DOI:
10.1039/C7QI00495H
(Research Article)
Inorg. Chem. Front., 2018,
5, 120-126
A water-stable Tb(III)-based metal–organic gel (MOG) for detection of antibiotics and explosives†
Received
19th August 2017
, Accepted 1st November 2017
First published on 2nd November 2017
Abstract
The rapid detection of antibiotics and organic explosives has gradually garnered great attention, due to concerns about sustainability and the environment. In this work, a luminescent Tb-based metal organic gel (MOG(Tb) gel) was prepared by Al-based MOG induced in situ replacement of Al3+ with Tb3+. Remarkably, the strong emission of the post-synthesized MOG(Tb) xerogel can be quenched efficiently by using trace amounts of sulfamethazine (SMZ) and sulfadiazine (SDZ) even in the presence of other competing antibiotics. Furthermore, the MOG(Tb) xerogel has shown its application as a chemosensor for the highly selective detection of nitroaromatics such as 2,4-dinitrophenol (2,4-DNT) and 2,6-dinitrophenol (2,6-DNT). To further verify the recyclability of MOG(Tb), detection experiments were carried out with recovered xerogel to give a satisfactory result.
Introduction
The number of various antibiotics has reached more than one thousand and hundreds of them have been used in clinical trials. In particular, China, per capita, consumes up to 138 grams of antibiotics per year (only 13 grams in USA), and this number will gradually increase with the growth of population and the development of industry.1,2 Unfortunately, the abuse of antibiotics strongly inhibits the treatment of antibiotic resistant infections. Recent reports have already indicated that there exist some antibiotics in wastewater, even in drinking water. Such issues inevitably cause public panic. Thus the rapid and efficient detection of antibiotics in the aquatic environment has become an urgent need.
As one of the common organic pollutants and antibiotics in wastewater, nitroaromatics are highly toxic and hard to degrade naturally. Thus, monitoring these chemicals in water is very important. Although they can be detected through some techniques such as ion mobility spectrometry3 and mass spectrometry (MS),4 these instrumental methods are complex and expensive and sophisticated equipment and professionals are required. Therefore, the development of new favorable materials and reliable methods to detect such environmental pollutants is highly desirable.
Although metal–organic frameworks (MOFs)5–10 have been demonstrated to detect antibiotics,11 employing metal–organic gels (MOGs, as a new type of soft material) as active materials for such detection is unprecedented. Compared with crystalline MOFs, MOGs possess a lower density, higher surface area and larger porosity, which should benefit a lot the fast and efficient detection of antibiotics.12–15 Moreover, the research on MOGs is still in its infancy, there is still plenty of room remaining for scientists due to the uncertain factors in the structure and gelation process of MOGs.
In this sense, inspired by the metal metathesis in MOFs,16–18 the partial and irreversible metal node metathesis is achieved in MOG(Al) gels based on MIL-100(Al) particles. Through this post-synthetic strategy, a novel luminescent lanthanide MOG (MOG(Tb) gel) was obtained from a transition metal based MOG (MOG(Al) gel). As expected, the MOG(Tb) xerogel has shown highly selective and sensitive detection abilities towards antibiotics and organic explosives owing to its excellent luminescence and stability in water. What is more, the strategy shown here for preparing the MOG(Tb) gel is very simple and feasible for large-scale synthesis, which would open a novel route towards the functional applications and design of new MOG materials.
Experimental section
Materials and measurements
All chemicals and solvents are commercially available and used without further treatment. Powder X-ray diffraction (PXRD) patterns were recorded on a Rigaku Ultima IV diffractometer equipped with Cu Kα radiation (λ = 1.5406 Å) at a scan speed of 4° min−1. Thermogravimetric (TG) curves were recorded on a NETZSCH 449C thermal analyzer with a heating rate of 10 °C min−1 under a N2 atmosphere.
Fluorescence spectroscopy was conducted on a Hitachi FS5 fluorescence spectrometer. The fluorescence lifetime of the sample was measured on an FLS920 fluorescence spectrometer from Edinburgh Instruments Corp. Inductively coupled plasma (ICP) spectroscopy was performed on an Agilent 720 ICP-OES spectrometer. The UV-Vis spectra were recorded on a Shimadzu UV-2550 spectrophotometer. The nitrogen adsorption–desorption measurements were performed on a Belsorp-max instrument. Prior to the measurement, all materials were activated at 150 °C for 6 h under a dynamic vacuum.
