DOI:
10.1039/C7NR05499H
(Paper)
Nanoscale, 2018,
10, 791-799
Upconverting nanocomposites with combined photothermal and photodynamic effects†
Received
26th July 2017
, Accepted 3rd December 2017
First published on 6th December 2017
Abstract
Lanthanide-doped upconverting nanoparticles (UCNPs) have been studied for diverse biomedical applications due to their inherent ability to convert near-infrared (NIR) excitation light to higher energies (spanning the ultraviolet, visible, and NIR regions). To explore additional functionalities, rational combination with other optically active nanostructures may lead to the development of new multimodal nanoplatforms with theranostic (therapy and diagnostic) capabilities. Here, we develop a nanocomposite consisting of NaGdF4:Er3+, Yb3+ UCNPs, mesoporous silica (SiO2), gold nanorods (GNRs) and a photosensitizer, with integrated functionalities including luminescence imaging, photothermal generation, nanothermometry and photodynamic effects. Under 980 nm irradiation, GNRs and UCNPs are simultaneously excited due to the overlap between the surface plasmon resonance of the GNRs and the absorption of the UCNPs leading to plasmonic enhancement of the upconverted luminescence, while concomitantly creating a temperature gradient. The temperature increase can be determined from the intensity ratio of the upconverted green emission of the UCNPs. Finally, a photosensitizer, zinc phthalocyanine, was loaded into the mesoporous SiO2. Upon laser irradiation, the upconverted visible light subsequently activates the photosensitizer to release reactive oxygen species. The multifunctional GNR@SiO2@UCNPs nanocomposites showed strong luminescence signal when incubated in HeLa cervical cancer cells, making them ideal bioprobes for future theranostic applications.
Introduction
In recent years, lanthanide (Ln3+)-doped upconverting nanoparticles (UCNPs) have been intensively investigated, largely focusing on potential applications such as nanoscale thermometry, diagnostics and therapy. In particular, significant research has focused on their use as multifunctional nanoscale platforms with combined therapeutic and diagnostic (theranostic) modalities.1–3 The rising interest in UCNPs stems from their unorthodox excitation schemes where they can be excited with near-infrared (NIR) light and ultimately emit higher energy photons spanning the ultraviolet (UV), visible and NIR regions via a multiphoton excitation process, known as upconversion.4–6 The upconverted (or anti-Stokes) luminescence of UCNPs is advantageous when compared with conventional (Stokes) fluorescence from other nanomaterials, for several reasons. The NIR excitation light causes minimal photodamage, induces practically no autofluorescence background, and can penetrate biological tissues to a much greater extent.7,8 As an added benefit, some of the upconverted Ln3+ emission bands are sensitive to the surrounding temperatures, thereby allowing them to be effectively used as thermal nanoprobes (nanoscale thermometers also known as nanothermometers). One of the most well-known examples of an optical nanothermometer exploits the temperature sensitive emissions of the Er3+ ion. In particular, the relative intensity of the two green emission bands emanating from the 2H11/2 and 4S3/2 excited states of Er3+ change as a function of temperature. Consequently, Er3+-doped UCNPs have been used as non-contact, non-invasive optical nanothermometers to measure the temperature of living cells.9,10
In parallel to research on UCNPs, a significant body of work has focused on applying the heat generation capabilities of certain nanostructures, such as noble metal nanoparticles, for the photothermal therapy of cancer (also known as hyperthermia).11–13 Metal nanoparticles possess a high optical absorption coefficient and thermal dissipation rate, which is desired for highly efficient photothermal therapy (PTT). This allows these nanostructures to efficiently convert photon energy into heat following laser irradiation of their surface plasmon resonance (SPR), the resonant oscillation of conduction electrons at the metal interface, subsequently resulting in the controlled death of surrounding cells.14,15 Among metal nanostructures for PTT, gold nanorods (GNRs) are considered to be one of the most promising and versatile platforms.16–18 GNRs with fine-tuned optical properties can be excited by NIR light and simultaneously exhibit excellent photothermal conversion effects due to high surface-to-volume ratios and can thus generate efficient and localized hyperthermia.19 However, excess use of cetyltrimethylammonium bromide (CTAB) as a bilayer