Protected 2′-deoxyribonucleoside triphosphate building blocks for the photocaging of epigenetic 5-(hydroxymethyl)cytosine in DNA†
Received
11th May 2018
, Accepted 8th June 2018
First published on 8th June 2018
Abstract
2′-Deoxyribonucleoside triphosphates (dNTPs) containing 5-(hydroxymethyl)cytosine (5hmC) protected with photocleavable groups (2-nitrobenzyl or 6-nitropiperonyl) were prepared and studied as substrates for the enzymatic synthesis of oligonucleotides and DNA containing a photocaged epigenetic 5hmC base. DNA probes containing photocaged or free 5hmC in the recognition sequence of restriction endonucleases were prepared and used for the study of the photorelease of caged DNA by UV or visible light at different wavelengths. The nitrobenzyl-protected dNTP was a slightly better substrate for DNA polymerases in primer extension or PCR, whereas the nitropiperonyl-protected nucleotide underwent slightly faster photorelease at 400 nm. However, both photocaged building blocks can be used in polymerase synthesis and the photorelease of 5hmC in DNA.
Introduction
5-(Hydroxymethyl)cytosine (5hmC) is an epigenetic DNA base1 which is not only an intermediate in active DNA demethylation2 but also an epigenetic signal regulating gene expression.3,4 Several protected 5hmC 2′-deoxyribonucleoside building blocks for the phosphoramidite synthesis of modified oligonucleotides (ONs) have been reported,5 as well as the synthesis and use of the corresponding unprotected 5hmC 2′-deoxyribonucleoside triphosphate (dChmTP) in the polymerase synthesis of modified DNA.6 For a deeper study of the role of 5hmC in the regulation of transcription,3,4 genomic stability and active demethylation,2 it might be advantageous to have a masked/caged 5hmC, which can be released in DNA on demand.
Photocleavable protecting groups7 play an important role in chemical biology. The photocaging of biomolecules7,8 and cleavage of the photolabile protective groups by light is often used for triggering or switching biological processes. In nucleic acids,9 photocaging is mostly introduced at nucleobases10 to prevent base-pairing and hybridization or at the sugar11 or phosphodiester backbone12 to prevent hydrolysis or interactions with other biomolecules. Photocaging in the major-groove of DNA has been studied less frequently.13,14 Nitrobenzyl- (NB), and phenylethyl-protected 5-(hydroxymethyl)pyrimidine or 7-hydroxymethyl-7-deazapurine-2′-deoxyribonucleoside triphosphates (dNTPs) were used as reversible terminators of primer extension in sequencing.14 We have recently reported the use of NB15 or 6-nitropiperonyl (NP)16 caged 5-hydroxymethyluracil dNTPs for the polymerase synthesis of photocaged DNA that can release 5hmU upon irradiation by UV or visible light (up to 425 nm for NP-protection). Here, we report on the photocaging of dNTPs derived from epigenetic 5hmC, their polymerase incorporation to DNA and photorelease.
Results and discussion
Synthesis
Photocaged 2′-deoxycytidines were prepared from previously reported modified 2′-deoxyuridines 115,16 (Scheme 1). The silyl-protected deoxyuridines 1a and 1b were converted to the corresponding deoxycytidines 2a and 2b in two steps consisting of an activation of the oxo group through reaction with 2,4,6-triisopropyl-benzenesulfonyl chloride and DMAP, followed by nucleophilic substitution using ammonia. Protective groups were removed using Et3N·3HF in THF to yield the desired caged nucleosides dCNB and dCNP in moderate yields of 41 and 39%, respectively. Triphosphorylation reactions were carried out under standard conditions.17 The nucleosides were treated with POCl3 in trimethyl phosphate at 0 °C followed by an addition of pyrophosphate and tributyl amine in DMF and, finally, treatment with triethylammonium bicarbonate (TEAB). The targets dCNBTP and dCNPTP were obtained in moderate yields after isolation with HPLC.
