DOI:
10.1039/C7NR06293A
(Communication)
Nanoscale, 2018,
10, 124-131
Strong magnetic resonances and largely enhanced second-harmonic generation of colloidal MoS2 and ReS2@Au nanoantennas with assembled 2D nanosheets†
Received
23rd August 2017
, Accepted 29th November 2017
First published on 29th November 2017
Abstract
Colloidal disk-like and sphere-like MoS2 nanoantennas are synthesized. They consist of curly and interlaced 2D nanosheets. The resonance peak of the MoS2 nanoantennas can be tuned from 500 to 900 nm by adjusting the size and shape. The strong magnetic and electric resonances of the dielectric antennas are revealed by theoretical calculations with Mie theory. The second harmonic generation (SHG) of the exfoliated nanosheets and the synthesized nanodisks and nanospheres is investigated and compared by scanning the excitation laser wavelength. SHG enhancement of 52 fold is observed for the spherical nanoantennas at 400 nm, which is attributed to the nanoantenna-enhanced two-photon resonance excitation of the D exciton of MoS2 monolayers. Moreover, ReS2@Au plasmon–dielectric hybrid nanoantennas are also synthesized. The SHG of Au nanoparticles is enhanced 8.5 times by the coupling of the two types of nanoantennas. This new class of optical nanoantennas consisting of 2D materials and exhibiting unique linear and nonlinear optical responses will bring promising applications ranging from nonlinear photonics to photochemistry.
1. Introduction
Two-dimensional (2D) transition metal dichalcogenide (TMDC) materials MX2 (M = Mo, W, and Re; X = S, Se, and Te) possess unique optical properties as they evolve from indirect to direct bandgap semiconductors.1–3 They are promising functional materials for nanophotonic,4–6 optoelectronic,7–10 and photovoltaic applications.11,12 The breaking of the crystalline inversion symmetry and strong exciton effects make 2D TMDCs an ideal class of optical nonlinear materials.13–25 The Coulomb interaction for the formation of excitons from electron–hole pairs is significantly enhanced by the strong quantum confinement, large effective masses, and reduced dielectric screening in these 2D systems, which results in multiple well-defined exciton resonances with large exciton binding energies.26–30 Specifically, four excitonic resonances have been identified in MoS2 monolayers in the visible region.31–34 They reflect the behaviors of k-valley transitions and the crystal phase. The resonant two-photon excitation at the lowest excitonic transition in MoSe2 monolayers induces maximal valley coherence and giant enhancement of the second harmonic generation (SHG) owing to the unusual combination of electric dipole and magnetic dipole transitions.35–37
On the other front, optical antennas, including plasmonic and dielectric nanoantennas,38–43 provide an effective tool for interconnecting local electromagnetic fields and propagating radiations and also for strongly enhancing light–matter interaction at the nanoscale. Optical antennas have been successfully used to manipulate light scattering and propagation, improve the fluorescence emission of nearby molecules,44,45 and enhance nonlinear emissions of the constituting nanomaterials.46 All-dielectric optical antennas with appropriate sizes and refractive indexes can support both electric and magnetic resonances in the visible region. They have the advantages for the aforementioned optical applications. For instance, directional forward scattering in the visible region is induced by colloidal Cu2O nanospheres with a moderate refractive index owing to the spectral overlap of their electric and magnetic dipole resonances.47 Molecular fluorescence is enhanced more than 300 times by a Si nanodisk dimer with a high refractive index owing to the strong local field enhancement.48 The linear and nonlinear responses of the excitons of the constituting semiconductors can also be enhanced by the electromagnetic resonances of dielectric antennas.
TMDC monolayers have strong exciton confinement and high refractive indexes.49 They are therefore good candidates for building dielectric nanoantennas. Combining the advantages of 2D nanomaterials and dielectric antennas is highly desired for applications ranging from photochemistry to optical nonlinear nanodevices, but the shape and resonance control of this new class of nanoantennas is challenging.
In this study, we have synthesized disk-like and sphere-like colloidal MoS2 nanoantennas via the self-assembly of 2D nanosheets with resonance wavelengths tuned from 500 nm to 900 nm. The size and shape dependence of the electric and magnetic resonances of the nanoantennas is analyzed by using Mie scattering theory and finite difference time domain (FDTD) simulations. The SHG intensity of the MoS2 nanoantennas is enhanced 52 times in comparison with that of the individual monolayers at the laser wavelength of 800 nm, which is attributed to the resonant two-photon excitation of the D exciton of semiconducting MoS2 and the magnetic dipole resonance of the nanoantennas. Furthermore, ReS2@Au plasmon–dielectric hybrid nanoantennas have also been synthesized. The SHG of Au nanoparticles is enhanced 8.5 times by the coupling of the two types of nanoantennas.
2. Experimental section
2.1 Synthesis of MoS2, ReS2, and ReS2@Au nanoantennas
Sodium molybdate dehydrate (Na2MoO4·2H2O, 99.0%), hydroxylammonium chloride (NH2OH·HCl, 98.5%), L-cysteine (HSCH2(NH2)CO2H, 99.0%), chloroauric acid (HAuCl4·4H2O, 99.9%), methanol (CH3OH, 99.9%) and ethanol (CH3CH2OH, 99.9%) were purchased from Sinopharm Chemical Reagent Co. Ltd (Shanghai, China). Ammonium perrhenate (NH4ReO4, 99.0%) was obtained from Sigma-Aldrich (United States). The mechanically exfoliated monolayer MoS2 nanosheets were obtained from Nanjing XFNANO Materials Tech Co., Ltd, which were dispersed in Li(OH) solutions. All the chemicals were used as received without purification. Ultrapure water with a resistivity of approximately 18.25 MΩ cm was used as the solvent in all the experiments.