stabilizer around the GNR surface during their preparation would restrict their clinical application, resulting from the cytotoxicity caused by the CTAB capping molecules.20 To address this challenge, surface functionalization of GNRs has been investigated to further their potential in nanomedicine-based theranostic approaches.21 Mesoporous silica (SiO2) is an excellent option for surface functionalization and has been successfully used to coat GNRs. This mesoporous coating not only reduces the cytotoxicity and aggregation of GNRs, but also imparts drug (or cargo) loading ability while providing an excellent scaffold for subsequent chemical modification of the surface with additional moieties.22,23
The combination of these two optically excited nanostructures onto a single nanoplatform could lead to additional functionalities. For instance, controlling temperature in hyperthermia applications is a critical factor preventing healthy cells from also being destroyed (and consequently inducing severe side effects).24 Thus, coupling the nanoheaters with “built-in” nanothermometers would minimize the possibility of destroying healthy tissue surrounding the hyperthermia target and accordingly, the patient's side effects. In addition, when UCNPs and GNRs are in close proximity, it has been previously demonstrated that their interaction could influence the luminescence intensity of the UCNPs. For example, if the SPR of the GNRs was tuned to the excitation frequency of UCNPs (typically 980 nm, if Yb3+ is used as a sensitizer), the local electromagnetic field would become much larger and the luminescence intensity would be enhanced.25 For this enhancement to occur, the distance between plasmonic metal nanoparticles and UCNPs is critical and using SiO2 as a spacer, for example, would lead to a noticeable enhancement of the upconverted luminescence intensity.26,27
Recently, additional functionalities could be attained from UCNPs through rational combination with other optically active nanostructures, resulting in promising multi-modal nanoplatforms combining therapeutic and diagnostic actions.28–30 In this work, we developed a multifunctional nanocomposite based on the combination of UCNPs with GNRs, prepared through a layer-by-layer assembly. The GNR cores were coated with a mesoporous SiO2 shell, which was decorated with NaGdF4:Er3+, Yb3+ UCNPs. This multifunctional nanocomposite is ideal for controlled photothermal heating since both the nanoheaters (GNRs at the core) and the nanothermometers (UCNPs decorated on the surface of the SiO2) can be excited in the NIR region at 980 nm. To add further functionality, zinc phthalocyanine (ZnPc) was loaded into the mesoporous layer. ZnPc, a model photodynamic therapy (PDT) drug has been shown to have high cytotoxic efficiency in cancer cells due to the generation of reactive oxygen species (ROS) including singlet oxygen following light excitation.31,32 Typically, ZnPc and its analogues are excited with visible light, which restricts its deployment in clinical cancer treatments due to the limited penetration depth of the excitation light. By employing UCNPs, this inherent drawback could be overcome since they can convert tissue penetrating NIR excitation light to visible, which can then be absorbed by the photosensitizer (ZnPc) thereby triggering the release of ROS for application in the photodynamic therapy of cancer. Here, we investigate the luminescence properties of these new GNR@SiO2@UCNPs nanoplatforms and study their ability for controlled photoheating/nanothermometry and photodynamic effects. Finally, we also investigated the cytotoxicity of this nanocomposite. The multifunctional nanocomposite developed here could not only be well suited for bioimaging, but also for future multi-pronged cancer theranostics through the combination of imaging, nanothermometry as well PTT and PDT effects.
Experimental section
Materials
The lanthanide oxides (Ln2O3 (99.99%), Ln = Gd, Yb, Er), trifluoroacetic acid (99%), sodium trifluoroacetate (98%), oleic acid (90%), 1-octadecene (90%), hydrogen tetrachloroaurate(III) hydrate (HAuCl4·3H2O), cetyltrimethylammonium bromide (CTAB, 98%), silver nitrate (AgNO3, >99%), L-ascorbic acid (C6H8O6, >99%), 5-bromosalicylic acid (>98.0%), zinc phthalocyanine (ZnPc, 95%) were purchased from Alfa Aesar. Sodium borohydride (NaBH4, 99.99%), 3-aminopropyltriethoxysilane (APTES, 99%), 9,10-anthracenediyl-bis(methylene)dimalonic acid (ABDA), Triton™ X-100 were purchased from Sigma Aldrich. Ultrapure deionized water (Millipore system) was used throughout the experiments. All starting chemicals in this work were used without further purification.