 |
| Scheme 1 Synthesis of photocaged 2′-deoxycytosine triphosphates (i) 2,4,6,-triisopropylbenzenesulfonyl chloride, DMAP, CH3CN or DCM, (ii) NH3 (g) or NH4OH, (iii) Et3N·3HF, THF, (iv) 1. POCl3, PO(OMe)3, 2. (NHBu3)2H2P2O7, 3. TEAB. | |
Fig. 1 shows the absorption spectra of the dCNBTP and dCNPTP in water and calculated molar absorption coefficients at the absorption maxima (for more detailed analysis of absorption spectra, see Fig. S1–S4 in the ESI†). While the NB-caged nucleotide dCNBTP exerted an absorption maximum at 272 nm and showed a low absorption above 300 nm, the nitropiperonyl nucleotide dCNPTP exerted another maximum at 359 nm and still some non-negligible absorbance at 400 nm indicating its potential for photorelease with visible light.
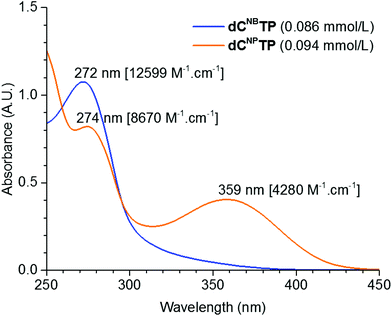 |
| Fig. 1 Absorption spectra of dCNBTP and dCNPTP. | |
Incorporation of caged nucleotides into DNA
Caged triphosphates dCNBTP and dCNPTP as well as the uncaged dChmTP were tested as substrates for DNA polymerases.18 The primer extension (PEX) reaction was studied using a 19-mer template (for the sequences of ONs, see Table S2 in the ESI†) encoding for the incorporation of one modified dCR followed by three guanines. Fig. 2a shows the successful incorporation of all modified dCR nucleotides using KOD XL (for successful PEX using Vent (exo-) polymerase, see Fig. S5 in the ESI†). The identity of the extended modified oligonucleotide (ON) products was confirmed by MALDI-TOF analysis showing masses corresponding to Chm-containing ONs (Fig. S8 and S9 in the ESI†) since the laser irradiation used in MALDI cleaves the photocaging groups. Then we conducted a simple kinetic study of single nucleotide extension (Fig. S10 and S11 in the ESI†), which indicated that the incorporation of modified dCRTPs was only slightly slower compared to that of natural dCTP. The PEX reaction using a longer 31-mer template encoding for the incorporation of 4 dCR modifications (Fig. 2b) also proceeded successfully with dChmTP, dCNBTP or dCNPTP giving clean, fully extended products.
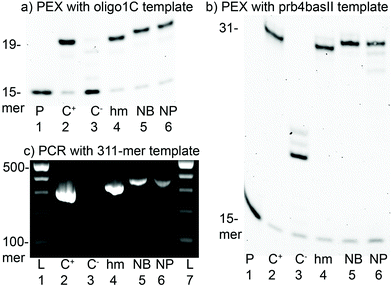 |
| Fig. 2 Denaturing PAGE analysis of the KOD XL DNA polymerase synthesis of modified DNA. (a) PEX with oligo1C 19-mer template and (b) PEX with prb4basII 31-mer template: lane 1, P: primer; lane 2, C+: product of PEX with natural dNTPs; lane 3, C−: products of PEX in the absence of dCTP; lane 4, hm: product of PEX with dChmTP and three natural dNTPs; lane 5, NB: product of PEX with dCNBTP and three natural dNTPs; lane 6, NP: product of PEX with dCNPTP and three natural dNTPs. (C) Agarose gel analysis of PCR with the 311-mer template: lanes 1 and 7, L: 100 bp ladder; lane 2, C+: product of PCR with natural dNTPs; lane 3, C−: product of PCR in the absence of dCTP; lane 4, hm: product of PCR with dChmTP and three natural dNTPs; lane 5, NB: product of PCR with dCNBTP and three natural dNTPs; lane 6, NP: product of PCR with dCNPTP and three natural dNTPs. | |