Synthesis of the MOG(Al) gel
The MOG(Al) gel was synthesized through a simple reaction between Al(NO3)3·9H2O (0.5627 g, 1.5 mmol) and 1,3,5-benzenetricarboxylic acid (0.2101 g, 1 mmol) in ethanol (16 mL) as reported in the literature.19 The reaction was carried out in a Teflon-lined steel autoclave at 120 °C for 6 h. After cooling to room temperature, the light yellow gel was obtained and then washed with ethanol three times. For the synthesis of the homogeneous MOG(Al) gel, the mole ratio between metal sources and ligand is 3
:
2.
Synthesis of the MOG(Tb) xerogel
The MOG(Tb) gel was obtained by soaking the MOG(Tb) gel (the as-synthesized) in an ethanol solution of Tb(NO3)3·6H2O (30 mL, 3.5 mmol) for seven days (Scheme 1). After soaking, the gel was washed with ethanol to remove exterior Tb3+ ions on the surface, and then the MOG(Tb) xerogel was obtained by drying the gel at 80 °C under a dynamic vacuum for 12 h.
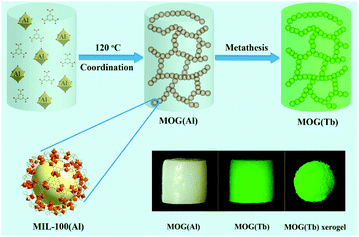 |
| Scheme 1 Schematic illustration of the synthesis of the MOG(Al) gel and the MOG(Tb) gel (bottom right: photographs of gels under UV-light irradiation). | |
Water stability test
In order to examine the stability of the MOG(Tb) xerogel in water, the as-synthesized xerogel samples were soaked in aqueous solutions of different pH values (pH = 2, 3, 5, 7, 9, 11, and 12) for 24 h. After soaking and drying, all samples were examined by powder X-ray diffraction (PXRD).
Luminescent experiments
For detecting antibiotics and organic explosives, MOG(Tb) xerogel powder (5 mg) was immersed in an aqueous solution (10 mL, 4 × 10−4 mol L−1) of analytes at room temperature. It is noteworthy that all the mixtures should be treated by ultrasonication for 30 min to produce a stable suspension.
For the experiments of selective detection, 5 mg MOG(Tb) xerogel powder was immersed in an aqueous solution (4 × 10−4 mol L−1) of sulfamethazine or sulfadiazine in the presence of another antibiotic (4 × 10−4 mol L−1). Similarly, 5 mg MOG(Tb) xerogel powder was immersed in an aqueous solution (4 × 10−4 mol L−1) of 2,6-dinitrophenol or 2,4-dinitrophenol in the presence of organic explosives (4 × 10−4 mol L−1). Prior to the measurements, the mixtures should be treated by ultrasonication for 30 min to produce a stable suspension.
Results and discussion
Characterization of the MOG(Al) xerogel and the MOG(Tb) xerogel
Compared with the crystal of MIL-100(Al), the PXRD patterns of the MOG(Al) xerogel represent low crystallinity because only several broad peaks (Fig. 1a) were observed and this result is similar to the reported xerogel. Besides the peak from the MOG(Al) xerogel, there exists an additional peak, which can be assigned to the MIL-100(Al) crystal. Clearly, compared with the MOG(Al) xerogel, there is almost no change in the PXRD pattern (Fig. 1a) of the MOG(Tb) xerogel after the central metal node exchange. The nitrogen sorption measurement has been conducted to study the porosity of the as-prepared MOG(Al) xerogel. As shown in Fig. 1b, a typical type-IV curve with a hysteresis loop, which appears between the adsorption and desorption branches at a relative pressure of P/P0 = 0.5–0.9 was clearly observed, indicating the existence of a certain degree of mesoporous structure in the MOG(Al) xerogel. The pore-size distribution of the MOG(Al) xerogel, which is obtained from the analysis of the nitrogen isotherm by using the NLDFT method, is about 3.1 nm and the BET surface area is about 1421.9 m2 g−1.
