Enhanced phosphorescence properties of a Pt-porphyrin derivative fixed on the surface of nano-porous glass†
Received
14th December 2017
, Accepted 16th March 2018
First published on 16th April 2018
Abstract
The room-temperature phosphorescence chromophore, Pt(II) coproporphyrin I (PtCP), was fixed on the surface of a 3D-network of nanoscale pores of porous glass through ion-exchange reaction. The absorption and phosphorescence spectra indicated that PtCP can be loaded while maintaining monomeric dispersion at concentrations well beyond solubility limits of PtCP in solution. The phosphorescence quantum yield of PtCP fixed on the surface was also found to have double the enhancement of solution. The extended lifetime of phosphorescence of PtCP bonded on the surface compared to that in solution clearly indicated that suppression of nonradiative deactivation plays a key role in high quantum yield and long triplet lifetime. This hybridization with nano-porous glass provides opportunities for various potential applications.
Introduction
Some chromophores like metalloporphyrins are known to show room-temperature (RT) phosphorescence, which has attracted considerable attention due to various applications such as oxygen1–4 and pressure sensors,4,5 organic light-emitting diodes (OLED),6–11 and sensitizers for wavelength conversion devices12–17 based on triplet–triplet annihilation upconversion (TTA-UC) utilising high triplet quantum yield. Solid materials are preferable for such device applications; however, many RT-phosphorescence chromophores easily aggregate18 and cause phosphorescence quenching. Therefore, it is important to develop a method for achieving molecular-dispersed chromophores in the solid state at high concentration, retaining phosphorescence properties at the monomeric level.
One strategy to prevent aggregation is by fixing chromophores on the surface of a solid platform while maintaining molecular dispersion. Using a rigid fixing process, such as chemical bonding, each chromophore is bonded at a specific point of the solid surface while prohibiting their migration, which prevents further aggregation on the surface. Moreover, by placing chromophores on the solid surface rather than in the bulk solid, the chromophores are exposed to free space that can be filled with the target matter, i.e. gases, liquids, or solids that can receive excitation energy from the chromophores for sensing and other purposes. Possible solid platforms include glass, oxides such as TiO2, and nano-sheet materials like clay. The luminescence properties of chromophores, especially porphyrins, hybridized with such materials have been studied extensively. For example, it was reported that porphyrin derivatives could be fixed on the surface of clay nano-sheets without aggregation and exhibited monomeric fluorescence.19 Nano-sheet materials, however, are not suitable for the device applications mentioned above because they are contained in a dispersed solution or stacked as solids.
Nano-porous glass20 is a material with unique properties. It has nm-scale pores connected to each other and forms a 3D-network of nano-pores, enabling an extremely large pore surface area per volume and a large free volume into which other materials like gases and fluids can penetrate. Nano-porous glass could be fabricated through phase-separation technology20 from soda borosilicate glass capable of an average pore diameter of 30 nm or more. It was considered then that this material has potential for use as a solid platform for fixing chromophores.
In this work, we use nano-porous glass as a solid platform for fixing RT-phosphorescence chromophores and investigated the phosphorescence properties of chromophores bonded on the solid surface. For the chromophore, we chose Pt(II) coproporphyrin I (PtCP, Fig. 1a), a tetracarboxylic-acid derivative of Pt(II)-octaethylporphyrin (PtOEP), which is widely used as an oxygen sensor,1–3 a red OLED pigment,6–8 and a TTA-UC sensitizer.12–15 For anionic carboxylic-acid groups, it is possible to bind chromophores to the surface of the nano-pore through ionic interaction after replacing silanol groups on the glass surface with cationic amino groups.21 This hybrid material can be equipped with the various features discussed above such as strong absorption due to multiple interfaces along the optical path in the 3D-pore-network like bulk-hetero-junctions in organic photovoltaic devices, high loading concentration and a possibility of monomeric dispersion due to the large surface area and free volumes, allowing the other materials to penetrate. We found that the chromophores can retain their monomeric nature in the hybrid and show superior properties to those in solution, namely higher phosphorescence quantum yield at higher loading concentration beyond solubility in solution. Phosphorescence lifetime measurements clarify that suppression of nonradiative deactivation due to the fixing of chromophores on the solid surface is a key to superior phosphorescence properties.