Finally, we tested dChmTP, dCNBTP and dCNPTP as substrates in PCR amplifications using 98-mer (Fig. S21†) and 311-mer templates. The PCR reactions with dChmTP or dCNBTP proceeded very well giving significant amounts of the modified amplicons (Fig. 2c). The use of bulkier dCNPTP gave a somewhat weaker band of the full-length product, but the amplification still worked out. These results indicate that the photocaged 5hmC triphosphates (dCNBTP and dCNPTP) are comparable or better substrates for the PCR reaction, than analogous photocaged 5-(hydroxymethyl)uracil dNTPs reported previously (although the templates were different).15,16
Photochemical release of the caged DNA
In order to study the photorelease of the caged DNA, we used the previously described approach utilizing the cleavage of modified DNA by restriction endonucleases (REs) for monitoring the photochemical deprotection of DNA.15,16 We have repeatedly shown19,20 that modified DNA containing bulky groups in the major groove is not cleaved by RE, whereas non-modified DNA or DNA containing small modifications at T or A is fully cleaved by some REs. However, DNA duplexes containing modified C20 or G21 bases are typically not recognized and cleaved by most REs. Therefore, the first goal was to identify some REs that would recognize and cleave DNA containing 5hmC and not cleave the photocaged DNA. We prepared the corresponding modified DNA duplexes containing 5hmC or the photocaged dCNB by PEX. The sequences always contained restriction sites for one of the tested 6 different REs (AflII, EcoRI, KpnI, PvuII, RsaI and HF-ScaI). Then we studied the cleavage of the modified DNA by REs (see Fig. S16 in the ESI†). Luckily, we found two enzymes (KpnI and RsaI) that fully cleaved hmC-modified DNA and did not cleave the photocaged DNA (Fig. S16†). Subsequently, we prepared 30-mer PEX products containing a dCR modification in the recognition site for KpnI or RsaI. We tested the cleavage of the DNA by RE before and after irradiation using different LED diodes (Scheme 2). Analogous to our previous work on photocaged 5hmU DNA,16 we tested the photorelease of each caging group from 5hmC with UV or visible light at three different wavelengths (355, 400 and 425 nm).
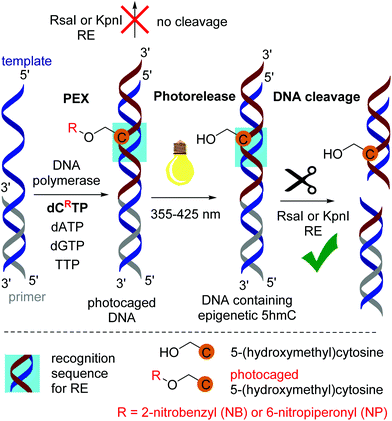 |
| Scheme 2 PEX synthesis of photocaged DNA, photorelease and cleavage of DNA by the restriction enzyme. | |