Synthesis of (CF3COO)3Ln (Ln = Gd, Yb, Er) precursors
In a typical synthesis of the lanthanide trifluoroacetate precursors, 0.975 mmol (353.4 mg) Gd2O3, 0.025 mmol (9.6 mg, 2 mol% doping rate) Er2O3, and 0.25 mmol (98.5 mg, 20 mol% doping rate) Yb2O3 were mixed in a 100 mL three-neck round-bottom flask with 5 mL distilled water and 5 mL trifluoroacetic acid, followed by refluxing under magnetic stirring at 80 °C until a clear solution was obtained. Subsequently, the temperature was reduced to 60 °C to evaporate the residual trifluoroacetic acid and water. The dried precursor was isolated in the form of a colorless powder.
Synthesis of NaGdF4:Er3+, Yb3+ UCNPs
For the synthesis of NaGdF4:Er3+, Yb3+ UCNPs, 2.5 mmol (340 mg) sodium trifluoroacetate as well as 20 mL 1-octadecene, 10 mL oleic acid and 10 mL oleylamine were added to the obtained (CF3COO)3Ln precursor. Subsequently, the mixture was degassed for 30 min at 100 °C under vacuum and magnetic stirring. The reaction mixture was then heated to 240 °C under stirring with a gentle argon flow and maintained at this temperature for 2 h. Subsequently, the solution was allowed to cool to room temperature and the resultant oleate-capped UCNPs were precipitated with ethanol and collected by centrifugation. The obtained product was washed three times with hexane/ethanol (4
:
1) and dispersed in 5 mL hexane as stock solution.
Oleate-citrate ligand exchange
To obtain water dispersible UCNPs, ligand exchange was carried out as previously described in the literature.33 60 mg of oleate-capped NaGdF4:Er3+, Yb3+ UCNPs obtained from the previous step were dispersed in 5 mL of hexane, and mixed with 5 mL of a 0.2 M trisodium citrate buffer (adjusted to pH 4). The mixture was kept on a shaker for 3 h, and subsequently transferred into a separation funnel, from which the aqueous phase containing the NaGdF4:Er3+, Yb3+ was collected. The UCNPs were precipitated with acetone (1
:
5 aqueous
:
organic ratio), collected by centrifugation and re-dispersed in 5 mL of trisodium citrate buffer (adjusted to pH 7). The dispersion was placed on the shaker for an additional 2 h. Finally, the UCNPs were precipitated with acetone, washed three times with acetone and water, collected by centrifugation and dispersed in 2 mL of water for further experiments.
Preparation of gold nanorods (GNRs)
The seed solution for GNRs was prepared as reported previously.34 5 mL of 0.5 mM HAuCl4 was mixed with 5 mL of 0.2 M CTAB solution. Subsequently, 0.60 mL of ice-cold 0.010 M NaBH4 was added under vigorous stirring, which resulted in a color change from yellow to brownish-yellow. The seed solution was kept at room temperature before use.
For the growth solution preparation, 0.36 g of CTAB together with 0.044 g of 5-bromosalicylic acid was dissolved in 10 mL of deionized water. With the solution at 30 °C, 0.60 mL of 4 mM AgNO3 was added and kept undisturbed for 15 min. Subsequently, 10 mL of 1 mM HAuCl4 was added, and 0.084 mL HCl (37 wt%) was added. After 15 min of slow stirring, 80 μL of 0.064 M ascorbic acid was slowly added to the mixture. The final step was the addition of 32 μL of the seed solution to the growth solution causing a gradual color change of the solution. The temperature of the growth solution was kept constant at 30 °C during the entire procedure.
Preparation of silica coated GNRs (GNR@SiO2)
To remove excess CTAB surfactant, the synthesized GNRs were washed by centrifugation and the sample was dispersed in 10 mL of deionized water. After that, 100 μL of 0.1 M NaOH was added. Following this step, three 30 μL injections of 20% TEOS in methanol were added under gentle stirring at 30 min intervals. The mixture was left to react for 2 days.