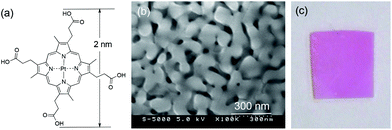 |
| Fig. 1 (a) Chemical structure of PtCP. (b) SEM image of the nano-porous glass. (c) Appearance of the PtCP-fixed porous glass. | |
Experimental
Nano-porous glass was prepared by a previously reported method.21 Borosilicate glass with a composition of SiO2:62.5%-B2O3:28.3%-Na2O:9.2% in weight (Akagawa glass, 20 × 20 × 0.5 mm3) was cut into pieces (10 × 10 × 0.5 mm3), and then heated at 600 °C for 72 h in air to induce nanoscopic phase separation between SiO2 and B2O3 in each piece. The B2O3 phase was leached from the glass by treatment with 0.5 mol l−1 sulfuric acid at 99 °C for 96 h, followed by drying at 140 °C for 3 h under vacuum. The pore size distribution was carried out by mercury porosimetry22 (Thermo Scientific Pascal 240) in the pressure range of 0 to 200 MPa. This is the method in which the intrusion of mercury into a porous sample as a function of the applied pressure is analyzed and gives more precise information than the nitrogen adsorption method in the pore range of several 10 nm.23 The average diameter of the formed nano-pores was 55.6 nm and the surface area was 35.2 m2 g−1. The nano-porous glass was then refluxed with 3-aminopropyldimethylethoxysilane as a coupling agent and rinsed in toluene at 99 °C for 3 h. PtCP ((3,8,13,18-tetramethylporphyrin-2,7,12,17-tetrapropionato)platinum(II), Frontier Scientific C40425) was used without further purification. To fix PtCP on the surface of the nano-porous glass, pieces of the glass were dipped in a PtCP solution (75 μM) in tetrahydrofuran (THF). In the cases where the loading concentration of PtCP was higher than 1 mM, the solution was additionally refluxed for 3 h. The loading concentration of PtCP was controlled by the dipping (and reflux) time. The glass pieces were then rinsed in THF at 60 °C for several hours to remove unreacted PtCP. Through this treatment, the surface area was reduced, but remained in the same order of magnitude as the original sample. This was followed by optical measurements of the PtCP-fixed porous glass.
UV-vis absorption spectra were recorded using a Shimadzu UV3150 spectrophotometer. The phosphorescence spectrum and quantum yield (ΦP) were measured using a spectrometer combined with a calibrated integrating sphere (Hamamatsu Photonics, C9920)24 under air or Ar (99.99% + ). Phosphorescence decay was measured with a custom setup using a frequency-doubled ns-Nd:YAG laser (532 nm, a pulse width of 20 ns, a repetition rate of 10–15 Hz) as the light source. The incident pulse energy was 11 μJ or as noted otherwise. A piece of the dye-fixed glass placed in a sealed glass tube under either Ar or vacuum was used as the sample. The emission from the sample was collected by using a lens and guided to a monochromator (Jobin-Yvon H-10) through an OD4 notch filter to block 532 nm scattering. The signal was detected using a photomultiplier (Hamamatsu R936) and recorded using a digital oscilloscope (500 MHz, 5 Gs s−1).
All the optical measurements were performed at 23 °C.