Fig. 3 shows the results of the photochemical uncaging reactions of caged DNA at 355 (a) and 400 nm (b), whereas Table 1 (and Fig. S18–S20 in the ESI†) gives the complete data at all tested wavelengths. ImageJ software was used to quantify the ratios of caged to uncaged DNA, which correspond to the reaction conversions (Tables 1 and 2). Because of a different optical power of the different LEDs, the reaction times differed. In all cases, we tested at least 3 different irradiation times to reach complete (or almost complete) conversion whenever possible. The cleavage of NB and NP groups proceeded with comparable efficiencies at lower wavelengths (355 nm) reaching good conversions (60–70%) in reasonably short reaction times (10–120 min). Only at higher wavelengths (400 and 425 nm) was the nitropiperonyl group removed significantly faster. When using visible light (at 425 nm), the removal of the photocaging groups never reached full conversion even after 300 min of irradiation (maximum conversion for the NP group was ca. 65%). On the other hand, at 400 nm, both groups were uncaged efficiently reaching high or almost quantitative conversions (80–95%). Apparently, both NB and NP photocaging groups at 5hmC can be cleaved with UV or visible light but the cleavage at 425 nm is less efficient compared to that of NP-protected 5hmU.16
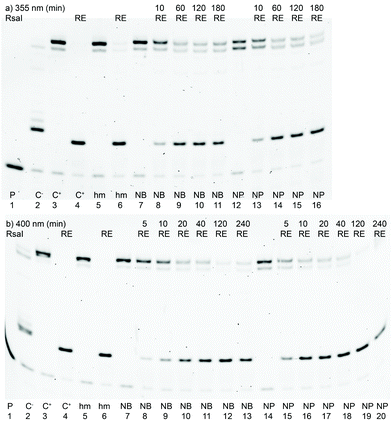 |
| Fig. 3 Denaturing PAGE analysis of PEX with RsC 30-mer template followed by uncaging with a UV LED (a) 355 nm (0.8–1.2 mW), (b) 400 nm (21–29 mW): lane 1, P: primer; lane 2, C−: product of PEX in the absence of dCTP; lane 3, C+: product of PEX with natural dNTPs; lane 4, C+: products of PEX with natural dNTPs followed by reaction with RE; lane 5, hm: product of PEX with dChmTP and three natural dNTPs; lane 6, hm: product of PEX with dChmTP and three natural dNTPs followed by reaction with RE; lane 7: products of PEX with dCNBTP; lanes 8–11, or 8–13: product of PEX with dCNBTP and three natural dNTPs after irradiation with a UV lamp (irradiation time in minutes) followed by reaction with RE; lane 12 or 14: products of PEX with or dCNPTP and three natural dNTPs; lanes 13–16 or 15–20: products of PEX with dCNPTP and three natural dNTPs after irradiation with a UV lamp (irradiation time in minutes) followed by reaction with RE. | |
Table 1 KpC template – DNA uncaging conversion quantification evaluated from gels using ImageJ software
Caging group |
Λ (nm) |
Optical powera (mW) |
Time 1 (min) |
Conv. (%) |
Time 2 (min) |
Conv. (%) |
Time 3 (min) |
Conv. (%) |
Optical power of the LED diodes supplied by the manufacturer.
|
NB |
355 |
0.8–1.2 |
10 |
16 |
60 |
67 |
120 |
66 |
NP |
355 |
0.8–1.2 |
10 |
24 |
60 |
62 |
120 |
71 |
NB |
400 |
21–29 |
5 |
9 |
20 |
43 |
120 |
81 |
NP |
400 |
21–29 |
5 |
27 |
20 |
65 |
120 |
78 |
NB |
425 |
10–16 |
30 |
2 |
120 |
11 |
180 |
28 |
NP |
425 |
10–16 |
30 |
11 |
120 |
44 |
180 |
49 |
Table 2 RsC template – DNA uncaging conversion quantification evaluated from gels using ImageJ software
Caging group |
Λ (nm) |
Optical powera (mW) |
Time 1 (min) |
Conv. (%) |
Time 2 (min) |
Conv. (%) |
Time 3 (min) |
Conv. (%) |
Optical power of the LED diodes supplied by the manufacturer.