0.4–1 ml of 0.1 M Na2MoO4, 2 mL of 0.1 M L-cysteine and 7 mL water were mixed well, then transferred to a Teflon bottle (20 ml in capacity) in a stainless steel autoclave, sealed and stored at 180 °C for 12 h. After the mixture was cooled to room temperature, the solution was centrifuged at 8000 rpm for 5 min followed by the removal of the supernatant. The products, MoS2 nanodisks and nanospheres consisting of 2D nanosheets, are obtained by adjusting the amount of Na2MoO4.
0.4–1 ml of 0.1 M NH4ReO4, 3 mL of 0.1 M L-cysteine, 3 mL of 0.1 M NH2OH·HCl and 3 ml water were mixed well, then transferred to a Teflon bottle (20 ml in capacity) in a stainless steel autoclave, sealed and stored at 200 °C for 12 h. After the mixture was cooled to room temperature, the solution was centrifuged at 8000 rpm for 5 min followed by the removal of the supernatant. The products are ReS2 nanospheres consisting of 2D nanosheets. 5 ml methanol was added into 5 ml of as-prepared MoS2 or ReS2 water solution, and then a certain amount of 5 mM HAuCl4 was injected. The mixture was irradiated by a Xe lamp (300 W) for 3 h, and the products, ReS2@Au, were obtained by centrifugation at 6000 rpm for 5 min.
2.2 Characterization and SHG measurements
The morphology of the MoS2, ReS2, and ReS2@Au nanoantennas was investigated by using a scanning electron microscope (SEM, Hitachi S-4800) and a transmission electron microscope (TEM). The TEM and high resolution TEM (HRTEM) images were obtained with a JEOL 2010 HT TEM and a JEOL 2010 FET TEM at an accelerating voltage of 200 kV, respectively.
The absorption spectra were recorded by using a TU-1810 UV-Vis-NIR spectrophotometer (Purkinje General Instrument Co. Ltd, Beijing, China). The ultrafast laser pulses were generated using a mode-locked Ti/sapphire laser (Mira 900, Coherent); the pulse width is 150 fs and the repetition rate is 76 MHz. In the SHG measurements, the fundamental excitation laser beam is p-polarized with an incidence angle of ∼π/3. The SHG emissions are recorded by spectrometry (Spectrapro 2500i, Acton) with a liquid-nitrogen-cooled CCD (SPEC-10, Princeton).
2.3 Numerical calculations
The extinction spectra of the dielectric nanospheres were calculated using Mie theory; the nanospheres were placed in a uniform medium of water with a refractive index of 1.333. The extinction spectra of the oblate ellipsoids and the local electric fields were calculated using the finite difference time domain (FDTD) method, which was performed by the commercial software FDTD Solutions v8.6 (Lumerical Solutions). The mesh size was set at 2.2 × 2.2 × 2.2 nm3 with the use of the total-field scattered-field source. The absorbing boundary was set to be perfectly matched layers. The dielectric constants of MoS2 and Au were taken from previously reported values.49,50
3. Results and discussion
3.1 Growth of MoS2 nanodisks and nanospheres
The disk-like and sphere-like MoS2 nanostructures with assembled nanosheets are synthesized by adjusting the concentration of MoO42− ions (μMo) and the reaction temperature during the hydrothermal synthesis process.51,52 At a very low MoO42− concentration μMo = 4 mM, the assembly of the nanosheets at the beginning stage leads to the formation of MoS2 nanodisks, which has a low density of nanosheets and a large variation of the lateral size and shape, as shown by the TEM image in Fig. 1a. The average lateral size 2R is approximately 220 nm. The SEM image in the inset in Fig. 1a shows the side view of the nanodisk. The aspect ratio ρ of the nanodisks between the height and lateral size is approximately 0.37.
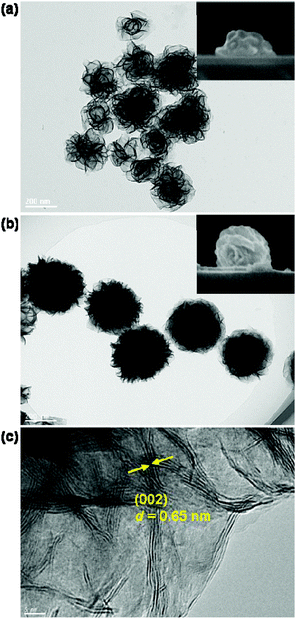 |
| Fig. 1 Nanostructures of the synthesized MoS2 nanodisks and nanospheres consisting of nanosheets. (a) TEM image of the MoS2 nanodisks with average lateral diameters of 2R = 220 nm. Inset: Side view of the SEM image of a nanodisk with aspect ratio ρ ≈ 0.37. (b) TEM image of the MoS2 nanospheres (2R = 310 nm) with a high density of assembled nanosheets. Inset: Side view of the SEM image of a nanosphere with aspect ratio ρ ≈ 0.80. (c) HRTEM image of the MoS2 nanosheets on the edge region of nanospheres with a lattice fringe d-spacing of 0.65 nm. | |
MoS2 nanospheres are obtained when the MoO42− concentration μMo is increased to higher than 10 mM. They have a much higher density of curly and interlaced nanosheets growing in all directions and relatively uniform sizes and shapes in comparison with the MoS2 nanodisks (see Fig. 1b). The top-view TEM image reveals that the average lateral size 2R increases to 310 nm, and the side-view SEM image shows that the aspect ratio increases to 0.80. Both synthesized MoS2 nanodisks and nanospheres can be viewed as oblate ellipsoids.
