and diffusing in a heating process could obtain a high ionic conductivity of 1.67 × 10−3 S cm−1 at a working temperature of 200 °C. In addition, it is worth mentioning that the combination of an MOF electrode and ionic liquid electrode can be used to efficiently catalyze the electrochemical reduction of CO2 to CH4 and generate a high current density.167
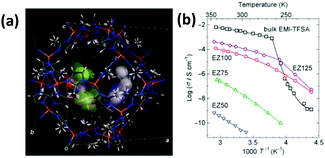 |
| Fig. 20 (a) Model crystal structure obtained from the Rietveld refinement of EZ25; (b) Arrhenius plots of the ionic conductivity of EZ50, EZ75, EZ100, EZ125 and bulky EMI-TFSA on heating (EZ is an abbreviation of EMI-TFSA@ZIF-8, and the Arabic numerals refer to the micropore volume occupied in the ZIF-8 at loadings of 50%, 75%, 100%, and 125%) (reproduced with permission from ref. 73. Copyright 2015, The Royal Society of Chemistry). | |
5.5 As precursors for the synthesis of heteroatom-doped carbon materials
In fact, it is well known that the carbonization of organic carbon-rich precursors at high temperatures is a general protocol to prepare carbonaceous materials. In this regard, MOFs, as an ideal sacrificial template for fabricating highly porous carbon-based materials, have aroused extensive attention.168–171 Moreover, together with the unique properties (negligible vapour pressure and element diversity) of ionic liquids, it is expected that introduction of ILs into MOFs can be used to form a sort of composite precursor to achieve heteroatom-doped carbon materials via pyrolysis. Very recently, the viability of this concept was confirmed by Xu's and Jhung's groups.74,172,173 Specifically, N- and B-decorated microporous carbon materials with a large surface area can be synthesized by the impregnation of 1-ethyl-3-methylimidazolium dicyanamide ([EMIM][DCN] and 1-ethyl-3-methylimidazolium tetracyanoborate [EMIM][TCB]) within the nanocages of MIL-100 (Al), followed by carbonization under given pyrolysis conditions, respectively. The resulting nanoporous carbons exhibited high CO2 and H2 uptake capacities.74 In addition, Jhung and co-workers reported that the pyrolysis of IL@ZIF-8 composites obtained via the ship-in-bottle method could produce highly porous carbons with a high nitrogen content. Notably, these porous carbon materials derived from the pyrolysis of MTHMs demonstrated a remarkable adsorptive removal of contaminants in liquid-phase adsorption.172,173
5.6 Others
Finally, MTHMs have also been involved in other applications; however, very few reports on their applications, such as in chromatography49 and tuning of the phase behaviour (e.g. decreasing the freezing point of ionic liquids) have been reported.174 Herein, we highlighted an interesting study from Kitagawa's group. They demonstrated the first example of anomalous dynamics phase behaviour concerning the freezing point of ILs via the incorporation of ILs within the micropores of MOFs. Specifically, differential scanning calorimetry and solid-state NMR measurements showed that 1-ethyl-3-methylimidazolium bis(trifluoromethylsulfonyl) (EMI-TFSA) ionic liquid inside the micropores of ZIF-8 exhibited no freezing transition down to 123 K, whereas bulk EMI-TFSA was frozen at 231 K. This anomalous dynamic phase behaviour was attributed to the nanosize effect of MOFs on ionic liquids. More importantly, it was expected that this result would offer an alternative strategy to stabilize the liquid-state of ILs in lower temperature regions.
6. Concluding remarks and outlooks
Taking into consideration all the abovementioned discussion, it can be explicitly recognized that the respective strengths of MOFs and TSILs endow a god-given opportunity and potential for their hybridization. On the other hand, their individual weaknesses have also exposed more or less limitations and challenges in the hybridization process of MOFs and TSILs and real applications. In any case, it is witnessed that the development of hybrid materials derived from MOFs and TSILs is step by step moving to a mature class of hybrid composite materials. Together with their current potential applications, it is expected that MTHM systems represent not only an excellent playground to enrich the functionality and diversity of MOFs, but also an alternative toolbox to address several practical issues in the scale-up applications of TSILs, such as the recovery, separation, purification, and recycling of TSILs.
After knowing the advantages of MTHM systems, it is also necessary to stress herein what the specific bottlenecks and challenges are in the design, synthesis, application, optimization, and fundamental understanding of MTHMs because this is of the utmost importance to determine how far MTHMs can move forward and how likely they can be applied in real and even industrial processes. In combination with our first-hand experience and state-of-the-art progress in MTHM systems, we herein attempted to put forward a few specific challenges including compatibility between MOFs and TSILs, hybridization strategy, fundamental understanding on interaction of MOFs and TSILs, as well as stability and reusability of MTHMs in real applications.