|
NB |
355 |
0.8–1.2 |
10 |
19 |
60 |
57 |
120 |
61 |
NP |
355 |
0.8–1.2 |
10 |
19 |
60 |
60 |
120 |
61 |
NB |
400 |
21–29 |
5 |
10 |
20 |
55 |
120 |
85 |
NP |
400 |
21–29 |
5 |
36 |
20 |
71 |
120 |
95 |
NB |
425 |
10–16 |
60 |
15 |
180 |
27 |
300 |
42 |
NP |
425 |
10–16 |
60 |
31 |
180 |
65 |
300 |
65 |
Conclusions
We prepared two types of photocaged derivatives of 2′-deoxy-5-(hydroxymethyl)cytosine dNTPs protected by 2-nitrobenzyl or 6-nitropiperonyl groups, and tested their enzymatic incorporations into DNA and photochemical deprotections. The NB- and NP-caged dNTPs (dCNBTP and dCNPTP) were good substrates for DNA polymerases and worked in PEX reactions, as well as in PCR amplifications. The liberation of the photocaged DNA was tested using monitoring by cleavage of DNA with REs, which tolerate hmC. We showed that both NB- and NP-caged DNA can be efficiently deprotected by UV irradiation, whereas the nitropiperonyl group is more suitable for uncaging with visible light at 400 or 425 nm. Although the visible-light photorelease of 5hmC from NB-caged DNA is slightly less efficient than the uncaging of the corresponding NP-caged 5hmU-containing DNA,16 the NB-protection of 5hmC still has a good potential for photocaging of DNA containing this important epigenetic base and for some biological applications including studies of active demethylation and regulation of transcription in cellulo or in vivo.
Experimental
The full experimental part is given in the ESI.† Selected typical experiments are given below.
PEX – multiple modifications
The reaction mixture (20 μL) contained KOD XL DNA polymerase (2.5 U μL−1, 0.02 μL), a mixture of dATP, dGTP and dTTP (1 mM, 1.5 μL), dTTP (1 mM, 3 μL) or modified dCRTP (1 mM, 1.5 μL or 3 μL for dCNBTP and dCNPTP), 6-FAM labelled primer 248sh (3 μM, 1 μL), 31-mer template prb4basII (3 μM, 1.5 μL) and 10× buffer for KOD XL DNA polymerase (2 μL) supplied by the manufacturer. Reaction mixtures were incubated for 30 min at 60 °C in a thermal cycler. After reaction samples were denatured by the addition of stop solution (20 μL, 80% [v/v] formamide, 20 mM EDTA, 0.025%, [w/v] bromophenol blue, 0.025% [w/v] xylene cyanol, PCR water) followed by heating for 5 min at 95 °C. Reaction mixtures were separated using 12.5% denaturing PAGE.
Uncaging of DNA
KpC template.
The reaction mixture (20 μL) contained KOD XL DNA polymerase (2.5 U μL−1, 0.02 μL), mixture of dATP, dGTP and dTTP (1 mM, 0.5 μL), dCTP or modified dCRTP (1 mM, 0.5 μL or 1 μL for dCNBTP and dCNPTP), 6-FAM labelled primer 248sh (3 μM, 1 μL), 30-mer template KpC (3 μM, 1.5 μL) and 10× buffer for KOD XL DNA polymerase (2 μL) supplied by the manufacturer.
RsC template.
The reaction mixture (20 μL) contained KOD XL DNA polymerase (2.5 U μL−1, 0.02 μL), mixture of dATP, dGTP and dTTP (1 mM, 0.2 μL), dCTP or modified dCRTP (1 mM, 0.2 μL or 0.25 μL for dCNBTP and dCNPTP), 6-FAM labelled primer 248sh (3 μM, 1 μL), 30-mer template RsC (3 μM, 1.5 μL) and 10× buffer for KOD XL DNA polymerase (2 μL) supplied by the manufacturer.
Reaction mixtures were incubated for 30 min at 60 °C in a thermal cycler.
After reaction the samples were either:
(a) Denatured by the addition of stop solution (23 μL, 80% [v/v] formamide, 20 mM EDTA, 0.025%, [w/v] bromophenol blue, 0.025% [w/v] xylene cyanol, PCR water) and water (3 μL) and heating at 95 °C for 5 min.
(b) Incubated with RE (1.6 μL) in CutSmart or 1.1 NEBuffer (2.2 μL) and denatured by the addition of stop solution (23 μL) and heating at 95 °C for 5 min.