Fig. 1c presents a HRTEM image in the edge region of a MoS2 nanosphere. It reveals that the nanosheets curl and stretch out towards the edges. The lattice fringes with a d-spacing of 0.65 nm can be assigned to the (002) lattice plane of the hexagonal MoS2. They arise from stacked nanosheets along the c-axis. Compared to MoS2 sub-microspheres reported in the literature,52,53 the MoS2 nanospheres prepared in this study have relatively small sizes and high densities of nanosheets. The small size and high density nanosheets bring the advantage of highly tunable magnetic and electric resonances for the MoS2 nanoantennas in the visible region and largely enhanced linear and nonlinear responses.
3.2 Excitonic absorption and magnetic resonances of MoS2 nanodisks and nanospheres
Fig. 2 presents the extinction spectra of the exfoliated nanosheets and the synthesized MoS2 nanodisks and nanospheres. For the reference sample of mechanically exfoliated monolayers (see Fig. 2c), the extinction is mainly attributed to photon absorption. The absorption strength dramatically decreases with increasing wavelengths and becomes very weak when the wavelength is longer than 630 nm.
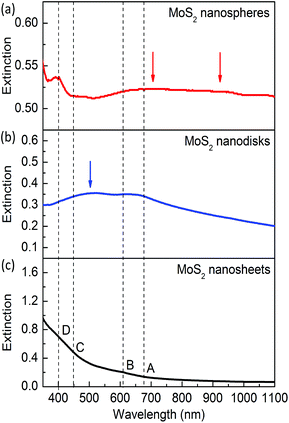 |
| Fig. 2 Typical extinction spectrum of (a) nanospheres, (b) nanodisks, and (c) exfoliated monolayers of MoS2. The resonances of four excitons of MoS2 monolayers, labeled as A, B, C, and D and with dashed lines, are enhanced in the nanodisks and nanospheres. The resonances induced by dielectric nanoantennas are marked by arrows. The nanodisks have a resonance peak around 500 nm, and the nanospheres exhibit broadened resonances in the range 650–1100 nm and two weak peaks around 700 and 900 nm. | |
There are four weak absorption peaks around 670, 610, 450, and 400 nm, which are usually labeled as A, B, C, and D excitons, respectively. The A and B excitons at the band edge arise from the k point of the Brillouin zone. The C and D excitons are attributed to the semiconducting 2H phase. The exfoliated monolayer MoS2 nanosheets used in this study are dispersed in Li(OH) solutions. Li intercalation causes the loss of the semiconducting properties and results in weakened exciton absorption. Pronounced excitonic absorption peaks can be obtained by annealing or treatment in N,N-dimethylformamide solutions.51,54,55
For the synthesized MoS2 nanospheres with an average lateral size 2R ∼ 220 nm, the A and B excitons at the band edge become prominent (see Fig. 2b). The semiconducting C and D excitons are hard to observe for this sample, which might be caused by the very low concentration of Mo in the hydrothermal synthesis. A new peak appears on the extinction spectrum around 500 nm, which is caused by the dipole mode of the resonant scattering of the MoS2 nanoantennas. This resonance mode is similar to that of Cu2O dielectric nanoantennas.47
The synthesized MoS2 nanospheres with 2R ∼ 310 nm exhibit rich resonances including four excitons and multiple modes of dielectric nanoantennas (see Fig. 2a). The resonance around 400 nm becomes prominent and much stronger than that of mechanically exfoliated monolayers, which indicates that the D exciton resonance is enhanced in the spherical nanoantennas. Strong extinction in the range from 650 to 1100 nm is observed, which is attributed to the A and B excitons and two resonances of the dielectric antenna. The magnetic and electric resonance modes will be theoretically analyzed and discussed later.
3.3 SHG enhancement of MoS2 nanoantennas
The SHG behaviors of the MoS2 nanosheets, nanodisks, and nanospheres are investigated. The fundamental laser wavelength is tuned from 760 to 880 nm and the corresponding SHG wavelength is around the resonances of the C and D excitons. Fig. 3a presents the SHG spectra of the MoS2 nanoantennas with 2R = 310 nm and with the excitation laser wavelength λexc = 760, 780, 800, 820, 840, 860, and 880 nm; Fig. 3b clearly shows that the corresponding SHG intensity has a maximum when λSHG = 400 nm, which is in resonance with the D exciton of MoS2 monolayers. The SHG intensities of the exfoliated MoS2 nanosheets and the synthesized nanodisks monotonously increase as the excitation wavelength λexc is increased from 760 to 880 nm (see Fig. 3). This can be explained on the basis of the two-photon resonance excitation of the C exciton.