APTES functionalized GNR@SiO2
The solution of GNR@SiO2 was centrifuged and suspended in ethanol. Then, 20 μL of APTES was added and mixed by gentle shaking for another 6 h to accomplish the aminolization. Finally, the sample was centrifuged and washed with ethanol.
UCNPs attachment to GNR@SiO2
The APTES functionalized GNR@SiO2 was added drop wise to the solution of UCNPs under vigorous stirring. After 2 h, the final product (GNR@SiO2@UCNPs) was centrifuged out, and then redispersed either in ethanol or water for further characterization.
Measurement of singlet oxygen (1O2)
The 1O2 production was detected by singlet oxygen sensors using 9,10-anthracenediyl-bis(methylene) dimalonic acid (ABDA).35 Different samples in phosphate buffer (PBS) (pH 7–7.4) were mixed with ABDA (10 μM) and placed in a cuvette. The solution was irradiated by a 980 nm laser for different periods of time, and the generation of 1O2 resulted in the bleaching of ABDA absorption at 380 nm.
Cellular viability
The cell viability was investigated in HeLa cervical cancer cells. The cells were seeded onto 96-well plates with a density of 1 × 104 cells per well and incubated at 37 °C for 48 h. After cell attachment, different samples were added to the wells and subsequently incubated additionally at 37 °C for 24 h. Cell viability was measured using the 3-(4,5-dimethylthiazol-2-yl)-2,5-diphenyltetrazolium bromide (MTT) assay.36
Fluorescence cell image
For fluorescence imaging of HeLa cells incubated with GNR@SiO2@UCNPs, they were seeded in 6-well plates containing sterile cover slips and were kept in a sterile environment for 24 h. Then, cells were exposed to incubation with GNR@SiO2@UCNPs for 4 h at 37 °C. Subsequently, the cells were fixed with 3.5% formaldehyde in PBS for 20 min at 4 °C. Once they were washed three times with PBS, the cells were scanned using an in house designed confocal microscope (Fig. S1†). Laser excitation was performed using a 980 nm diode laser coupled to a single mode fiber (LU0975M500 model from LUMICS GmbH). The 980 nm laser radiation was first collimated and then focused into the HeLa cancer cell using a 100× 0.85 NA microscope objective. The fluorescence generated by the GNR@SiO2@UCNPs incorporated into the cells was collected by the same microscope objective. This collected luminescence, after passing through different filters was spectrally analyzed by a monochromator (iH320, Horiba) and recorded with a high sensitivity Si CCD camera (Synapse, Horiba). The system was optimized for the analysis of the green emission of erbium (Er3+) ions at approximately 550 nm. The HeLa cells were mounted on a 0.1 μm resolution XY stage such that it was possible to scan the HeLa cells with respect to the 980 nm laser spot.
Characterization
The crystalline phase of all nanostructures under investigation was analyzed by X-ray diffraction (XRD, Bruker D8 Advanced Diffractometer, Cu Kα radiation). Dynamic light scattering (DLS, Malvern, Zetasizer Nano S90) was used to determine the hydrodynamic diameter of the nanostructures. The morphology and size distribution of the GNRs and UCNPs were determined with a Philips CM200 high-resolution transmission electron microscope (HR-TEM), equipped with an energy-dispersive X-ray (EDX) unit. The UV-Visible (UV-VIS) absorption spectra were obtained using a UV-VIS spectrometer (Varian Cary 5000). Luminescent measurements were carried out under 980 nm excitation using a laser diode (Thorlabs fiber-coupled laser 305 diode). The laser beam was focused on the sample using a lens to obtain a spot with a Gaussian intensity distribution with a 0.4 mm diameter. The emitted light was collected by a lens in a 90° configuration, and then transferred to a spectrophotometer (Avaspec-2048L-USB2) using an optical fiber.