6.1 Compatibility between MOFs and TSILs
To date, a large number of MOFs and TSILs have been synthesized. However, only a few of them are available to realize the hybridization. The main reason is related to the compatibility between MOFs and TSILs. In other words, whether the host MOFs can bear guest TSILs without interference of their intrinsic structures and compositions. Therefore, the stability of MOFs, without a doubt, is a fatal factor to determine the feasibility of MTHMs. However, the robust MOF materials (with good thermal and chemical stability) synthesized to date are limited to a few representatives of MIL-101, MIL-100, UiO-66, ZIF-8, and HKUST-1. As a result, a long-term and big challenge is to design and synthesize robust MOFs. In addition, the intrinsic physicochemical properties, including polarizability, electronegativity, acidity–basicity, molecular volume and size, as well as the chemical behaviour in different solvents, of TSILs have a profound effect on the preparation of MTHMs. In this regard, while using a given MOF material, the rational selection of TSILs and hybridization strategy must be cautiously considered, especially with appropriate compatibility between them.
6.2 Hybridization strategy
In all hybridization methods, TSIL immobilization via coordination bonds has an obvious limitation since only a few MOF materials bear metal coordinatively unsaturated sites. Moreover, TSILs are bound to contain an electron-rich group, such as amino, thiol or carboxyl, with a coordination ability. In comparison, the strategy for immobilization via covalent bonds is more flexible because organic ligands usually present more accessible active-sites or functional groups to anchor TSILs. With regard to the encapsulation of TSILs, this seems to be a specific technique because of the tailor-made MTHMs, but still has certain limitations due to the strict requirements towards the precursors of the TSILs. Finally, MOF-supported TSILs obtained by intermolecular force might be more appropriate to investigate the host–guest interaction, but this is not a vigorous approach to implement in real applications because of inevitable problems of leaching of the TSILs. Overall, it is expected that the most popular and promising strategy for the hybridization of MOFs and TSILs would be immobilization via covalent bonds within the organic linkers.
6.3 Fundamental understanding on interaction of MOFs and TSILs
With regard to the interaction between MOFs and TSILs, it is a relatively complicated system due to the presence of various interaction types including strong chemical binding (coordination bonds, covalent bonds and ionic bonds) and weak chemical interaction (π–π interactions and hydrogen-bonding), as well as intermolecular forces (Coulombic force and van der Waals interaction). As a consequence, there is a general lack of fundamental understanding of these systems; this, in turn, hampers the rational selection of MOFs and TSILs to design their hybrid systems. In the case of chemical bonds, it is relatively easy to elucidate the interaction mechanism via spectroscopic characterization. In general, the formation of covalent bonds can be evidenced through FT-IR and NMR, whereas the presence of coordination bonds is usually characterized by employing X-ray photoelectron spectroscopy (XPS) and X-ray absorption spectroscopy (XAS). It is worth mentioning that, herein, paramagnetic MOFs (e.g. Cr-MOFs) are always troublesome for solid-state CP/MAS-NMR characterization due to the cut-off of signal transformation. Intermolecular interactions, however, are still suspicious in the majority of systems to date due to the lack of direct experimental evidence. Much effort has been focused on theoretical calculations and simulations to reveal the interaction mechanism. It is generally accepted that the main interaction between MOFs and TSILs is ascribed to Coulombic forces resulting from the anions of TSILs and metal clusters of MOFs. From a personal perspective, however, the existence of van der Waals interaction, π–π interaction, and hydrogen-bonding in MTHM systems cannot be excluded although there is no report in this respect. Overall, to figure out the intrinsic interaction mechanism, it is highly desired to perform more systematic studies, especially a combination of experimental and theoretical calculations. To this end, the simple model system of MTHMs and advanced spectroscopic techniques need to be involved in the follow-up work.
6.4 Stability and reusability of MTHMs in real applications
No matter what kind of application is involved in the utilization of MTHMs, the stability and reusability of MHTMs are of great importance for their applications. In particular, the solid–liquid two-phase systems involved in adsorption or catalysis are most concerned with the abovementioned two aspects. In this regard, it is necessary to provide importance to the intrinsic stability of MOFs and the leaching of TSILs from MHTMs, which are usually dependent on the reaction/adsorption media (solvent) and reactor technology (batch or continuous-flow reactor). First, it is worth mentioning that the reaction/adsorption case selected should be compatible with MTHMs because a few reactants/adsorbates (e.g. aldehyde or ketone group) with reducibility can change the valence state of the metal centre within MOFs; this leads to a collapse of the framework. Second, taking into consideration the solvent effect, the solubility and chemical behaviour (ion or molecular state) of TSILs are two key parameters to be considered while selecting the appropriate reaction/adsorption medium. For instance, if a sort of ionic liquid has a good solubility in a polar solvent, a non-polar solvent as the reaction/adsorption medium may be preferable. Finally, the leaching issue of MHTMs in a batch reactor, in principle, is more serious than that in a continuous-flow reactor. As a consequence, the stability and reusability of MHTMs might be a chronic concern, especially for scale-up applications. Based on the abovementioned analysis, together with various applications of MTHMs, it is thus speculated that the application fields involving gas-solid two-phase systems (e.g. gas adsorption and separation), or relatively deprived of severe mass-transfer and diffusion (e.g. electrochemical devices) are more suitable and promising for MTHMs.