(c) Irradiated with a UV LED followed by incubation with RE (1.6 μL) in CutSmart or 1.1 NEBuffer (2.2 μL) and denaturation by the addition of stop solution (23 μL) and heating at 95 °C for 5 min.
Reaction mixtures were separated using 12.5% denaturing PAGE.
Conflicts of interest
There are no conflicts to declare.
Acknowledgements
This work was supported by the Czech Academy of Sciences (Praemium Academiae award to M. H.) and by the Czech Science Foundation (17-03419S to S. B., Z. V. and M. H).
References
-
(a) S. Kriaucionis and N. Heintz, Science, 2009, 324, 929–930 CrossRef PubMed;
(b) E. A. Raiber, R. Hardisty, P. van Delft and S. Balasubramanian, Nat. Rev. Chem., 2017, 1, 0069 CrossRef;
(c) T. Carell, M. Q. Kurz, M. Müller, M. Rossa and F. Spada, Angew. Chem., Int. Ed., 2018, 57, 4296–4212 CrossRef PubMed;
(d) K. Chen, B. S. Zhao and C. He, Cell Chem. Biol., 2016, 23, 74–85 CrossRef PubMed;
(e) Y. Fu and C. He, Curr. Opin. Chem. Biol., 2012, 16, 516–524 CrossRef PubMed.
-
(a) X. Lu, B. S. Zhao and C. He, Chem. Rev., 2015, 115, 2225–2239 CrossRef PubMed;
(b) M. Wagner, J. Steinbacher, T. F. J. Kraus, S. Michalakis, B. Hackner, T. Pfaffeneder, A. Perera, M. Müller, A. Giese, H. A. Kretzschmar and T. Carell, Angew. Chem., Int. Ed., 2015, 54, 12511–12514 CrossRef PubMed;
(c) T. Pfaffeneder, B. Hackner, M. Truss, M. Münzel, M. Müller, C. A. Deiml, C. Hagemeier and T. Carell, Angew. Chem., Int. Ed., 2011, 50, 7008–7012 CrossRef PubMed;
(d) D. Globisch, M. Münzel, M. Müller, S. Michalakis, M. Wagner, S. Koch, T. Brückl, M. Biel and T. Carell, PLoS One, 2010, 5, e15367 CrossRef PubMed.
-
(a) A. Perera, D. Eisen, M. Wagner, S. K. Laube, A. F. Künzel, S. Koch, J. Steinbacher, E. Schulze, V. Splith, N. Mittermeier, M. Muller, M. Biel, T. Carell and S. Michalakis, Cell Rep., 2015, 11, 283–294 CrossRef PubMed;
(b) C. M. Greco, P. Kunderfranco, M. Rubino, V. Larcher, P. Carullo, A. Anselmo, K. Kurz, T. Carell, A. Angius, M. V. G. Latronico, R. Papait and G. Condorelli, Nat. Commun., 2016, 7, 12418 CrossRef PubMed;
(c) C. You, D. Li, X. Dai and Y. Wang, Sci. Rep., 2014, 4, 7052 CrossRef PubMed;
(d) L. Wang, Y. Zhou, L. Xu, R. Xiao, X. Lu, L. Cheng, J. Chong, H. Li, C. He, X.-D. Fu and D. Wang, Nature, 2015, 523, 621–625 CrossRef PubMed;
(e) M. W. Kellinger, C.-X. Song, J. Chong, X.-Y. Lu, C. He and D. Wang, Nat. Struct. Mol. Biol., 2012, 19, 831–833 CrossRef PubMed.
- M. Janoušková, Z. Vaníková, F. Nici, S. Boháčová, D. Vítovská, H. Šanderová, M. Hocek and L. Krásný, Chem. Commun., 2017, 53, 13253–13255 RSC.