Results
UV-vis absorption spectrum
The prepared porous glass contained 3D-continuous pores of nm-dimensions as seen in the scanning electron microscopy (SEM) image (Fig. 1b). The nano-porous glass before dye-fixing (blank) was snow-white due to light scattering, and pink after the PtCP was fixed (Fig. 1c). The UV-vis absorption spectrum of the blank porous glass showed a monotonic increase with decreasing wavelength, which is a characteristic feature of light scattering (Fig. 2a). On the other hand, the spectrum of PtCP-fixed porous glass showed a sharp peak overlapping the scattering spectrum. The differential spectrum showed a strong peak at 534 nm and a weak peak at 501 nm (Fig. 2b), assigned to the Q (0, 0) and Q (1, 0) bands, respectively, exactly matching the spectral shape and wavelength of PtCP in THF solution. Loading concentrations of PtCP in the nano-porous glass were calculated from the absorbance of the Q(0, 0) band, the thickness of the glass samples, and the molar absorption coefficient of the Q(0, 0) band (3.7 × 104 M−1 cm−1 in THF).
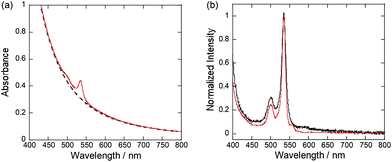 |
| Fig. 2 UV-vis absorption spectra of (a) PtCP-fixed porous glass with a concentration of 77 μM (red line) and blank porous glass (dashed black line). (b) Normalized UV-vis absorption spectra of PtCP obtained by subtracting the blank porous glass from the PtCP-fixed porous glass (solid black line) and PtCP in THF with a concentration of 7.9 μM (solid red line). | |
As control experiments, (1) non-ionic Pt-porphyrin (Pt-octaethylporphyrin) was used instead of PtCP, and (2) the bare porous glass was treated by silanol coupling instead of amino coupling while keeping other conditions the same as before. Both control samples were snow-white and showed no absorption peaks at the Q(1,0) and Q(0,0) bands in their UV-vis absorption spectra as shown in Fig. S1 in the ESI.† These facts clearly show that PtCP was fixed by forming ionic bonds between carboxyl groups of PtCP and amino groups on the surface of the glass.
Phosphorescence spectrum
Upon excitation with 532 nm near the Q(0,0) band, PtCP-fixed porous glass showed vivid deep red emission under an Ar atmosphere. The spectrum has a peak at around 649 nm and a shoulder at 713 nm (Fig. 3). Despite changing the loading concentration of PtCP from 75 μM to 310 μM, 5.1 mM, the spectral shape remained the same with a slightly red-shifted peak wavelength (2 nm) from that of PtCP in THF solution. It is worth noting that the spectra have no dimer emission peaks (770 nm).25 From the good agreement in the spectral shape of the absorption and phosphorescence spectra between the PtCP-fixed porous glass samples and the solution, in addition to the absence of the dimer emission peaks for the glass sample, it can be concluded that most of the PtCP was molecularly dispersed on the surface of the nano-pore of the glass without forming a considerable amount of aggregate. As PtCP cannot dissolve more than 300 μM in THF solution, this material therefore contains a higher PtCP-loading concentration while being free of aggregation. Meanwhile, the shoulder at around 720 nm of the PtCP-fixed glass with 5.1 mM concentration was slightly higher than that of glass samples with lower concentration, indicating that there may be a few dimer-like components.