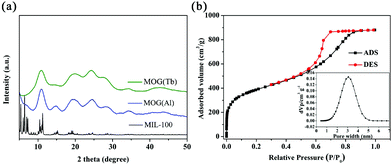 |
| Fig. 1 (a) PXRD patterns of MIL-100(Al), the MOG(Al) xerogel, and the MOG(Tb) xerogel. (b) N2 adsorption/desorption isotherms of the MOG(Al) xerogel (the inset shows the pore size distribution). | |
The TG analysis curves of the MOG(Al) xerogel and the MOG(Tb) xerogel (Fig. S1†) show their similar thermal stabilities. There are two major weight loss processes from 25 to 600 °C. The first weight loss at 25–150 °C corresponds to the solvent evaporation and the second stage weight loss may be attributed to the decomposition of organic ligands.
In order to verify the metal node metathesis in the MOG(Al) gel, the compositions of the MOG(Al) xerogel and the MOG(Tb) xerogel have been examined through ICP-OES equipment. The contents of Al3+ and Tb3+ ions in the xerogel have been summarized in Table S1.† Obviously, the content of Al3+ ions decreases significantly with the metathesis process, while the real content of Tb3+ ions increases from 0 to 2.4 mmol g−1 which is highly consistent with the reduced content of Tb3+ ions. The results indicated the fact of metal node metathesis in the MOG(Al) gel. Central aluminum ion exchange might be considered as being dominated by the stronger coordination ability of terbium ions to carboxylate groups.
Luminescence properties of the MOG(Tb) xerogel
Compared to the weak fluorescence emission at 387 nm (ex = 340 nm) of the independent H3btc ligand, the coordination between Tb3+ and H3btc in the MOG(Tb) gel leads to an intense fluorescence emission at 615, 585, 544, and 491 nm (ex = 257 nm) as a result of ligand-to-metal charge transfer (Fig. S2†).8c After the metal node exchange, the product possesses characteristic emission of Tb3+ as we expected. As shown in Fig. 2, the evident characteristic peaks of the MOG(Tb) xerogel are located at 615, 585, 544, and 491 nm, which are ascribed to the f–f transitions of Tb3+ (5D4 → 7FJ, J = 3–6).20,21 In addition, the MOG(Tb) xerogel shows strong green luminescence under UV-light, as shown in the inset of Fig. 2, indicating the occurrence of an antenna effect. Furthermore, the MOG(Tb) xerogel possesses a long decay lifetime of up to 1.27 ns and a high quantum yield as much as 18.5% owing to the efficacious energy transfer from the ligand to the Tb3+ ions.
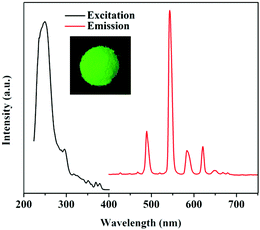 |
| Fig. 2 Excitation and emission spectra of the MOG(Tb) xerogel. The inset is the picture of the MOG(Tb) xerogel under UV-light irradiation. | |
Moreover, as proved by PXRD in Fig. 3, even after soaking in aqueous solution with a pH range of 2–12 for 24 h, the framework of the MOG(Tb) xerogel remains intact. In addition, the BET of the MOG(Tb) xerogel and the MOG(Tb) xerogel after treatment with an aqueous solution of pH = 2/12 shows no obvious change (Fig. S3†). Thus, it becomes very promising for the MOG(Tb) xerogel to become a novel sensing material in a water-related environmental system owing to its excellent luminescence and chemical stability in water.
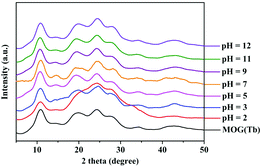 |
| Fig. 3 PXRD patterns of the MOG(Tb) xerogel upon the treatment of soaking in aqueous solution with different pH values for 24 h. | |
Detection of antibiotics and nitroaromatic compounds
Considering its excellent water stability and luminescence properties, the MOG(Tb) xerogel has been explored to detect trace amounts of antibiotics in water. Here, five kinds of commonly used antibiotics including sulfonamides (sulfamethazine, SMZ; sulfadiazine, SDZ), NFs (furazolidone, FZD), NMs (metronidazole, MDZ; dimetridazole, DTZ; ornidazole, ODZ; ronidazole, RDZ), chloramphenicols (chloramphenicol, CAP; florfenicol, FFC), and β-lactams (Penicillin G Potassium, PCLP) were selected and tested. As shown in Fig. 4, SMZ and SDZ possess the highest quenching efficiencies of up to 99.8% and 99.2%, respectively, while the quenching efficiencies of FZD and CAP are intermediate. The remaining antibiotics have low quenching efficiencies. In other words, the MOG(Tb) xerogel is more sensitive to sulfonamides than FFC. The trend of the quenching efficiency of these antibiotics follows the sequence: SMZ > SDZ > FZD > CAP > MDZ > DTZ > ODZ > PCLP > RDZ > FFC. It should be noted that the framework of the MOG(Tb) xerogel did not show any obvious change after detection experiments (Fig. S4†).