-
(a) M. Münzel, D. Globisch, C. Trindler and T. Carell, Org. Lett., 2010, 12, 5671–5673 CrossRef PubMed;
(b) M. Münzel, U. Lischke, D. Stathis, T. Pfaffeneder, F. A. Gnerlich, C. A. Deiml, S. C. Koch, K. Karaghiosoff and T. Carell, Chem. – Eur. J., 2011, 17, 13782–13788 CrossRef PubMed;
(c) M. Münzel, D. Globisch and T. Carell, Angew. Chem., Int. Ed., 2011, 50, 6460–6468 CrossRef PubMed;
(d) A. S. Schröder, J. Steinbacher, B. Steigenberger, F. A. Gnerlich, S. Schiesser, T. Pfaffeneder and T. Carell, Angew. Chem., Int. Ed., 2014, 53, 315–318 CrossRef PubMed.
- B. Steigenberger, S. Schiesser, B. Hackner, C. Brandmayr, S. K. Laube, J. Steinbacher, T. Pfaffeneder and T. Carell, Org. Lett., 2013, 15, 366–369 CrossRef PubMed.
-
(a) P. Klan, T. Solomek, C. G. Bochet, A. Blanc, R. Givens, M. Rubina, V. Popik, A. Kostikov and J. Wirz, Chem. Rev., 2013, 113, 119–191 CrossRef PubMed;
(b) T. Šolomek, J. Wirz and P. Klán, Acc. Chem. Res., 2015, 48, 3064–3072 CrossRef PubMed.
- Reviews:
(a) I. Ahmed and L. Fruk, Mol. Biosyst., 2013, 9, 565–570 RSC;
(b) C. Brieke, F. Rohrbach, A. Gottschalk, G. Mayer and A. Heckel, Angew. Chem., Int. Ed., 2012, 51, 8446–8476 CrossRef PubMed;
(c) C. W. Riggsbee and A. Deiters, Trends Biotechnol., 2010, 28, 468–475 CrossRef PubMed;
(d) H.-M. Lee, D. R. Larson and D. S. Lawrence, ACS Chem. Biol., 2009, 4, 409–427 CrossRef PubMed;
(e) A. Deiters, Curr. Opin. Chem. Biol., 2009, 13, 678–686 CrossRef PubMed;
(f) G. C. R. Ellis-Davies, Nat. Methods, 2007, 4, 619–628 CrossRef PubMed;
(g) D. D. Young and A. Deiters, Org. Biomol. Chem., 2007, 5, 999–1005 RSC.
- Reviews:
(a) M. Ikeda and M. Kabumoto, Chem. Lett., 2017, 46, 634–640 CrossRef;
(b) Q. Liu and A. Deiters, Acc. Chem. Res., 2014, 47, 45–55 CrossRef PubMed;
(c) L. M. Ceo and J. T. Koh, ChemBioChem, 2012, 13, 511–513 CrossRef PubMed;
(d) X. Tang, J. Zhang, J. Sun, Y. Wang, J. Wu and L. Zhang, Org. Biomol. Chem., 2013, 11, 7814–7824 RSC;
(e) A recent example: L. Anhauser, F. Muttach and A. Rentmeister, Chem. Commun., 2018, 54, 449–451 RSC.
-
(a) A. Deiters, R. A. Garner, H. Lusic, J. M. Goven, M. Dush, N. M. Nascone-Yoder and J. A. Yoder, J. Am. Chem. Soc., 2010, 132, 15644–15650 CrossRef PubMed;
(b) J. M. Govan, R. Uprety, J. Hemphill, M. O. Lively and A. Deiters, ACS Chem. Biol., 2012, 7, 1247–1256 CrossRef PubMed;
(c) J. Hemphill, J. Govan, R. Uprety, M. Tsang and A. Deiters, J. Am. Chem. Soc., 2014, 136, 7152–7158 CrossRef PubMed;
(d) A. Rodrigues-Correia, X. M. M. Weyel and A. Heckel, Org. Lett., 2013, 15, 5500–5503 CrossRef PubMed;
(e) M. A. H. Fichte, X. M. M. Weyel, S. Junek, F. Schäfer, C. Herbivo, M. Goeldner, A. Specht, J. Wachtveitl and A. Heckel, Angew. Chem., Int. Ed., 2016, 55, 8948–8952 CrossRef PubMed.