In summary, MOF and TSIL hybrid materials are attracting significant attention and are being widely developed. Therefore, this is only the beginning, and in the next decades, we would witness a rapid development of MTHMs. This is why we wrote this critical review to address the recent advances. Hopefully, the knowledge and learning points gained from these pioneering works would serve as a modest spur to appeal to more multidisciplinary communities coming forward with their valuable contributions in the coming decades although this review is still only the tip of the iceberg.
Conflicts of interest
There are no conflicts to declare.
Acknowledgements
We are grateful to the National Natural Science Foundation of China (21573016, 21776043 and 21706155) for providing the support. Dr Luo also thanks the project funded by China Postdoctoral Science Foundation (2016M600762).
References
- J. Mallet, Trends Ecol. Evol., 2005, 20, 229–237 CrossRef PubMed.
- G. R. Huxel, Biol. Conserv., 1999, 89, 143–152 CrossRef.
- M. L. Arnold, Annu. Rev. Ecol. Syst., 1992, 23, 237–261 CrossRef.
- E. Munch, M. E. Launey, D. H. Alsem, E. Saiz, A. P. Tomsia and R. O. Ritchie, Science, 2008, 322, 1516 CrossRef CAS PubMed.
- C. Sanchez, K. J. Shea and S. Kitagawa, Chem. Soc. Rev., 2011, 40, 471–472 RSC.
- K. G. Sharp, Adv. Mater., 1998, 10, 1243–1248 CrossRef CAS.
- G. Férey, Chem. Soc. Rev., 2008, 37, 191–214 RSC.
- Y. Benveniste, Mech. Mater., 1985, 4, 197–208 CrossRef.
- J. Lian, B. Baudelet and A. A. Nazarov, Mater. Sci. Eng., A, 1993, 172, 23–29 CrossRef.
- V. F. F. Barbosa and K. J. D. MacKenzie, Mater. Res. Bull., 2003, 38, 319–331 CrossRef CAS.
-
D. R. Askeland, The Science and Engineering of Materials, Electrical Behaviour of Materials, Springer US, Boston, MA, 1996 Search PubMed.
-
D. R. Askeland, The Science and Engineering of Materials, Optical Behaviour of Materials, Springer US, Boston, MA, 1996 Search PubMed.
- J. H. Holtz and S. A. Asher, Nature, 1997, 389, 829 CrossRef CAS PubMed.
- A. K. Cheetham, C. N. R. Rao and R. K. Feller, Chem. Commun., 2006, 4780–4795 RSC.
- C. Sanchez, P. Belleville, M. Popall and L. Nicole, Chem. Soc. Rev., 2011, 40, 696–753 RSC.
- C. Kagan, D. Mitzi and C. Dimitrakopoulos, Science, 1999, 286, 945–947 CrossRef CAS PubMed.
- P. Gómez-Romero, M. Chojak, K. Cuentas-Gallegos, J. A. Asensio, P. J. Kulesza, N. Casañ-Pastor and M. Lira-Cantú, Electrochem. Commun., 2003, 5, 149–153 CrossRef.
- M. Vallet-Regí, M. Colilla and B. González, Chem. Soc. Rev., 2011, 40, 596–607 RSC.
- P. Innocenzi and B. Lebeau, J. Mater. Chem., 2005, 15, 3821–3831 RSC.
- P. Kumar and V. V. Guliants, Microporous Mesoporous Mater., 2010, 132, 1–14 CrossRef CAS.
- A. Wight and M. Davis, Chem. Rev., 2002, 102, 3589–3614 CrossRef CAS PubMed.
- J. L. Rowsell and O. M. Yaghi, Microporous Mesoporous Mater., 2004, 73, 3–14 CrossRef CAS.
- O. K. Farha and J. T. Hupp, Acc. Chem. Res., 2010, 43, 1166–1175 CrossRef CAS PubMed.
- D. Zhao, D. J. Timmons, D. Yuan and H.-C. Zhou, Acc. Chem. Res., 2010, 44, 123–133 CrossRef PubMed.
- O. M. Yaghi, M. O'keeffe, N. W. Ockwig and H. K. Chae, Nature, 2003, 423, 705–714 CrossRef CAS PubMed.
- J. Dupont, R. F. de Souza and P. A. Suarez, Chem. Rev., 2002, 102, 3667–3692 CrossRef CAS PubMed.
- J. H. Davis, Chem. Lett., 2004, 33, 1072–1077 CrossRef CAS.
- R. Giernoth, Angew. Chem., Int. Ed., 2010, 49, 2834–2839 CrossRef CAS PubMed.
- Q.-x. Luo, M. Ji, M.-h. Lu, C. Hao, J.-s. Qiu and Y.-q. Li, J. Mater. Chem. A, 2013, 1, 6530–6534 CAS.