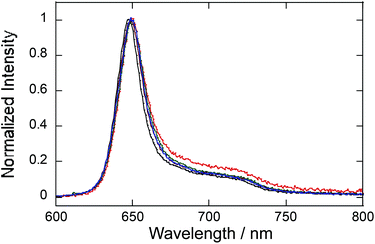 |
| Fig. 3 Phosphorescence emission spectra of PtCP fixed in nano-porous glass with different loading concentrations of 75 μM (blue), 310 μM (green), and 5.2 mM (red) together with PtCP solution in THF (75 μM, black). All samples were deoxygenated by Ar-bubbling. | |
Phosphorescence quantum yield
The phosphorescence quantum yield ΦP of the PtCP-fixed glass under an Ar atmosphere was found to be around 0.25 at a loading concentration of 75 μM, though it fluctuated somewhat from sample to sample (Fig. 4). Interestingly, the observed ΦP of the PtCP-fixed samples were much higher than those in the same concentration of THF solution (0.16 ± 0.018, shown with a triangle in Fig. 4). The ΦP of the PtCP-fixed glass remained constant or gradually decreased with an increase in loading concentration. However, even at the higher loading concentration of 0.64 mM, the value was higher than that of the solution at 75 μM. Meanwhile, the ΦP at concentrations of 4.4 and 5.1 mM are reduced to about 0.1 probably due to self-quenching.26 In contrast to the high ΦP under an Ar atmosphere, the phosphorescence of the glass samples was perfectly quenched (ΦP < 0.005) in the presence of oxygen under the regular atmospheric conditions.27 This suggests that oxygen can diffuse in porous glass through the continuous nano-pores.
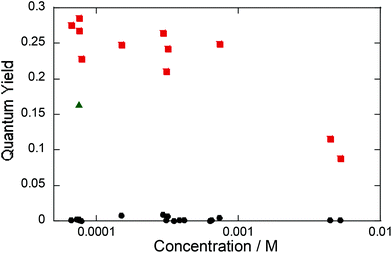 |
| Fig. 4 Phosphorescence quantum yield of PtCP-fixed glass as a function of PtCP concentration in air (black circle) and under an Ar atmosphere (red square). The green triangle shows that of a PtCP solution in THF with a concentration of 75 μM under an Ar atmosphere. | |
Phosphorescence lifetime
The phosphorescence of PtCP-fixed glass with 75 μM concentration exhibits a single exponential decay (τ = 95 μs, Fig. 5) under Ar conditions. The lifetime was unchanged after increasing the excitation intensity up to five times (data not shown). On the other hand, the decays became multi-exponential though the increase of the PtCP loading concentration and were analysed with a double-exponential function (Fig. 5 and Table 1). At a loading concentration of 310 μM, a fast component with a lifetime of 25 μs appeared while the lifetime of the slow component remained unchanged. The relative amplitude of the fast component was small (12%) and the shape of the decay was similar to that of 75 μM. At 5.1 mM, lifetimes of both the fast and slow components were shorter than that of 75 μM and the relative amplitude of the fast component increased (28%). We also measured the lifetime in the presence of oxygen with several concentrations (0.4–21% in volume for the 75 μM sample). The lifetime decreased with an increase in concentration (Fig. S2 in the ESI†).
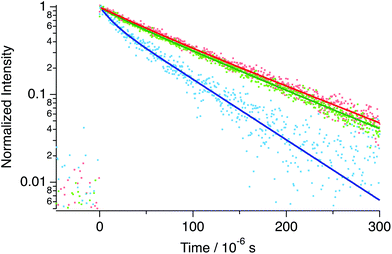 |
| Fig. 5 Phosphorescence decay of PtCP-fixed glass with different loading concentrations of PtCP: 75 μM (red), 310 μM (green), and 5.1 mM (blue) measured under an Ar-atmosphere with their curve fit (solid lines of the corresponding colors) to single- (75 μM) and double-exponential (310 μM and 5.1 mM) functions. | |
Table 1 Quantum yield, lifetimes, and rate constants of phosphorescence of the PtCP-fixed nano-porous glass and the solution, and the average distance between PtCP molecules
Sample |
Φ
P
|
τ
1/μs (A1) |
τ
2/μs (A2) |
〈τ〉/μs |
k
r/103 s−1 |
k
nr/103 s−1 |
d/nm |
Φ
P is the phosphorescence quantum yield. τi and Ai are the lifetime and relative amplitude (i = 1, 2) of PtCP phosphorescence, respectively. 〈τ〉 is the average lifetime for the double-component decays. kr and knr are radiative and nonradiative rate constants of PtCP triplet excited states (the average lifetime was used to calculate the double-component decays). d is the average distance between PtCP molecules on the surface for the PtCP-fixed glass calculated from the surface area and volume for the PtCP solution calculated from the concentration. |
Porous glass |
75 μM |
0.29 |
95 ± 1 |
— |
— |
3.1 |
7.5 |
27 |
310 μM |
0.21 |
96 ± 2 (0.88) |
25 ± 1 (0.12) |
94 ± 2 |
2.2 |
8.4 |
16 |
5.1 mM |
0.08 |
63 ± 1 (0.72) |
11 ± 1 (0.28) |
59 ± 2 |
1.4 |
15 |
2.9 |
THF (under Ar atmosphere) |
75 μM |
0.16 |
48 ± 1 (0.74) |
25 ± 1 (0.26) |
45 ± 1 |
3.6 |
19 |
28 |
Interestingly, these lifetimes were longer than those of the solution. At the same PtCP concentration of 75 μM, the PtCP-fixed glass had a phosphorescence lifetime twice that of the THF solution (48 μs and 25 μs for the Ar-bubbled sample and 48 ± 1 μs for the freeze–pump–thaw degassed sample, Fig. 6).