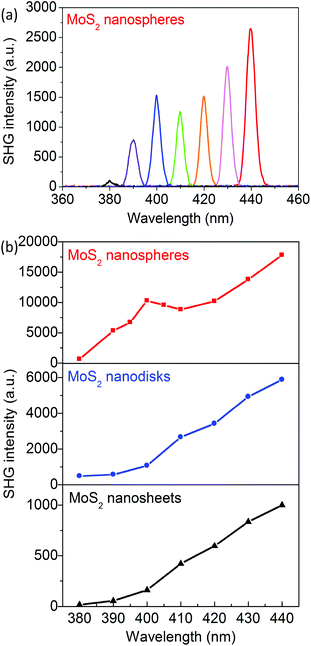 |
| Fig. 3 Enhanced SHG of the synthesized MoS2 nanosheets and nanospheres. (a) SHG spectrum of MoS2 nanospheres with excitation laser wavelength λexc = 760, 780, 800, 820, 840, 860, and 880 nm. (b) SHG peak intensity of MoS2 nanospheres (red lines), nanodisks (blue lines), and exfoliated monolayers (black lines) as a function of λexc. The MoS2 nanospheres exhibit a maximal SHG intensity at 400 nm around the D exciton of monolayers; the corresponding SHG enhancement factor FSHG reaches 52. | |
Compared to the exfoliated MoS2 nanosheets, both synthesized MoS2 nanodisks and nanospheres show significant enhancements of SHG in the wavelength range of 380–440 nm. The maximal SHG enhancement factor FSHG of the MoS2 nanodisks with 2R = 220 nm is 14 times at λSHG = 390 nm. The FSHG of the MoS2 nanospheres with 2R = 310 nm becomes 52 times larger at λSHG = 400 nm around the D exciton resonance. It decreases to ∼12 times as λSHG is increased to 440 nm.
3.4 Optical resonances and enhanced SHG of ReS2@Au hybrid nanoantennas
Colloidal disk-like and sphere-like ReS2 nanoantennas are also synthesized using a similar approach (see SEM images in Fig. 4a and b).56 ReS2@Au plasmon–dielectric hybrid nanoantennas are obtained by growing Au nanoparticles on the surface of the ReS2 nanospheres via a light-induced reduction reaction (see TEM and HRTEM in Fig. 4c).
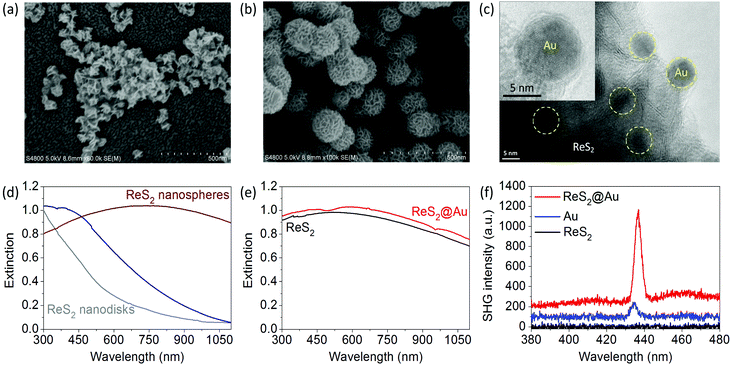 |
| Fig. 4 Nanostructure and linear and nonlinear resonances of ReS2 and ReS2@Au nanoantennas. (a) (b) SEM images of ReS2 nanodisks and nanospheres. (c) TEM image of ReS2@Au hybrids. Inset: HRTEM of an Au nanoparticle grown on the ReS2 nanosphere surface. (d) Extinction spectrum of ReS2 nanodisks and nanospheres. (e) Extinction spectrum of ReS2@Au hybrid nanoantennas. A weak dip appears at 500 nm and the surface plasmon resonance peak of Au nanoparticles is strongly modulated by the coupling of two nanoantennas. Inset: TEM image of Au nanoparticles on ReS2 nanospheres. (f) SHG of Au nanoparticles is enhanced in ReS2@Au hybrid nanoantennas. | |
The Au nanoparticles have an average diameter of 8 nm and are partially embedded inside the ReS2 nanosheets (see the TEM image in Fig. 4c and the HRTEM image in the inset). The band edge of ReS2 is around 800 nm. The resonance of the ReS2 nanodisks with 2R ∼ 150 nm is very weak owing to the small lateral sizes and very small aspect ratios.
The resonance wavelength of the ReS2 nanospheres is tunable in the range of 400–900 nm (Fig. 4d); the ReS2 nanospheres with 2R ∼ 200 nm exhibit strong resonances with a broadened peak around 720 nm. Fig. 4e presents the extinction spectrum of the hybrid nanostructures, which cannot be obtained by adding together the spectra of Au and ReS2. This result suggests the coupling of the plasmon and dielectric nanoantennas. A weak dip at 500 nm appears, and the prominent surface plasmon resonance of the spherical Au nanoparticles is significantly broadened. The small-sized spherical Au nanoparticles have a very weak SHG efficiency owing to the central symmetry.57–60 The SHG of the ReS2 nanospheres is not observed in the excitation laser wavelength range of 750–900 nm.
However, a much stronger SHG from the ReS2@Au hybrid nanostructures is observed (Fig. 4f). The corresponding SHG enhancement factor FSHG is 8.5 at λexc = 870 nm. The enhanced SHG of the hybrid nanostructures is mainly attributed to the local field enhancement near the surface of the ReS2 nanospheres and the coupling of two types of nanoantennas at the fundamental excitation wavelength. Therefore, the strong local field of the dielectric nanoantenna enhances both the SHG of MoS2 and ReS2@Au.