- S. Zeng, X. Zhang, L. Bai, X. Zhang, H. Wang, J. Wang, D. Bao, M. Li, X. Liu and S. Zhang, Chem. Rev., 2017, 117, 9625–9673 CrossRef CAS PubMed.
- K. B. Sezginel, S. Keskin and A. Uzun, Langmuir, 2016, 32, 1139–1147 CrossRef CAS PubMed.
- Y. Ban, Z. Li, Y. Li, Y. Peng, H. Jin, W. Jiao, A. Guo, P. Wang, Q. Yang and C. Zhong, Angew. Chem., 2015, 127, 15703–15707 CrossRef.
- O. Tzialla, C. Veziri, X. Papatryfon, K. Beltsios, A. Labropoulos, B. Iliev, G. Adamova, T. Schubert, M. Kroon and M. Francisco, J. Phys. Chem. C, 2013, 117, 18434–18440 CAS.
- C. Casado-Coterillo, A. Fernández-Barquín, B. Zornoza, C. Téllez, J. Coronas and Á. Irabien, RSC Adv., 2015, 5, 102350–102361 RSC.
- L. Hao, P. Li, T. Yang and T.-S. Chung, J. Membr. Sci., 2013, 436, 221–231 CrossRef CAS.
- J. M. Vicent-Luna, J. J. Gutiérrez-Sevillano, J. A. Anta and S. Calero, J. Phys. Chem. C, 2013, 117, 20762–20768 CAS.
- W. Xue, Z. Li, H. Huang, Q. Yang, D. Liu, Q. Xu and C. Zhong, Chem. Eng. Sci., 2016, 140, 1–9 CrossRef CAS.
- F. d. Silva, G. Magalhães, E. d. O. Jardim, J. Silvestre-Albero, A. Sepúlveda-Escribano, D. de Azevedo and S. de Lucena, Adsorpt. Sci. Technol., 2015, 33, 223–242 CrossRef.
- Z. Li, Y. Xiao, W. Xue, Q. Yang and C. Zhong, J. Phys. Chem. C, 2015, 119, 3674–3683 CAS.
- K. M. Gupta, Y. Chen, Z. Hu and J. Jiang, Phys. Chem. Chem. Phys., 2012, 14, 5785–5794 RSC.
- L.-G. Ding, B.-J. Yao, W.-L. Jiang, J.-T. Li, Q.-J. Fu, Y.-A. Li, Z.-H. Liu, J.-P. Ma and Y.-B. Dong, Inorg. Chem., 2017, 56, 2337–2344 CrossRef CAS PubMed.
- F. P. Kinik, C. Altintas, V. Balci, B. Koyuturk, A. Uzun and S. Keskin, ACS Appl. Mater. Interfaces, 2016, 8, 30992–31005 CAS.
- F. P. Kinik, C. Altintas, V. Balci, B. Koyuturk, A. Uzun and S. Keskin, ACS Appl. Mater. Interfaces, 2016, 8, 30992–31005 CAS.
- K. M. Gupta, Y. Chen and J. Jiang, J. Phys. Chem. C, 2013, 117, 5792–5799 CAS.
- Y. Chen, Z. Hu, K. M. Gupta and J. Jiang, J. Phys. Chem. C, 2011, 115, 21736–21742 CAS.
- J.-K. Sun, H. Lin, W. Zhang, M.-R. Gao, M. Antonietti and J. Yuan, Mater. Horiz., 2017, 4, 681–687 RSC.
- Z. Li, W. Wang, Y. Chen, C. Xiong, G. He, Y. Cao, H. Wu, M. D. Guiver and Z. Jiang, J. Mater. Chem. A, 2016, 4, 2340–2348 CAS.
- R. Lin, L. Ge, H. Diao, V. Rudolph and Z. Zhu, ACS Appl. Mater. Interfaces, 2016, 8, 32041–32049 CAS.
- Q. Dai, J. Ma, S. Ma, S. Wang, L. Li, X. Zhu and X. Qiao, ACS Appl. Mater. Interfaces, 2016, 8, 21632–21639 CAS.
- N. A. Khan, Z. Hasan and S. H. Jhung, Chem. – Eur. J., 2014, 20, 376–380 CrossRef CAS PubMed.
- J. Wang, D. Xie, Z. Zhang, Q. Yang, H. Xing, Y. Yang, Q. Ren and Z. Bao, AIChE J., 2017, 63, 2165–2175 CrossRef CAS.
- N. A. Khan, Z. Hasan and S. H. Jhung, Chem. Commun., 2016, 52, 2561–2564 RSC.
- H. M. Hassan, M. A. Betiha, S. K. Mohamed, E. El-Sharkawy and E. A. Ahmed, J. Mol. Liq., 2017, 236, 385–394 CrossRef CAS.
- Q.-x. Luo, X.-d. Song, M. Ji, S.-E. Park, C. Hao and Y.-q. Li, Appl. Catal., A, 2014, 478, 81–90 CrossRef CAS.