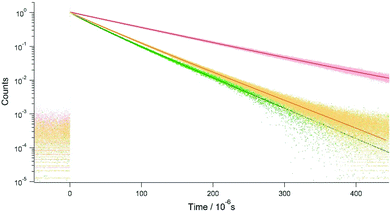 |
| Fig. 6 Phosphorescence decays of PtCP-fixed glass (red) and PtCP solutions in THF deoxygenated by Ar bubbling (green) and by freeze–pump–thaw cycles (orange), together with fitting curves (solid lines, see text). The concentration of PtCP was 75 μM for all samples. The excitation energy was 7.3 μJ per pulse. | |
Radiative and nonradiative rate constants (kr and knr, respectively) were estimated from the obtained lifetimes, τ, and ΦP using the following equations: kr = ΦPτ−1 and knr = τ−1 − kr, assuming that the quantum yield of intersystem crossing of PtCP was unity.28 It was found that the knr of PtCP on the surface of the nano-porous glass was less than half of that in solution (THF) while the kr were almost the same (Table 1), suggesting that the nonradiative deactivation pathway was suppressed when the dye molecule was fixed on the solid surface.
Meanwhile, comparison of the PtCP-fixed porous glass samples shows decreasing kr and increasing knr as the PtCP loading concentration increased. We used the average lifetime defined as 〈τ〉 = ∑iτi2Ai/∑iτiAi (where Ai is the relative amplitude)29 for the 310 μM and 5.1 mM samples to obtain kr and knr as their phosphorescence decays were multi-exponential. The use of average lifetime blurs the theoretical basis for the estimation of kr and knr; nevertheless, they indicate the general tendency of acceleration and suppression of radiative and nonradiative decays, respectively, at high loading concentration.
Discussion
To understand the spectroscopic properties of PtCP-fixed on the surface of nano-porous glass, we consider the intermolecular distance of two neighboring PtCP molecules, d, from the loading concentration and the surface area of the nano-porous glass (Table 1). For the solution, d was calculated from the inverse cubic root of the number density of molecules. The d for the 75 μM porous glass (27 nm) was the same as that of the solution with the same concentration (28 nm), suggesting that each PtCP molecule on the glass surface has enough space between neighbors to be dispersed on a molecular level as the size of each molecule is around 2 nm (Fig. 1a). This supports the observation that the absorption and phosphorescence spectra are identical to those of the solution and PtCP is fixed while maintaining molecular dispersion. Moreover, the smaller knr obtained from the higher ΦP and the longer τ – compared to the solution – indicates a suppressed deactivation pathway on the glass surface. The binding of the molecule to the surface by ionic interaction could be a possible cause of suppression from the restricted freedom of motion on the surface.