- W. A. Velema, A. M. Kietrys and E. T. Kool, J. Am. Chem. Soc., 2018, 140, 3491–3495 CrossRef PubMed.
-
(a) W. T. Monroe, M. M. McQuain, M. S. Chang, J. S. Alexander and F. R. Haselton, J. Biol. Chem., 1999, 274, 20895–20900 CrossRef PubMed;
(b) S. Shah, S. Rangarajan and S. H. Friedman, Angew. Chem., Int. Ed., 2005, 44, 1328–1332 CrossRef PubMed;
(c) A. Teraoka, K. Murakoshi, K. Fukamauchi, A. Z. Suzuki, S. Watanabe and T. Furuta, Chem. Commun., 2014, 50, 664–666 RSC.
-
(a) J. Wu and X. Tang, Bioorg. Med. Chem., 2013, 21, 6205–6211 CrossRef PubMed;
(b) K. Ohno, D. Sugiyama, L. Takeshita, T. Kanamori, Y. Masaki, M. Sekine and K. Seio, Bioorg. Med. Chem., 2017, 25, 6007–6015 CrossRef PubMed.
-
(a) V. A. Litosh, W. D. Wu, B. P. Stupi, J. C. Wang, S. E. Morris, M. N. Hersh and M. L. Metzker, Nucleic Acids Res., 2011, 39, e39 CrossRef PubMed;
(b) B. P. Stupi, H. Li, J. C. Wang, W. D. Wu, S. E. Morris, V. A. Litosh, J. Muniz, M. N. Hersh and M. L. Metzker, Angew. Chem., Int. Ed., 2012, 51, 1724–1727 CrossRef PubMed.
- Z. Vaníková and M. Hocek, Angew. Chem., Int. Ed., 2014, 53, 6734–6737 CrossRef PubMed.
- S. Boháčová, L. Ludvíková, L. Poštová Slavětínská, Z. Vaníková, P. Klán and M. Hocek, Org. Biomol. Chem., 2018, 16, 1527–1535 RSC.
- T. Kovacs and L. Ötvös, Tetrahedron Lett., 1988, 29, 4525–4528 CrossRef.
- For reviews on polymerase incorporation of modified nucleotides, see:
(a) M. Hocek, J. Org. Chem., 2014, 79, 9914–9921 CrossRef PubMed;
(b) A. Hottin and A. Marx, Acc. Chem. Res., 2016, 49, 418–427 CrossRef PubMed;
(c) M. Hollenstein, Molecules, 2012, 17, 13569–13591 CrossRef PubMed;
(d) M. Kuwahara and N. Sugimoto, Molecules, 2010, 15, 5423–5444 CrossRef PubMed.
-
(a) H. Macíčková-Cahová and M. Hocek, Nucleic Acids Res., 2009, 37, 7612–7622 CrossRef PubMed;
(b) P. Kielkowski, H. Macíčková-Cahová, R. Pohl and M. Hocek, Angew. Chem., Int. Ed., 2011, 50, 8727–8730 CrossRef PubMed.
- H. Macíčková-Cahová, R. Pohl and M. Hocek, ChemBioChem, 2011, 12, 431–438 CrossRef PubMed.
- M. Mačková, S. Boháčová, P. Perlíková, L. Poštová Slavětínská and M. Hocek, ChemBioChem, 2015, 16, 2225–2236 CrossRef PubMed.
Footnote |
† Electronic supplementary information (ESI) available. See DOI: 10.1039/c8ob01106k |
|
This journal is © The Royal Society of Chemistry 2018 |
Click here to see how this site uses Cookies. View our privacy policy here.