- Q.-x. Luo, B.-w. An, M. Ji, S.-E. Park, C. Hao and Y.-q. Li, J. Porous Mater., 2015, 22, 247–259 CrossRef CAS.
- Q.-x. Luo, M. J. S.-E. Park, C. Hao and Y.-q. Li, RSC Adv., 2016, 6, 33048–33054 RSC.
- M. Han, Z. Gu, C. Chen, Z. Wu, Y. Que, Q. Wang, H. Wan and G. Guan, RSC Adv., 2016, 6, 37110–37117 RSC.
- J. Tharun, K.-M. Bhin, R. Roshan, D. W. Kim, A. C. Kathalikkattil, R. Babu, H. Y. Ahn, Y. S. Won and D.-W. Park, Green Chem., 2016, 18, 2479–2487 RSC.
- J. Wu, Y. Gao, W. Zhang, Y. Tan, A. Tang, Y. Men and B. Tang, Appl. Organomet. Chem., 2015, 29, 96–100 CrossRef CAS.
- J. Song, Z. Zhang, S. Hu, T. Wu, T. Jiang and B. Han, Green Chem., 2009, 11, 1031–1036 RSC.
- S. Abednatanzi, K. Leus, P. G. Derakhshandeh, F. Nahra, K. De Keukeleere, K. Van Hecke, I. Van Driessche, A. Abbasi, S. P. Nolan and P. Van Der Voort, Catal. Sci. Technol., 2017, 7, 1478–1487 CAS.
- L. Peng, J. Zhang, S. Yang, B. Han, X. Sang, C. Liu and G. Yang, Green Chem., 2015, 17, 4178–4182 RSC.
- H. Wan, C. Chen, Z. Wu, Y. Que, Y. Feng, W. Wang, L. Wang, G. Guan and X. Liu, ChemCatChem, 2015, 7, 441–449 CrossRef CAS.
- C. Chen, Z. Wu, Y. Que, B. Li, Q. Guo, Z. Li, L. Wang, H. Wan and G. Guan, RSC Adv., 2016, 6, 54119–54128 RSC.
- D. Ma, B. Li, K. Liu, X. Zhang, W. Zou, Y. Yang, G. Li, Z. Shi and S. Feng, J. Mater. Chem. A, 2015, 3, 23136–23142 CAS.
- S. Abednatanzi, A. Abbasi and M. Masteri-Farahani, Catal. Commun., 2017, 96, 6–10 CrossRef CAS.
- Z. Wu, C. Chen, H. Wan, L. Wang, Z. Li, B. Li, Q. Guo and G. Guan, Energy Fuels, 2016, 30, 10739–10746 CrossRef CAS.
- X. L. Sun, W. H. Deng, H. Chen, H. L. Han, J. M. Taylor, C. Q. Wan and G. Xu, Chem. – Eur. J., 2017, 23, 1248–1252 CrossRef CAS PubMed.
- L.-H. Chen, B.-B. Wu, H.-X. Zhao, L.-S. Long and L.-S. Zheng, Inorg. Chem. Commun., 2017, 81, 1–4 CrossRef CAS.
- K. Fujie, R. Ikeda, K. Otsubo, T. Yamada and H. Kitagawa, Chem. Mater., 2015, 27, 7355–7361 CrossRef CAS.
- A. Singh, R. Vedarajan and N. Matsumi, J. Electrochem. Soc., 2017, 164, H5169–H5174 CrossRef CAS.
- R. Dutta and A. Kumar, J. Phys.: Conf. Ser., 2016, 765, 1–7 CrossRef.
- K. Fujie, K. Otsubo, R. Ikeda, T. Yamada and H. Kitagawa, Chem. Sci., 2015, 6, 4306–4310 RSC.
- A. Aijaz, T. Akita, H. Yang and Q. Xu, Chem. Commun., 2014, 50, 6498–6501 RSC.
- P. Kinik, A. Uzun and S. Keskin, ChemSusChem, 2017, 10, 2842–2863 CrossRef PubMed.
- K. Fujie and H. Kitagawa, Coord. Chem. Rev., 2016, 307, 382–390 CrossRef CAS.
- I. Ahmed and S. H. Jhung, Mater. Today, 2014, 17, 136–146 CrossRef CAS.
- I. Cota and F. F. Martinez, Coord. Chem. Rev., 2017, 351, 189–204 CrossRef CAS.
-
W. Schwieger, T. Selvam, M. Klumpp and M. Hartmann, Porous Inorganic Materials as Potential Supports for Ionic Liquids, in Supported Ionic Liquids: Fundamentals and Applications, Wiley-VCH Verlag GmbH & Co. KGaA, Weinheim, Germany, 2014 Search PubMed.
- J. Juan-Alcañiz, J. Gascon and F. Kapteijn, J. Mater. Chem., 2012, 22, 10102–10118 RSC.