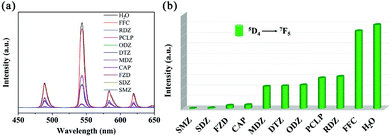 |
| Fig. 4 (a) Emission spectra of the MOG(Tb) xerogel in aqueous solutions of various antibiotics and (b) the corresponding fluorescence intensity at 544 nm. | |
In order to further understand the luminescent responses of the MOG(Tb) xerogel toward sulfonamides better, the changes of the fluorescence intensity of the MOG(Tb) xerogel with the increase of the sulfonamide concentration from 0 to 0.4 mM (Fig. 5a and c) were examined. The quenching effect can be quantitatively rationalized by using the Stern–Volmer (SV) equation:
where
I0 and
I are the luminescence intensities of the MOG(Tb) xerogel suspension without and with adding a certain concentration of sulfonamide solution, respectively. [M] is the molar concentration of sulfonamides and
KSV is the quenching constant.
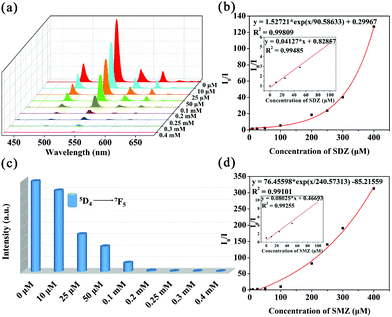 |
| Fig. 5 Emission spectra of the MOG(Tb) xerogel in aqueous solutions of SDZ (a) and SMZ (c), KSV curves of the MOG(Tb) xerogel in aqueous solutions in the presence of SDZ (b) and SMZ (d). | |
Apparently, the SV plots of SMZ and SDZ are not linear, which might be attributed to self-absorption or the process of energy-transfer;11,22,23 however, at low concentration ranges the SV plots are almost linear (Fig. 5b and d). The MOG(Tb) xerogel has the highest KSV values of 8.0 × 104 and 4.1 × 104 M−1 towards SMZ and SDZ, respectively, and the values of the detection limit (3Sb/KSV, Sb is the standard deviation) towards SMZ and SDZ are 0.086 and 0.218 ppm, respectively (Table S2†). The KSV values for SMZ and SDZ in the MOG(Tb) xerogel are also higher than the reported famous MOF materials, demonstrating their excellent quenching ability (Table S3†).
The ability of selective detection of the MOG(Tb) xerogel is very important for its practical application. Inspired by the high quenching efficiencies of the MOG(Tb) xerogel towards sulfonamides but very poor quenching efficiencies towards other antibiotics, the selective detection of the MOG(Tb) xerogel towards sulfonamides was explored. In a control experiment, we tested the fluorescence spectra of the MOG(Tb) xerogel, which was dispersed in water firstly. Then upon the addition of other antibiotics (0.4 mM) and sulphonamides (0.4 mM) successively, the corresponding emissions were monitored. As shown in Fig. 6, after the introduction of SMZ or SDZ into the mixtures of the MOG(Tb) xerogel and other antibiotics, the fluorescence quenches almost completely implying that the influence of other antibiotics can be neglected. The above results confirm the great selectivity and sensitivity of the MOG(Tb) xerogel towards sulfonamides. As for the detection of nitroaromatics with MOFs, there is a lot of effort going into this research.24,25 However, most of these detection experiments are conducted in organic solvents which greatly frustrates the possibility of practical application. Thus, a combination of water stability and excellent fluorescence properties would make the MOG(Tb) xerogel very suitable for the application of detecting nitroaromatics