Results and discussion
Preparation and characterization of GNR@SiO2@UCNPs
To prepare the multifunctional nanocomposites based on UCNPs and GNRs with combined luminescence, photodynamic and photothermal properties for potential use in theranostics, a layer-by-layer procedure was employed. This multifunctional nanocomposite contains a mesoporous SiO2 layer separating the UCNPs decorated on the surface and the GNR core, which has multiple roles. First, it is used as a scaffold for the attachment of the UCNPs. Second, it acts as a spacer to separate the plasmonic nanostructure (GNR) and the fluorescent species (UCNPs) leading to minimal luminescence quenching and potentially, plasmonic enhancement. Finally, the pores can be loaded with photosensitizer molecules capable of transforming the upconverted visible radiation to ROS (i.e.1O2) adding another modality to the nanoplatform. To prepare this nanocomposite, a multistep synthetic method was proposed and summarized in Scheme 1. Initially, the NaGdF4:Er3+, Yb3+ UCNPs were synthesized by the previously described thermal decomposition method.37 As seen in Fig. 1A, the synthesized UCNPs were monodisperse and uniform with an average diameter of approximately 6.5 nm. The HR-TEM image of the UCNPs is shown in the Fig. 1B and the lattice fringes were clearly observed with a spacing of ∼0.275 nm, ∼0.317 nm assigned to the {200}, {111} planes of cubic (α-) phase NaGdF4:Er3+, Yb3+ UCNPs, respectively. This result is consistent with XRD studies (Fig. 2B), which confirmed that the UCNPs crystallized in the α-phase, and no impurity crystalline phase was found in the diffraction pattern. Separately, GNRs were prepared according to a previously published protocol38 and their morphology and average size were imaged by TEM (Fig. 1C). GNRs with an average of 72 nm in length and 12 nm in diameter were successfully synthesized, yielding an aspect ratio of approximately 6
:
1. To characterize the GNRs' absorption properties, a key parameter in the photogeneration of heat, their SPR was investigated using UV-VIS absorption spectroscopy. We observed the presence of two SPR absorption bands around 525 and 972 nm, due to the transverse and longitudinal plasmon absorptions, respectively (Fig. 2A). Subsequently, the SiO2 layer was grown on the surface of the GNRs39 where CTAB served as the organic template for the formation of the mesoporous SiO2 layer with a thickness of ca. 15 nm (see Fig. 1D), offering the opportunity for loading of the therapeutic photosensitizer molecules, and providing significant spacing between the GNRs and UCNPs that can facilitate plasmon enhanced upconversion emission intensity. Following the growth of the SiO2 layer, the longitudinal SPR of the GNR@SiO2 nanostructures exhibited a small red-shift (∼11 nm), to 983 nm (Fig. 2A), overlapping perfectly with the 980 nm excitation wavelength.
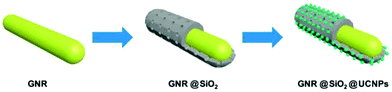 |
| Scheme 1 Schematic illustration of the synthetic procedure for the preparation of the GNR@SiO2@UCNPs nanocomposite. | |
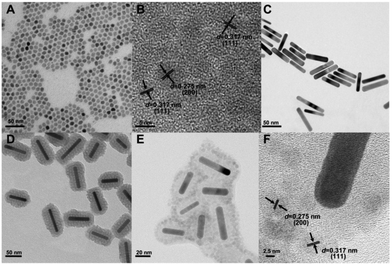 |
| Fig. 1 (A) TEM and (B) HR-TEM images of the synthesized NaGdF4:Er3+, Yb3+ UCNPs. TEM images of the (C) synthesized GNRs, (D) the SiO2 coated GNRs (GNR@SiO2), and (E) the hybrid SiO2 coated GNRs decorated with NaGdF4:Er3+, Yb3+ UCNPs (GNR@SiO2@UCNPs), (F) HR-TEM image of the GNR@SiO2@UCNPs interface. | |
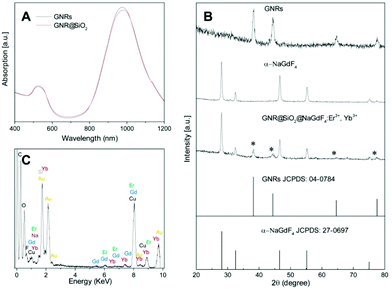 |
| Fig. 2 (A) Extinction spectra of GNRs and GNR@SiO2. (B) XRD patterns of GNRs, α-NaGdF4:Er3+, Yb3+, hybrid nanocomposite of GNRs and NaGdF4:Er3+, Yb3+ (GNR@SiO2@UCNPs). The standard pattern of pure GNRs (JCPDS file No: 04-0784), pure α-NaGdF4 (JCPDS file No: 27-0697) are provided for reference. The stars show the diffraction peaks of GNRs in the GNR@SiO2@UCNPs nanocomposite. (C) Elemental analysis of hybrid GNRs@SiO2@UCNPs nanocomposite by EDX. | |