- S. Kitagawa, S.-i. Noro and T. Nakamura, Chem. Commun., 2006, 701–707 RSC.
- K. K. Tanabe and S. M. Cohen, Angew. Chem., 2009, 121, 7560–7563 CrossRef.
- K. K. Tanabe and S. M. Cohen, Chem. Soc. Rev., 2011, 40, 498–519 RSC.
- Z. Wang and S. M. Cohen, Chem. Soc. Rev., 2009, 38, 1315–1329 RSC.
- S. M. Cohen, Chem. Rev., 2011, 112, 970–1000 CrossRef PubMed.
- S. M. Cohen, Chem. Sci., 2010, 1, 32–36 RSC.
- A. Schneemann, V. Bon, I. Schwedler, I. Senkovska, S. Kaskel and R. A. Fischer, Chem. Soc. Rev., 2014, 43, 6062–6096 RSC.
- H. Olivier-Bourbigou, L. Magna and D. Morvan, Appl. Catal., A, 2010, 373, 1–56 CrossRef CAS.
- J. S. Wilkes, Green Chem., 2002, 4, 73–80 RSC.
- K. Marsh, J. Boxall and R. Lichtenthaler, Fluid Phase Equilib., 2004, 219, 93–98 CrossRef CAS.
- R. D. Rogers and K. R. Seddon, Science, 2003, 302, 792–793 CrossRef PubMed.
- J. F. Brennecke and E. J. Maginn, AIChE J., 2001, 47, 2384–2389 CrossRef CAS.
- T. Welton, Chem. Rev., 1999, 99, 2071–2084 CrossRef CAS PubMed.
- J. P. Hallett and T. Welton, Chem. Rev., 2011, 111, 3508–3576 CrossRef CAS PubMed.
- P. Wasserscheid and W. Keim, Angew. Chem., Int. Ed., 2000, 39, 3772–3789 CrossRef CAS PubMed.
- R. Sheldon, Chem. Commun., 2001, 2399–2407 RSC.
- T. Welton, Coord. Chem. Rev., 2004, 248, 2459–2477 CrossRef CAS.
- A. C. Cole, J. L. Jensen, I. Ntai, K. L. T. Tran, K. J. Weaver, D. C. Forbes and J. H. Davis, J. Am. Chem. Soc., 2002, 124, 5962–5963 CrossRef CAS PubMed.
- C. M. Gordon, Appl. Catal., A, 2001, 222, 101–117 CrossRef CAS.
- E. D. Bates, R. D. Mayton, I. Ntai and J. H. Davis, J. Am. Chem. Soc., 2002, 124, 926–927 CrossRef CAS PubMed.
- J. G. Huddleston, H. D. Willauer, R. P. Swatloski, A. E. Visser and R. D. Rogers, Chem. Commun., 1998, 1765–1766 RSC.
- S. Dai, Y. Ju and C. Barnes, J. Chem. Soc., Dalton Trans., 1999, 1201–1202 RSC.
- C. Ye, W. Liu, Y. Chen and L. Yu, Chem. Commun., 2001, 2244–2245 RSC.
- M. Armand, F. Endres, D. R. MacFarlane, H. Ohno and B. Scrosati, Nat. Mater., 2009, 8, 621–629 CrossRef CAS PubMed.
- M. Galiński, A. Lewandowski and I. Stępniak, Electrochim. Acta, 2006, 51, 5567–5580 CrossRef.
- A. Lewandowski and A. Świderska-Mocek, J. Power Sources, 2009, 194, 601–609 CrossRef CAS.
- J. Ding, D. Zhou, G. Spinks, G. Wallace, S. Forsyth, M. Forsyth and D. MacFarlane, Chem. Mater., 2003, 15, 2392–2398 CrossRef CAS.
- Y.-S. Ye, J. Rick and B.-J. Hwang, J. Mater. Chem. A, 2013, 1, 2719–2743 CAS.
- M. Antonietti, D. Kuang, B. Smarsly and Y. Zhou, Angew. Chem., Int. Ed., 2004, 43, 4988–4992 CrossRef CAS PubMed.
- R. E. Morris, Chem. Commun., 2009, 2990–2998 RSC.
- E. R. Parnham and R. E. Morris, Acc. Chem. Res., 2007, 40, 1005–1013 CrossRef CAS PubMed.
- E. R. Parnham and R. E. Morris, J. Am. Chem. Soc., 2006, 128, 2204–2205 CrossRef CAS PubMed.
- M. Valkenberg and W. Hölderich, Green Chem., 2002, 4, 88–93 RSC.
- A. Riisagera, R. Fehrmanna, M. Haumannb and P. Wasserscheidb, Top. Catal., 2006, 40, 91–102 CrossRef.
- C. P. Mehnert, R. A. Cook, N. C. Dispenziere and M. Afeworki, J. Am. Chem. Soc., 2002, 124, 12932–12933 CrossRef CAS PubMed.
- C. P. Mehnert, Chem. – Eur. J., 2005, 11, 50–56 CrossRef PubMed.