The increase of loading concentration reduced d to 16 nm for 310 μM and 2.9 nm for 5.1 mM. At 310 μM, each molecule has significant intermolecular distance relative to its size, while at 5.1 mM they are comparable, thus allowing the neighboring molecules to contact and form aggregates. This scenario agrees with the observation that ΦP and τ of the 310 μM sample were almost identical to those of the 75 μM sample while decreasing ΦP and decreasing τ were observed for the 5.1 mM sample. The decreasing lifetime implies the existence of aggregation,27 which can enhance knr through mechanisms such as intermolecular vibration.
Conclusions
Through the use of ionic bonds, we successfully fabricated PtCP fixed nano-porous glass in which PtCP is molecularly dispersed on the surface of a 3D-network of nano-pores. It is worth noting that the phosphorescence quantum yield and loading concentration are much higher than the limits of PtCP in solution. The molecular dispersion of PtCP at 75 and 310 μM loading concentration was demonstrated by monomeric absorption and phosphorescence spectra and supported by the estimated intermolecular distance on the surface. This is consistent with the constant and high quantum yield (ΦP ∼ 25%) at these loading concentrations. The phosphorescence lifetime measurements clarified that PtCP bonded on glass surface has a much longer lifetime than that in inert solvent, facilitating the high ΦP by suppressing nonradiative deactivation pathways likely a result of the limited freedom of molecular motion from bonding to the nano-porous surface.
At high loading levels of the order of 5 mM, it was found that ΦP dropped with the lifetime, since the loading concentration is nearly saturated as the intermolecular distance approaches the size of the molecule. The molecular dispersion on the surface was considered to be disrupted by the high density of molecules.
The present results reveal that PtCP fixed on a nano-pore surface has unique properties differing from those in solution, and shows superior performance as a phosphorescent material for device applications. The through-hole network of nano-pores allows the flow or storage of gases, liquids and solids, which can be applied to oxygen sensors, wavelength-conversion devices based on the TTA-UC mechanism, and other devices. An application study for TTA-UC devices is currently in progress in our lab.
Conflicts of interest
There are no conflicts to declare.
Acknowledgements
This work was supported by JSPS KAKENHI Grant Numbers JP26107004 and JP17K05852 in Scientific Research on Innovative Areas “Photosynergetics” and in Scientific Research (C), respectively. We thank Dr Takeyuki Uchida of Innovation-Boosting Equipment Common (IBEC), AIST, for the SEM observation. We also thank Ms Yuuko Matsumoto of IFMRI for the fabrication of nano-porous glass and PtCP-fixed glass. Furthermore, we thank Dr Hiroaki Sakurai and Ms Masako Iwamatsu for the gas chromatography measurements.
Notes and references
- D. B. Papkovsky, J. Olah, I. V. Troyanovsky, N. A. Sadovsky, V. D. Rumyantseva, A. F. Mironov, A. I. Yaropolov and A. P. Savitsky, Biosens. Bioelectron., 1992, 7, 199 CrossRef.
- Y. Amao, K. Asai, T. Miyashita and I. Okura, Chem. Lett., 1999, 10, 1031 CrossRef.
- B. Zelelow, G. E. Khalil, G. Phelan, B. Carlson, M. Gouterman, J. B. Callis and L. R. Dalton, Sens. Actuators, B, 2003, 96, 304 CrossRef CAS.
- C.-J. Jin and J.-W. Park, Sens. Actuators, B, 2017, 253, 934 CrossRef.
- C.-S. Chu and T.-H. Lin, Sens. Actuators, B, 2014, 202, 508 CrossRef CAS.
- S. Lamansky, R. C. Kwong, M. Nugent, P. I. Djurovich and M. E. Thompson, Org. Electron., 2001, 2, 53 CrossRef CAS.
- E. M. Gross, N. R. Armstrong and R. M. Wightman, J. Electrochem. Soc., 2002, 149(5), E137 CrossRef CAS.
- T. Tsuboi, Y. Wasai and N. Nabatova-Gabain, Thin Solid Films, 2006, 496, 674 CrossRef CAS.