3.5 Calculations of the magnetic and electric resonance modes of MoS2 nanoantennas
To reveal the physical mechanism of the largely enhanced SHG of MoS2 nanospheres, we calculate and analyze the electric and magnetic resonances of the optical nanoantennas. For simplification, we calculate the extinction coefficient σext/V0 of an ideal MoS2 nanosphere and nanoellipsoid, where V0 = 4πR3/3 is the volume of the sphere. As shown in Fig. 5a, the resonance wavelength of the ideal nanosphere increases linearly with the diameter D of the nanosphere. The higher-order resonances become prominent when D > 280 nm. Fig. 5b presents the aspect-ratio (ρ) dependence of the extinction spectrum σext of the MoS2 nanoellipsoid (D = 330 nm, ρ = 1.00, 0.95, 0.90, 0.85, and 0.80). The resonance wavelength blue-shifts as the value of ρ is decreased.
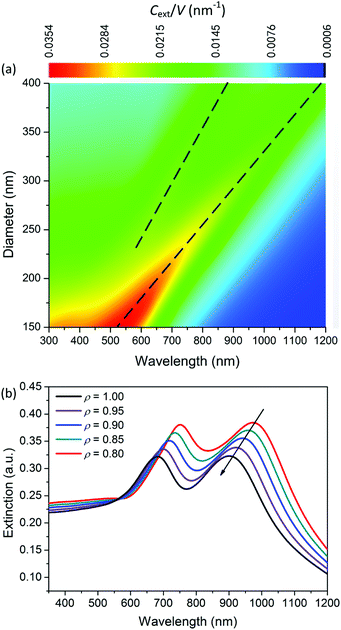 |
| Fig. 5 Calculated size and shape dependence of the resonances of MoS2 nanoantennas. (a) Size dependence of the extinction spectrum (σext/V0) of a solid MoS2 nanosphere with dielectric constants of monolayers. The resonant wavelength increases linearly with the diameter 2R of the nanospheres and the higher-order resonances become prominent when 2R > 280 nm. (b) Aspect-ratio dependence of the extinction spectrum σext of ellipsoid MoS2 nanospheres (2R = 310 nm, ρ = 1.00, 0.95, 0.90, 0.85, and 0.80). The excitation polarization is along the long axis of the oblate ellipsoid. The resonance wavelength blue-shifts as the value of ρ decreases. | |
Fig. 6a shows the first- (b1 and a1) and second- (b2 and a2) order magnetic and electric resonances on the extinction spectrum of a MoS2 nanosphere with D = 300 nm. Compared to the electric dipole resonance (a1), the magnetic dipole resonance (b1) has a stronger peak intensity with a better quality factor and longer wavelength. The resonance peaks of the magnetic dipole and quadrupole (b1 and b2) are located at 920 and 680 nm, which are the positions of the two peaks exhibited on the extinction spectrum. The extinction dip around 780 nm in the black curve is not induced by the Fano resonance due to the interaction between the electric and magnetic dipole modes. Therefore, the observed SHG enhancement is not induced by the Fano resonance.
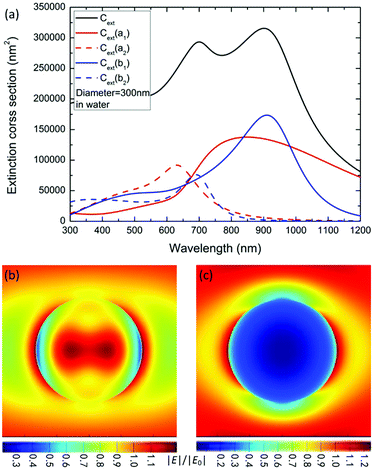 |
| Fig. 6 Multipole magnetic and electric resonance modes of a solid MoS2 nanosphere with 2R = 300 nm. (a) Electric (red line), magnetic (blue line), and total extinction (black lines) resonances of spherical nanoantennas. The resonance peaks of the first- and second-order magnetic modes are located at 920 and 700 nm, which are observable in the extinction spectrum. (b) Local electric field distribution |E|/|E0| of the nanosphere induced by the magnetic and electric dipole resonance at the wavelength of 800 nm. (c) Local field |E|/|E0| induced by the multipolar resonances at the wavelength of 400 nm. | |
Therefore, we can find out the magnetic resonance peaks from the extinction spectrum. At the wavelength of 800 nm, the electric dipole resonance is slightly stronger than the magnetic one. A combination of the magnetic and electric dipole resonance leads to a strong local field enhancement within and on the surface of the nanosphere (see Fig. 6b), which leads to a more efficient two-photon excitation for SHG. Differently, the plasmonic antennas with Fano interference exhibit strong SHG enhancement when the fundamental excitation wavelength matches the Fano dip.61,62
At the wavelength of 400 nm, the electric and magnetic quadrupole resonances have approximately the same intensity. A very strong scattering effect is observed in Fig. 6c, which brings the advantage of more efficient emission of SHG.
3.6 Discussion on the SHG enhancement factor
The SHG enhancement factor FSHG of the MoS2 nanosheets in the spherical dielectric nanoantennas can be approximately expressed as | FSHG(2ω) = η(2ω)|f(2ω)|2|f(ω)|4, | (1) |
where η(2ω) is the enhancement of the SHG excitation and emission efficiency, and f(ω) and f(2ω) represent the local field enhancement factors at the fundamental laser and SHG frequencies, respectively. The combination of the magnetic and electric dipole resonances leads to a large |f(ω)|4 within and near the surface of the dielectric nanoantenna as illustrated in Fig. 6b.