- B. Wu, D. Hu, Y. Kuang, B. Liu, X. Zhang and J. Chen, Angew. Chem., 2009, 121, 4845–4848 CrossRef.
- D. W. Kim and D. Y. Chi, Angew. Chem., Int. Ed., 2004, 43, 483–485 CrossRef CAS PubMed.
- N. R. Dhumal, M. P. Singh, J. A. Anderson, J. Kiefer and H. J. Kim, J. Phys. Chem. C, 2016, 120, 3295–3304 CAS.
- S. Zhang, J. Zhang, Y. Zhang and Y. Deng, Chem. Rev., 2016, 117, 6755–6833 CrossRef PubMed.
- C. P. Mehnert, E. J. Mozeleski and R. A. Cook, Chem. Commun., 2002, 3010–3011 RSC.
- B. Karimi and M. Vafaeezadeh, Chem. Commun., 2012, 48, 3327–3329 RSC.
- A. Riisager, R. Fehrmann, S. Flicker, R. van Hal, M. Haumann and P. Wasserscheid, Angew. Chem., Int. Ed., 2005, 44, 815–819 CrossRef CAS PubMed.
- Y. Zhang, Y. Shen, J. Li, L. Niu, S. Dong and A. Ivaska, Langmuir, 2005, 21, 4797–4800 CrossRef CAS PubMed.
- T. Fukushima, K. Asaka, A. Kosaka and T. Aida, Angew. Chem., Int. Ed., 2005, 44, 2410–2413 CrossRef CAS PubMed.
- K. Jin, X. Huang, L. Pang, J. Li, A. Appel and S. Wherland, Chem. Commun., 2002, 2872–2873 RSC.
- J.-H. Liao, P.-C. Wu and W.-C. Huang, Cryst. Growth Des., 2006, 6, 1062–1063 CAS.
- D. N. Dybtsev, H. Chun and K. Kim, Chem. Commun., 2004, 1594–1595 RSC.
- Z. Lin, D. S. Wragg and R. E. Morris, Chem. Commun., 2006, 2021–2023 RSC.
- J.-K. Sun, M. Antonietti and J. Yuan, Chem. Soc. Rev., 2016, 45, 6627–6656 RSC.
- Y. K. Hwang, D. Y. Hong, J. S. Chang, S. H. Jhung, Y. K. Seo, J. Kim, A. Vimont, M. Daturi, C. Serre and G. Férey, Angew. Chem., Int. Ed., 2008, 47, 4144–4148 CrossRef CAS PubMed.
- D. Y. Hong, Y. K. Hwang, C. Serre, G. Ferey and J. S. Chang, Adv. Funct. Mater., 2009, 19, 1537–1552 CrossRef CAS.
- J. F. Jansen, E. de Brabander-vanden Berg and E. Meijer, Science, 1994, 266, 1226 CAS.
- L. M. Ellerby and C. R. Nishida, Science, 1992, 255, 1113 CAS.
- T. Douglas and M. Young, Nature, 1998, 393, 152 CrossRef CAS.
- L. H. Wee, S. R. Bajpe, N. Janssens, I. Hermans, K. Houthoofd, C. E. Kirschhock and J. A. Martens, Chem. Commun., 2010, 46, 8186–8188 RSC.
- Y. Cui, T. Song, J. Yu, Y. Yang, Z. Wang and G. Qian, Adv. Funct. Mater., 2015, 25, 4796–4802 CrossRef CAS.
- H. Liu, L. Chang, C. Bai, L. Chen, R. Luque and Y. Li, Angew. Chem., Int. Ed., 2016, 55, 5019–5023 CrossRef CAS PubMed.
- G. Lu, S. Li, Z. Guo, O. K. Farha, B. G. Hauser, X. Qi, Y. Wang, X. Wang, S. Han and X. Liu, Nat. Chem., 2012, 4, 310–316 CrossRef CAS PubMed.
- S. Zhang, J. Zhang, Y. Zhang and Y. Deng, Chem. Rev., 2017, 117, 6755–6833 CrossRef CAS PubMed.
- J. Gascon, A. Corma, F. Kapteijn and F. X. Llabres i Xamena, ACS Catal., 2013, 4, 361–378 CrossRef.
- J. Lee, O. K. Farha, J. Roberts, K. A. Scheidt, S. T. Nguyen and J. T. Hupp, Chem. Soc. Rev., 2009, 38, 1450–1459 RSC.
- J. Song, Z. Zhang, S. Hu, T. Wu, T. Jiang and B. Han, Green Chem., 2009, 11, 1031–1036 RSC.
- H. Wan, C. Chen, Z. Wu, Y. Que, Y. Feng, W. Wang, L. Wang, G. Guan and X. Liu, ChemCatChem, 2015, 7, 441–449 CrossRef CAS.
- J. H. Kim, X. Ma, A. Zhou and C. Song, Catal. Today, 2006, 111, 74–83 CrossRef CAS.