- M. Janghouri, J. Electron. Mater., 2017, 46, 5635 CrossRef CAS.
- M. Janghouri and M. Adinech, J. Photochem. Photobiol., A, 2017, 341, 31 CrossRef CAS.
- M. R. Jafari and B. Bahrami, Appl. Phys. A, 2015, 119, 1491 CrossRef CAS.
- A. Monguzzi, R. Tubino, S. Hoseinkhani, M. Campione and F. Meinardi, Phys. Chem. Chem. Phys., 2012, 14, 4322 RSC.
- N. Yanai and N. Kimizuka, Chem. Commun., 2016, 52, 5354 RSC.
- K. Kamada, Y. Sakagami, T. Mizokuro, Y. Fujiwara, K. Kobayashi, K. Narushima, S. Hirata and M. Vacha, Mater. Horiz., 2017, 4, 83 RSC.
- Q. Dou, L. Jiang, D. Kai, C. Owh and X. J. Loh, Drug Discovery Today, 2017, 22, 1400 CrossRef CAS PubMed.
- Y. Bai, J.-H. Olivier, H. Yoo, N. F. Polizzi, J. Park, J. Rawson and M. J. Therien, J. Am. Chem. Soc., 2017, 139, 16946 CrossRef CAS PubMed.
- V. Gray, K. Börjesson, D. Dzebo, M. Abrahamsson, B. Albinsson and K. M. Poulsen, J. Phys. Chem. C, 2016, 120, 19018 CAS.
- S. Takagi, T. Shimada, M. Eguchi, T. Yui, H. Yoshida, D. A. Tryk and H. Inoue, Langmuir, 2002, 18, 2265 CrossRef CAS.
- S. Takagi, M. Eguchi, D. A. Tryk and H. Inoue, J. Photochem. Photobiol., C, 2006, 7, 104 CrossRef CAS.
- T. Tanaka, T. Yazawa, K. Eguchi, H. Nagasawa, N. Matsuda and T. Einishi, J. Non-Cryst. Solids, 1984, 65, 301 CrossRef.
- T. Jin, A. H. Ali and T. Yazawa, Chem. Commun., 2001, 99 RSC.
-
W. D. Kingery, H. K. Bowen and D. R. Uhlmann, Introduction to Ceramics, Wiley, New York, 2nd edn, 1976, p. 425 Search PubMed.
- H. Giesche, Part. Part. Syst. Charact., 2006, 23, 1 CrossRef.
- K. Suzuki, A. Kobayashi, S. Kaneko, K. Takehira, T. Yoshihara, H. Ishida, Y. Shiina, S. Oishi and S. Tobita, Phys. Chem. Chem. Phys., 2009, 11, 9850 RSC.
- H. Goudarzi and P. E. Keivanidis, J. Phys. Chem. C, 2014, 118, 14256 CAS.
- A. K. Bansal, W. Holzer, A. Penzkofer and T. Tsuboi, Chem. Phys., 2006, 330, 118 CrossRef CAS.
-
J. R. Lakowicz, Principles of Fluorescence Spectroscopy, Springer, New York, 3rd edn, 2006, p. 317 Search PubMed.
- The quantum yield of intersystem crossing of Pt-octaethylporphyrin (i.e., replaced carboxylic acid with ethyl group in PtCP) was reported to be 0.9 in J. Kavandi, J. Callis, M. Gouterman, G. Khalil, D. Wright and E. Green, Rev. Sci. Instrum., 1990, 61, 3340 CrossRef.
-
J. R. Lakowicz, Principles of Fluorescence Spectroscopy, Springer, New York, 3rd edn, 2006, p. 142 Search PubMed.
Footnote |
† Electronic supplementary information (ESI) available. See DOI: 10.1039/c7pp00449d |
|
This journal is © The Royal Society of Chemistry and Owner Societies 2018 |
Click here to see how this site uses Cookies. View our privacy policy here.