The real MoS2 nanospheres consisting of curly and interlaced nanosheets together with the random porous nanostructure increase the magnetic resonance strength and further enhance the local field of the nanosheets. η(2ω) is enhanced by the quadrupolar magnetic and electric resonances and also significantly enhanced by the two-photon resonance excitation of the exciton of monolayers,35 which results in a maximal SHG intensity of MoS2 nanospheres at the wavelength of 400 nm.
For the ReS2@Au hybrid nanospheres, the coupling of the plasmonic and dielectric nanoantennas re-distributes the local field of the Au nanoparticles, which results in a stronger local field enhancement and a larger field gradient along the radial direction of the ReS2 nanospheres. Therefore, both |f(ω)|4 and η(2ω) of the small Au nanoparticles are enhanced by the dielectric nanoantenna. Except the enhancement of SHG, the strong resonance of the electric as well as the magnetic modes of these dielectric nanoantennas can also be used to enhance other linear and nonlinear optical processes.
4. Conclusions
In summary, colloidal MoS2 and ReS2 nanoantennas consisting of 2D nanosheets are synthesized. This new form of dielectric nanoantennas exhibits strong electric as well as magnetic resonances, and the resonant wavelengths are tunable in the visible region by adjusting their size and aspect ratio. Compared to exfoliated MoS2 nanosheets, the synthesized MoS2 nanospheres exhibit 52-fold enhancement of SHG at the wavelength of 400 nm, which is ascribed to the two-photon resonance excitation of the D exciton enhanced by the multiple magnetic and electric modes of the dielectric antenna. Moreover, a significantly enhanced SHG of small-sized Au nanoparticles is also observed on ReS2@Au hybrid nanostructures owing to the coupling of plasmon and dielectric nanoantennas. This new class of nanoantennas consisting of 2D materials will have applications ranging from nonlinear photonics to photochemistry.
Author contributions
S. J. D. and Z. J. L. prepared the samples and recorded the absorption spectra. S. J. D. and L. Z. performed the experimental data analysis. Y. M. X. and G. M. P. performed the FDTD simulations and analysis. Y. H. Q. and K. C. performed the SHG and TEM measurements. H. Q. L., J. F. W. and Q. Q. W. were responsible for the experimental design, theoretical analysis and interpretation as well as the writing, revision and finalisation of the manuscript.
Conflicts of interest
There are no conflicts to declare.
Acknowledgements
We thank Zi-Qiang Cheng and Qiang Wang for the assistance in sample preparations and SEM measurements. This work was supported by the National Program on Key Science Research of China (2017YFA0303402), the Natural Science Foundation of China (NSFC) (11174042 and 11374039), and the China Postdoctoral Science Foundation (2016M602338).
Notes and references
- J. M. Hamm and O. Hess, Science, 2013, 340, 1298–1299 CrossRef PubMed.
- K. F. Mak, C. Lee, J. Hone, J. Shan and T. F. Heinz, Phys. Rev. Lett., 2010, 105, 136805 CrossRef PubMed.
-
A. V. Kolobov and J. Tominaga, Chemistry of Chalcogenides and Transition Metals, Springer International Publishing, Switzerland, 2016 Search PubMed.
- H. Zeng, J. Dai, W. Yao, D. Xiao and X. Cui, Nat. Nanotechnol., 2012, 7, 490–493 CrossRef CAS PubMed.
- D. Xiao, G. B. Liu, W. Feng, X. Xu and W. Yao, Phys. Rev. Lett., 2012, 108, 196802 CrossRef PubMed.
- K. F. Mak and J. Shan, Nat. Photonics, 2016, 10, 216–226 CrossRef CAS.
- Q. H. Wang, K. Kalantar-Zadeh, A. Kis, J. N. Coleman and M. S. Strano, Nat. Nanotechnol., 2012, 7, 699–712 CrossRef CAS PubMed.
- S. Lei, F. Wen, B. Li, Q. Wang, Y. Huang, Y. Gong, Y. He, P. Dong, J. Bellah, A. George, L. Ge, J. Lou, N. J. Halas, R. Vajtai and P. M. Ajayan, Nano Lett., 2015, 15, 259–265 CrossRef CAS PubMed.
- Z. Yin, H. Li, H. Li, L. Jiang, Y. Shi, Y. Sun, G. Lu, Q. Zhang, X. Chen and H. Zhang, ACS Nano, 2012, 6, 74–80 CrossRef CAS PubMed.
- O. Lopez-Sanchez, D. Lembke, M. Kayci, A. Radenovic and A. Kis, Nat. Nanotechnol., 2013, 8, 497–501 CrossRef CAS PubMed.
- M. Bernardi, M. Palummo and J. C. Grossman, Nano Lett., 2013, 13, 3664–3670 CrossRef CAS PubMed.
- A. B. Laursen, S. Kegnaes, S. Dahl and I. Chorkendorff, Energy Environ. Sci., 2012, 5, 5577–5591 CAS.
- K. L. Seyler, J. R. Schaibley, P. Gong, P. Rivera, A. M. Jones, S. Wu, J. Yan, D. G. Mandrus, W. Yao and X. Xu, Nat. Nanotechnol., 2015, 10, 407–411 CrossRef CAS PubMed.