- C. Song, Catal. Today, 2003, 86, 211–263 CrossRef CAS.
- M. Breysse, G. Djega-Mariadassou, S. Pessayre, C. Geantet, M. Vrinat, G. Pérot and M. Lemaire, Catal. Today, 2003, 84, 129–138 CrossRef CAS.
- W. Li, Q. Liu, J. Xing, H. Gao, X. Xiong, Y. Li, X. Li and H. Liu, AIChE J., 2007, 53, 3263–3268 CrossRef CAS.
- X. Ma, S. Velu, J. H. Kim and C. Song, Appl. Catal., B, 2005, 56, 137–147 CrossRef CAS.
- R. T. Yang, A. J. Hernández-Maldonado and F. H. Yang, Science, 2003, 301, 79–81 CrossRef CAS PubMed.
- P. S. Kulkarni and C. A. Afonso, Green Chem., 2010, 12, 1139–1149 RSC.
- S. Zhang and Z. C. Zhang, Green Chem., 2002, 4, 376–379 RSC.
- X. Wang, H. Wan, M. Han, L. Gao and G. Guan, Ind. Eng. Chem. Res., 2012, 51, 3418–3424 CrossRef CAS.
- N. A. Khan, Z. Hasan and S. H. Jhung, J. Hazard. Mater., 2013, 244, 444–456 CrossRef PubMed.
- N. A. Khan and S. H. Jhung, J. Hazard. Mater., 2012, 237, 180–185 CrossRef PubMed.
- I. Ahmed and S. H. Jhung, Chem. Eng. J., 2014, 251, 35–42 CrossRef CAS.
- N. A. Khan and S. H. Jhung, Angew. Chem., 2012, 124, 1224–1227 CrossRef.
- G. Wang, L. Zhang and J. Zhang, Chem. Soc. Rev., 2012, 41, 797–828 RSC.
- M. D. Stoller and R. S. Ruoff, Energy Environ. Sci., 2010, 3, 1294–1301 CAS.
- Y. Zhang, H. Feng, X. Wu, L. Wang, A. Zhang, T. Xia, H. Dong, X. Li and L. Zhang, Int. J. Hydrogen Energy, 2009, 34, 4889–4899 CrossRef CAS.
- P. Simon and Y. Gogotsi, Nat. Mater., 2008, 7, 845–854 CrossRef CAS PubMed.
- R. Díaz, M. G. Orcajo, J. A. Botas, G. Calleja and J. Palma, Mater. Lett., 2012, 68, 126–128 CrossRef.
- D. Wu, Z. Guo, X. Yin, Q. Pang, B. Tu, L. Zhang, Y. G. Wang and Q. Li, Adv. Mater., 2014, 26, 3258–3262 CrossRef CAS PubMed.
- P. Ramaswamy, N. E. Wong and G. K. Shimizu, Chem. Soc. Rev., 2014, 43, 5913–5932 RSC.
- J. Yang, P. Xiong, C. Zheng, H. Qiu and M. Wei, J. Mater. Chem. A, 2014, 2, 16640–16644 CAS.
- M. Ishikawa, T. Sugimoto, M. Kikuta, E. Ishiko and M. Kono, J. Power Sources, 2006, 162, 658–662 CrossRef CAS.
- X. Kang, Q. Zhu, X. Sun, J. Hu, J. Zhang, Z. Liu and B. Han, Chem. Sci., 2016, 7, 266–273 RSC.
- M. Hu, J. Reboul, S. Furukawa, N. L. Torad, Q. Ji, P. Srinivasu, K. Ariga, S. Kitagawa and Y. Yamauchi, J. Am. Chem. Soc., 2012, 134, 2864–2867 CrossRef CAS PubMed.
- H.-L. Jiang, B. Liu, Y.-Q. Lan, K. Kuratani, T. Akita, H. Shioyama, F. Zong and Q. Xu, J. Am. Chem. Soc., 2011, 133, 11854–11857 CrossRef CAS PubMed.
- B. Liu, H. Shioyama, T. Akita and Q. Xu, J. Am. Chem. Soc., 2008, 130, 5390–5391 CrossRef CAS PubMed.
- L. Oar-Arteta, T. Wezendonk, X. Sun, F. Kapteijn and J. Gascon, Mater. Chem. Front., 2017, 1, 1709–1745 RSC.
- I. Ahmed, T. Panja, N. A. Khan, M. Sarker, J.-S. Yu and S. H. Jhung, ACS Appl. Mater. Interfaces, 2017, 9, 10276–10285 CAS.
- M. Sarker, I. Ahmed and S. H. Jhung, Chem. Eng. J., 2017, 323, 203–211 CrossRef CAS.
- K. Fujie, T. Yamada, R. Ikeda and H. Kitagawa, Angew. Chem., Int. Ed., 2014, 53, 11302–11305 CrossRef CAS PubMed.
|
This journal is © the Partner Organisations 2018 |
Click here to see how this site uses Cookies. View our privacy policy here.