in water. For comparison, some aliphatic nitro compounds and other aromatics were also tested. Here, eleven detected objects including 2,4-dinitrophenol (2,4-DNT), 2,6-dinitrophenol (2,6-DNT), 2,4,6-trinitrophenol (TNP), nitrobenzene (NB), 4-nitrophenol (4-NP), phenol (PHL), benzoic acid (BC), nitromethane (NM), chlorobenzene (CB), methylbenzene (MB), and 2,3-dimethyl-2,3-dinitrobutane (DMNB) were selected to explore their influence on the fluorescence properties of the MOG(Tb) xerogel in water. The quenching efficiency of the MOG(Tb) xerogel towards analytes (Fig. 7) reveals that almost complete fluorescence quenching of the MOG(Tb) xerogel occurs towards 2,4-DNT and 2,6-DNT while little quenching effect was observed for DMNB. Visibly, 2,4-DNT and 2,6-DNT lead to the highest quenching efficiencies of up to 99.4% and 98.8%, respectively. Moreover, the quenching degrees of TNP, NB, and 4-NP all appear relatively high, while the remaining analytes lead to small quenching efficiencies. The degree of quenching efficiencies of these analytes follows the order: 2,4-DNT > 2,6-DNT > TNP > NB > 4-NP > PHL > BC > NM > CB > MB > DMNB. It is worth noting that the framework of the MOG(Tb) xerogel doesn't show any change after detection, as shown in Fig. S5.†
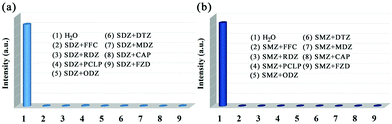 |
| Fig. 6 (a) Fluorescence intensity (λ = 544 nm) of the MOG(Tb) xerogel (5 mg) in the solutions (0.4 mM) of SDZ and SMZ (b) in the presence of other antibiotics (0.4 mM). | |
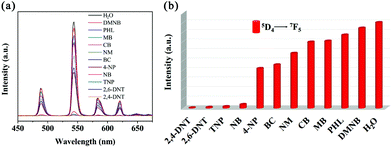 |
| Fig. 7 (a) Emission spectra of the MOG(Tb) xerogel in aqueous solutions of various analytes and (b) the corresponding fluorescence intensity at 544 nm. | |
The same situation occurs in the detection of nitroaromatics, the SV plots of 2,4-DNT and 2,6-DNT are not linear, however, at low concentration ranges the SV plots are almost linear (Fig. 8b and d). The KSV values of the MOG(Tb) xerogel towards 2,4-DNT and 2,6-DNT are 1.4 × 104 and 4.1 × 103 M−1, respectively (Table S2†). The detection limits towards 2,4-DNT and 2,6-DNT are estimated to be 1.115 and 1.589 ppm, respectively (Table S2†). The KSV values for 2,4-DNT and 2,6-DNT in the MOG(Tb) xerogel are comparable to the reported related materials, demonstrating their good quenching ability (Table S3†). Moreover, we also explore the influence of other analytes (TNP, 4-NP, NB, PHL, DMNB, MB, CB, BC, and NM) on the detection of the MOG(Tb) xerogel towards 2,4-DNT or 2,6-DNT.
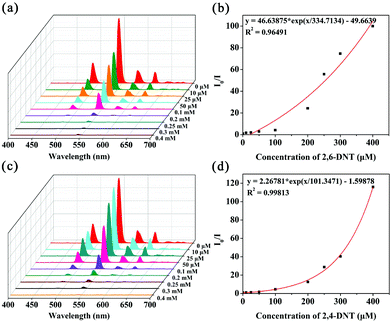 |
| Fig. 8 Emission spectra of the MOG(Tb) xerogel in aqueous solutions of 2,6-DNT (a) and 2,4-DNT (c), KSV curves of the MOG(Tb) xerogel in aqueous solutions in the presence of 2,6-DNT (b) and 2,4-DNT (d). | |
The fluorescence of the MOG(Tb) xerogel quenches almost completely (Fig. 9) upon the introduction of 2,4-DNT or 2,6-DNT into the mixture of the MOG(Tb) xerogel and other analytes, which suggests that the influence of other analytes can be neglected. The above results reveal the excellent selectivity and selectivity of the MOG(Tb) xerogel towards 2,4-DNT and 2,6-DNT.