- Y. Li, Y. Rao, K. F. Mak, Y. You, S. Wang, C. R. Dean and T. F. Heinz, Nano Lett., 2013, 13, 3329–3333 CrossRef CAS PubMed.
- W. T. Hsu, Z. A. Zhao, L. J. Li, C. H. Chen, M. H. Chiu, P. S. Chang, Y. C. Chou and W. H. Chang, ACS Nano, 2014, 8, 2951–2958 CrossRef CAS PubMed.
- N. Kumar, S. Najmaei, Q. Cui, F. Ceballos, P. M. Ajayan, J. Lou and H. Zhao, Phys. Rev. B: Condens. Matter, 2013, 87, 161403(R) CrossRef.
- L. M. Malard, T. V. Alencar, A. P. M. Barboza, K. F. Mak and A. M. de Paula, Phys. Rev. B: Condens. Matter, 2013, 87, 201401(R) CrossRef.
- M. Grüning and C. Attaccalite, Phys. Rev. B: Condens. Matter, 2014, 89, 081102 CrossRef.
- A. Kudryavtsev, S. Lavrov, A. Shestakova, L. Kulyuk and E. Mishina, AIP Adv., 2016, 6, 095306 CrossRef.
- X. Yin, Z. Ye, D. A. Chenet, Y. Ye, K. O'Brien, J. C. Hone and X. Zhang, Science, 2014, 344, 488–490 CrossRef CAS PubMed.
- R. Wang, H. C. Chien, J. Kumar, N. Kumar, H. Y. Chiu and H. Zhao, ACS Appl. Mater. Interfaces, 2014, 6, 314–318 CAS.
- S. Zhang, N. Dong, N. McEvoy, M. O. Brien, W. Sinéad, N. C. Berner, C. Yim, Y. Li, X. Zhang, Z. Chen, L. Zhang, G. S. Duesberg and J. Wang, ACS Nano, 2015, 9, 7142–7150 CrossRef CAS PubMed.
- C. Greg and G. W. Leach, Langmuir, 2006, 22, 8995–9001 CrossRef PubMed.
- K. Wierzbowski, S. Florian, R. Heimbach, M. Jahnke and W. K. Finley, Nano Lett., 2017, 17, 392–398 CrossRef PubMed.
- Z. Mervin, Z. Ye and R. Suzuki, Light: Sci. Appl., 2016, 5, 16131 CrossRef.
- A. Chernikov, T. C. Berkelbach, H. M. Hill, A. Rigosi, Y. Li, O. B. Aslan, D. R. Reichman, M. S. Hybertsen and T. F. Heinz, Phys. Rev. Lett., 2014, 113, 076802 CrossRef PubMed.
- Z. Ye, T. Cao, K. O. Brien, H. Zhu, X. Yin, Y. Wang, S. G. Louie and X. Zhang, Nature, 2014, 513, 214–218 CrossRef CAS PubMed.
- M. M. Ugeda, A. J. Bradley, S. F. Shi, F. H. da Jornada, Y. Zhang, D. Y. Qiu, W. Ruan, S. K. Mo, Z. Hussain, Z. X. Shen, F. Wang, S. G. Louie and M. F. Crommie, Nat. Mater., 2014, 13, 1091–1095 CrossRef CAS PubMed.
- A. R. Klots, A. K. M. Newaz, B. Wang, D. Prasai, H. Krzyzanowska, J. Lin, D. Caudel, N. J. Ghimire, J. Yan, B. L. Ivanov, K. A. Velizhanin, A. Burger, D. G. Mandrus, N. H. Tolk, S. T. Pantelides and K. I. Bolotin, Sci. Rep., 2014, 4, 6608 CrossRef CAS PubMed.
- D. Y. Qiu, F. H. da Jornada and S. G. Louie, Phys. Rev. Lett., 2013, 111, 216805 CrossRef PubMed.
- L. A. King, W. Zhao, M. Chhowalla, D. J. Rileya and G. Eda, J. Mater. Chem. A, 2013, 1, 8935–8941 CAS.
- L. F. Mattheis, Phys. Rev. Lett., 1973, 30, 784–787 CrossRef.
- R. Coehoorn, C. Haas, J. Dijkstra, C. J. F. Flipse, R. A. de Groot and A. Wold, Phys. Rev. B: Condens. Matter, 1987, 35, 6195–6202 CrossRef CAS.
- A. D. Yoffe, Annu. Rev. Mater. Sci., 1973, 3, 147–170 CrossRef CAS.
- G. Wang, X. Marie, I. Gerber, T. Amand, D. Lagarde, L. Bouet, M. Vidal, A. Balocchi and B. Urbaszek, Phys. Rev. Lett., 2015, 114, 097403 CrossRef CAS PubMed.
- C. T. Le, D. J. Clark, F. Ullah, J. I. Jang, V. Senthilkumar, Y. Sim, M. J. Seong, K. H. Chung, J. W. Kim, S. Park, S. H. Rhim, G. Kim and Y. S. Kim, ACS Photonics, 2017, 4, 38–44 CrossRef CAS.
- J. Sun, Y. J. Gu, D. Y. Lei, S. P. Lau, W. T. Wong, K. Y. Wong and H. L. W. Chan, ACS Photonics, 2016, 3, 2434–2444 CrossRef CAS.