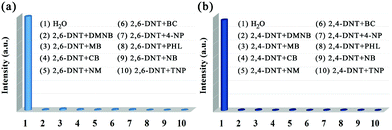 |
| Fig. 9 Fluorescence intensity (λ = 544 nm) of the MOG(Tb) xerogel (5 mg) in the solutions (0.4 mM) of 2,6-DNT (a) and 2,4-DNT (b) in the presence of other analytes (0.4 mM). | |
It is worth noting that the MOG(Tb) xerogel can be easily recovered from the reaction solution by filtration and regenerated through washing with ethanol several times. The quenching efficiencies remain largely unchanged up to six cycles, indicating good recyclability and stability of the MOG(Tb) xerogel for these detection applications (Fig. 10). It is worth noting that the framework of the MOG(Tb) xerogel remains intact after six cycles, as confirmed by ICP and PXRD, as shown in Table S4 and Fig. S6 respectively.†
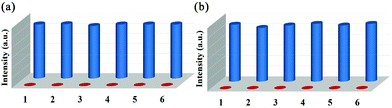 |
| Fig. 10 Reproducibility of the quenching ability of the MOG(Tb) xerogel dispersed in water in the presence of 0.4 mM aqueous solutions of (a) SMZ and (b) 2,4-DNT, respectively (the blue cylinders represent the initial fluorescence intensity and the red cylinders represent the intensity upon addition of an aqueous solution of 0.4 mM SMZ or 2,4-DNT). | |
Mechanism of fluorescence quenching responses
In order to realize the quenching mechanism of sulfonamides and nitroaromatics leading to the fluorescence quenching of the MOG(Tb) xerogel, investigative tests have been performed. Hence, we recorded the UV-Vis spectra of the analytes and the MOG(Tb) xerogel, as shown in Fig. S7 and 8.† Obviously, the absorption band of sulfonamides has the maximum degree of overlapping with the excitation band of the MOG(Tb) xerogel, which can lead to lower absorption of the ligand of the MOG(Tb) xerogel. This implies that the stronger quenching effect on the fluorescence intensity of MOG(Tb) towards sulfonamides originates from the energy-transfer process between the ligands and the metal center. For nitroaromatics and non-nitroaromatics, the nitroaromatics have the maximum overlap. Obviously, the quenching efficiencies of the MOG(Tb) xerogel towards both antibiotics and nitroaromatics are highly consistent with the degree of overlapping. According to the above results, we can speculate the possible sensing mechanism: the luminescence quenching of MOG(Tb) by sulfonamides, 2,4-DNT, and 2,6-DNT, can be attributed to the competition between them and the ligand of the MOG(Tb) xerogel. Sulfonamides, 2,4-DNT, and 2,6-DNT absorb the excitation energy and decrease the light absorbed by the ligand, hence the energy transfer from the ligand to Tb3+ ions is reduced and subsequently the characteristic luminescence of Tb3+ is quenched.
Conclusions
In conclusion, we designed and synthesized a fluorescent MOG(Tb) gel with good stability by post-synthetic metal node metathesis in the MOG(Al) gel. As a result, the MOG(Tb) xerogel exhibits sensitive detection ability effectively towards SMZ, SDZ, 2,4-DNT, and 2,6-DNT over other analytes with low detection limits of 0.086, 0.218, 1.115, and 1.589 ppm, respectively. The high degree quenching efficiencies for the antibiotics and nitroaromatics can be attributed to the competition of light adsorption. Moreover, we strongly believe that our strategy can be a good guideline to develop novel MOGs with novel applications.
Conflicts of interest
There are no conflicts to declare.
Acknowledgements
This work was financially supported by the National Natural Science Foundation of China (Grant No. 21671119, 21673127 and 21733122). Q. Z. acknowledges financial support from AcRF Tier1 (RG133/14 and RG 13/15) from MOE, Singapore.
Notes and references
- K. Kümmerer, Chemosphere, 2009, 75, 417–434 CrossRef PubMed.
- Q. Q. Zhang, G. G. Ying, C. G. Pan, Y. S. Liu and J. L. Zhao, Environ. Sci. Technol., 2015, 49, 6772–6782 CrossRef CAS PubMed.
- M. Tabrizchi and V. Ilbeigi, J. Hazard. Mater., 2010, 176, 692–696 CrossRef CAS PubMed.
- K. Håkansson, R. V. Coorey, R. A. Zubarev, V. L. Talrose and P. Håkansson, J. Mass Spectrom., 2000, 35, 337–346 CrossRef.
-
(a) G. Férey, C. Mellot-Draznieks, C. Serre, F. Millange, J. Dutour, S. Surblé and I. Margiolaki, Science, 2005, 309, 2040–2042 CrossRef PubMed;
(b) Y. Bai, Y. B. Dou, L. H. Xie, W. Rutledge, J. R. Li and H. C. Zhou, Chem. Soc. Rev., 2016, 45, 2327–2367 RSC;
(c) J. W. Liu, L. F. Chen, H. Cui, J. Y. Zhang, L. Zhang and C. Y. Su, Chem. Soc. Rev., 2014, 43, 6011–6061 RSC;
(d) Q. G. Zhai, X. H. Bu, X. Zhao, D. S. Li and P. Y. Feng, Acc. Chem. Res., 2017, 50, 407–417 CrossRef CAS PubMed;
(e) D. S. Li, Y. P. Wu, J. Zhao, J. Zhang and J. Y. Lu, Coord. Chem. Rev., 2014, 261, 1–27 CrossRef CAS.
-
(a) A. H. Chughtai, N. Ahmad, H. A. Younus, A. Laypkov and F. Verpoort, Chem. Soc. Rev., 2015, 44, 6804–6849 RSC;
(b) F. Wang, Z. S. Liu, H. Yang, Y. X. Tan and J. Zhang, Angew. Chem., Int. Ed., 2011, 50, 450–453 CrossRef CAS PubMed;
(c) Y. J. Tang, M. R. Gao, C. H. Liu, S. L. Li, H. L. Jiang, Y. Q. Lan, M. Han and S. H. Yu, Angew. Chem., Int. Ed., 2015, 54, 12928–12932 CrossRef CAS PubMed.
-
(a) K. Manna, P. F. Ji, F. X. Greene and W. B. Lin, J. Am. Chem. Soc., 2016, 138, 7488–7491 CrossRef CAS PubMed;
(b) J. D. Xiao, Q. C. Shang, Y. J. Xiong, Q. Zhang, Y. Luo, S. H. Yu and H. L. Jiang, Angew. Chem., Int. Ed., 2016, 55, 1–6 CrossRef.
-
(a) L. E. Kreno, K. Leong, O. K. Farha, M. Allendorf, R. P. Van Duyne and J. T. Hupp, Chem. Rev., 2012, 112, 1105–1125 CrossRef CAS PubMed;
(b) H. H. Wang, L. Hou, Y. Z. Li, C. Y. Jiang, Y. Y. Wang and Z. H. Zhu, ACS Appl. Mater. Interfaces, 2017, 9, 17969–17976 CrossRef CAS PubMed;
(c) Y. J. Cui, Y. F. Yue, G. D. Qian and B. L. Chen, Chem. Rev., 2012, 112, 1126–1162 CrossRef CAS PubMed;
(d) S. Khatua, S. Goswami, S. Biswas, K. Tomar, H. S. Jena and S. Konar, Chem. Mater., 2015, 27, 5349–5360 CrossRef CAS.
-
(a) Z. C. Hu, B. J. Deibert and J. Li, Chem. Soc. Rev., 2014, 43, 5815–5840 RSC;
(b) J. Zhao, Y. N. Wang, W. W. Dong, Y. P. Wu, D. S. Li, B. Liu and Q. C. Zhang, Chem. Commun., 2015, 51, 9479–9482 RSC;
(c) J. Zhao, W. W. Dong, Y. P. Wu, Y. N. Wang, C. Wang, D. S. Li and Q. C. Zhang, J. Mater. Chem. A, 2015, 3, 6962–6969 RSC.
-
(a) M. G. Campbell, D. Sheberla, S. F. Liu, T. M. Swager and M. Dincă, Angew. Chem., Int. Ed., 2015, 54, 4349–4352 CrossRef CAS PubMed;
(b) Y. J. Cui, B. Li, H. J. He, W. Zhou, B. L. Chen and G. D. Qian, Acc. Chem. Res., 2016, 49, 483–493 CrossRef CAS PubMed;
(c) G. W. Xu, Y. P. Wu, W. W. Dong, J. Zhao, X. Q. Wu, D. S. Li and Q. C. Zhang, Small, 2017, 13, 1602996 CrossRef PubMed.
-
(a) B. Wang, X. L. Lv, D. W. Feng, L. H. Xie, J. Zhang, M. Li, Y. B. Xie, J. R. Li and H. C. Zhou, J. Am. Chem. Soc., 2016, 138, 6204–6216 CrossRef CAS PubMed;
(b) M. L. Han, G. X. Wen, W. W. Dong, Z. H. Zhou, Y. P. Wu, J. Zhao, D. S. Li, L. F. Ma and X. Bu, J. Mater. Chem. C, 2017, 5, 8469–8474 RSC.