- H. Chen, J. Yang, E. Rusak, J. Straubel, R. Guo, Y. W. Myint, J. Pei, M. Decker, I. Staude, C. Rockstuhl, Y. Lu, Y. S. Kivshar and D. Neshev, Sci. Rep., 2016, 6, 22296 CrossRef CAS PubMed.
- X. Liu, Q. Zhang, W. K. Chong, J. N. Yip, X. Wen, Z. Li, F. Wei, G. Yu, Q. Xiong and T. C. Sum, ACS Nano, 2015, 9, 5018–5026 CrossRef CAS PubMed.
- I. S. Lim, J. Ouyang, J. Luo, L. Wang, S. Zhou and C.-J. Zhong, Chem. Mater., 2005, 17, 6528–6531 CrossRef CAS.
- S. Gómez-Graña, C. Fernández-López, L. Polavarapu, J.-B. Salmon, J. Leng, I. Pastoriza-Santos and J. Pérez-Juste, Chem. Mater., 2015, 27, 8310–8317 CrossRef.
- M. Nguyen, N. Felidj and C. Mangeney, Chem. Mater., 2016, 28, 3564–3577 CrossRef CAS.
- R. Guo, E. Rusak, I. Staude, J. Dominguez, M. Decker, C. Rockstuhl, I. Brener, D. N. Neshev and Y. S. Kivshar, ACS Photonics, 2016, 3, 349–353 CrossRef CAS.
- A. Devilez, B. Stout and N. N. Bonod, ACS Nano, 2010, 4, 3390–3396 CrossRef CAS PubMed.
- X. Zeng, W. Yu, P. Yao, Z. Xi, Y. Lu and P. Wang, Opt. Express, 2014, 22, 14517 CrossRef PubMed.
- P. Mühlschlegel, H. J. Eisler, O. J. F. Martin, B. Hecht and D. W. Pohl, Science, 2005, 308, 1607–1609 CrossRef PubMed.
- S. Zhang, R. Jiang, Y. M. Xie, Q. Ruan, B. Yang, J. Wang and H. Q. Lin, Adv. Mater., 2015, 27, 7432–7439 CrossRef CAS PubMed.
- R. Regmi, J. Berthelot, P. M. Winkler, M. Mivelle, J. Proust, F. Bedu, I. Ozerov, T. Begou, J. Lumeau, H. Rigneault, M. García-Parajó, S. Bidault, J. Wenger and N. Bonod, Nano Lett., 2016, 16, 5143–5151 CrossRef CAS PubMed.
- T. Lei, S. Wu, Y. Zhang, J. Liu, H. Guo and Z. Zhang, Rare Met. Mater. Eng., 2013, 42, 2477 CrossRef CAS PubMed.
- P. B. Johnson and R. W. Christy, Phys. Rev. B: Solid State, 1972, 6, 4370–4379 CrossRef CAS.
- Q. Liu, X. Li, Q. He, A. Khalil, D. Liu, T. Xiang, X. Wu and L. Song, Small, 2015, 11, 5556–5564 CrossRef CAS PubMed.
- T. Yang, Y. Chen, B. Qu, L. Mei, D. Lei, H. Zhang, Q. Li and T. Wang, Electrochim. Acta, 2014, 115, 165–169 CrossRef CAS.
- L. Maa, W. X. Chena, H. Li and Z. D. Xua, Mater. Chem. Phys., 2009, 116, 400–405 CrossRef.
- G. Eda, H. Yamaguchi, D. Voiry, T. Fujita, M. Chen and M. Chhowalla, Nano Lett., 2011, 11, 5111–5116 CrossRef CAS PubMed.
- J. H. Sun, Y. J. Gu, D. Y. Lei, S. P. Lau, W. T. Wong, K. Y. Wong and H. L. W. Chan, ACS Photonics, 2016, 3, 2434–2444 CrossRef CAS.
- F. Qi, Y. Chen, B. Zheng, J. He, Q. Li, X. Wang, B. Yu, J. Lin, J. Zhou, P. Li and W. Zhang, J. Mater. Sci., 2017, 52, 3622–3629 CrossRef CAS.
- A. Capretti, E. F. Pecora, C. Forestiere, L. D. Negro and G. Miano, Phys. Rev. B: Condens. Matter, 2014, 89, 125414 CrossRef.
- I. Russier-Antoine, E. Benichou, G. Bachelier, C. Jonin and P. F. Brevet, J. Phys. Chem. C, 2007, 111, 9044–9048 CAS.
- J. I. Dadap, J. Shan and T. F. Heinz, J. Opt. Soc. Am. B, 2004, 21, 1328–1346 CrossRef CAS.
- J. Butet, I. Russier-Antoine, C. Jonin, N. Lascoux, E. Benichou and P. F. Brevet, Nano Lett., 2012, 12, 1697–1701 CrossRef CAS PubMed.
- S. D. Liu, E. S. P. Leong, G. C. Li, Y. Hou, J. Deng, J. H. Teng, H. C. Ong and D. Y. Lei, ACS Nano, 2016, 10, 1442–1453 CrossRef CAS PubMed.
- S. Zhang, G. C. Li, Y. Chen, X. Zhu, S. D. Liu, D. Y. Lei and H. Duan, ACS Nano, 2016, 10, 11105–11114 CrossRef CAS PubMed.
Footnotes |
† Electronic supplementary information (ESI) available. See DOI: 10.1039/c7nr06293a |
‡ These authors equally contributed to this work. |
|
This journal is © The Royal Society of Chemistry 2018 |