- Y. R. Liu, L. S. He, J. Y. Zhang, X. B. Wang and C. Y. Su, Chem. Mater., 2009, 31, 557–563 CrossRef.
-
(a) L. Li, S. L. Xiang, S. Q. Cao, J. Y. Zhang, G. F. Ouyang, L. P. Chen and C. Y. Su, Nat. Commun., 2013, 4, 1774–1782 CrossRef PubMed;
(b) J. Y. Zhang and C. Y. Su, Coord. Chem. Rev., 2013, 257, 1373–1408 CrossRef CAS.
-
(a) A. Mahmood, W. Xia, N. Mahmood, Q. F. Wang and R. Q. Zou, Sci. Rep., 2015, 5, 10556 CrossRef PubMed;
(b) H. B. Aiyappa, S. Saha, P. Wadge, R. Banerjee and S. Kurungot, Chem. Sci., 2015, 6, 603–607 RSC.
-
(a) P. Sutar and T. K. Maji, Chem. Commun., 2016, 52, 8055–8074 RSC;
(b) X. Zhao, L. Yuan, Z. Q. Zhang, Y. S. Wang, Q. Yu and J. Li, Inorg. Chem., 2016, 55, 5287–5296 CrossRef CAS PubMed.
-
(a) M. Lalonde, W. Bury, O. Karagiaridi, Z. Brown, J. T. Hupp and O. K. Farha, J. Mater. Chem. A, 2013, 1, 5453–5468 RSC;
(b) C. K. Brozek and M. Dincă, Chem. Soc. Rev., 2014, 43, 5456–5467 RSC.
- J. Yang, X. Q. Wang, F. N. Dai, L. L. Zhang, R. M. Wang and D. F. Sun, Inorg. Chem., 2014, 53, 10649–10653 CrossRef CAS PubMed.
- K. S. Asha, R. Bhattacharjee and S. Mandal, Angew. Chem., Int. Ed., 2016, 55, 11528–11532 CrossRef CAS PubMed.
- W. Xia, X. M. Zhang, L. Xu, Y. X. Wang, J. H. Lin and R. Q. Zou, RSC Adv., 2013, 3, 11007–11013 RSC.
- H. Y. Li, Y. L. Wei, X. Y. Dong, S. Q. Zang and T. C. W. Mak, Chem. Mater., 2015, 27, 1327–1331 CrossRef CAS.
- J. Zhao, Y. N. Wang, W. W. Dong, Y. P. Wu, D. S. Li and Q. C. Zhang, Inorg. Chem., 2016, 55, 3265–3271 CrossRef CAS PubMed.
-
(a) S. Pramanik, C. Zheng, X. Zhang, T. J. Emge and J. Li, J. Am. Chem. Soc., 2011, 133, 4153–4155 CrossRef CAS PubMed;
(b) X. H. Zhou, L. Li, H. H. Li, A. Li, T. Yang and W. Huang, Dalton Trans., 2013, 42, 12403–12409 RSC;
(c) S. S. Nagarkar, A. V. Desai and S. K. Ghosh, Chem. Commun., 2014, 50, 8915–8918 RSC.
-
(a) Z. Q. Shi, Z. J. Guo and H. G. Zheng, Chem. Commun., 2015, 51, 8300–8303 RSC;
(b) S. S. Nagarkar, B. Joarder, A. K. Chaudhari, S. Mukherjee and D. S. K. Ghosh, Angew. Chem., Int. Ed., 2013, 52, 2881–2885 CrossRef CAS PubMed;
(c) L. H. Cao, F. Shi, W. M. Zhang, S. Q. Zang and T. C. W. Mark, Chem. – Eur. J., 2015, 21, 15705–15712 CrossRef CAS PubMed.
- B. Gole, A. K. Bar and P. S. Mukherjee, Chem. – Eur. J., 2014, 20, 2276–2291 CrossRef CAS PubMed.
- T. K. Pal, N. Chatterjee and P. K. Bharadwaj, Inorg. Chem., 2016, 55, 1741–1747 CrossRef CAS PubMed.
Footnotes |
† Electronic supplementary information (ESI) available: Details of ICP measurement results, TG analysis, powder X-ray diffraction (PXRD) patterns and other electronic formats. See DOI: 10.1039/c7qi00495h |
‡ These authors contributed equally to this work. |
|
This journal is © the Partner Organisations 2018 |
Click here to see how this site uses Cookies. View our privacy policy here.