After functionalization of the SiO2 coated GNRs using 3-aminopropyltriethoxylsilane (APTES), they were mixed together with the citrate-capped UCNPs. At pH 6 of the colloidal solution, the amino group of the APTES was fully protonated (NH4+), whereas the UCNPs possessed negative charge (cit−). Hence, electrostatic forces between the UCNPs and APTES functionalized GNR@SiO2 allowed for their surface decoration. DLS results (Fig. S2†) show that the hydrodynamic diameter of the nanocomposites reaches 142 nm, which is larger than that of GNR@SiO2 (122 nm). This additionally indicates that UCNPs are in fact attached onto the surface of GNR@SiO2 nanostructures. Fig. 1E shows the morphology of the SiO2 coated GNRs following attachment of the UCNPs as well as the interface between the UCNPs and GNR@SiO2 as characterized by HR-TEM. As shown in Fig. 1F, two lattice fringes from the NaGdF4:Er3+, Yb3+ UCNPs were visible, with spacing of ∼0.275 nm corresponding to the {200} planes and the larger lattice spacing of ∼0.317 nm matching the {111} crystal planes of the α-NaGdF4:Er3+, Yb3+ UCNPs. The assembly of the hybrid nanocomposite composed of NaGdF4:Er3+, Yb3+ UCNPs and GNRs could be further confirmed by XRD (Fig. 2B). The main diffraction peaks coincided well with the pure α-phase NaGdF4 crystal (JCPDS No: 27-0697) and face-centered cubic (fcc) structure of metallic Au (JCPDS No: 04-0784). In addition, compositional analysis by energy-dispersive X-ray microanalysis (EDX) indicated that Na, Gd, F, Yb, Er, Au, and Si elements were present in this hybrid nanocomposite (Fig. 2C), further confirming that it consists of GNR@SiO2 and UCNPs.
Photoluminescence properties of the GNR@SiO2@UCNPs
The upconversion mechanism as well as the energy state diagrams of Yb3+ and Er3+ ions are illustrated schematically in Fig. S3 of the ESI.† It is well known that NaGdF4:Er3+, Yb3+ UCNPs possess a large absorption at 980 nm and can be efficiently excited via a continuous wave NIR laser to induce the upconversion process. In this mechanism, the Yb3+ ions (the sensitizers) are excited from the ground state (2F7/2) to the excited state (2F5/2) and then undergo subsequent energy transfers to the Er3+ ions (the activators) in close proximity. This excites the Er3+ ions from the ground state (4I15/2) to the first intermediate excited state (4I11/2) and further to the 4F7/2 excited state. Following multiphonon (non-radiative) decay, the lower energy 2H11/2 and 4S3/2 emitting states are populated, which produce the green emission at 525 and 545 nm through radiative decay from the 2H11/2 and 4S3/2 excited states to the 4I15/2 ground state, respectively. Alternatively, the excited Er3+ ions in the 4S3/2 excited state can further decay non-radiatively to the lower-lying 4F9/2 excited state producing the observed red emission at approximately 660 nm from the radiative transition of the 4F9/2 excited state to the ground state. However, the 4F9/2 excited state can also be populated by direct energy transfer. In this case, once the Er3+ ion is excited to the 4I11/2 intermediate state following the first energy transfer from an excited Yb3+ ion, it can also undergo non-radiative decay from the 4I11/2 excited intermediate state to the lower 4I13/2 state. A transfer of energy from another excited Yb3+ ion in the 2F5/2 state in close proximity can then directly populate the red 4F9/2 emitting state.
Previous reports have demonstrated that the upconverted emission intensity could be used to monitor changes in temperature of the environment.9,10 For Er3+ ions, given that the difference in energy between the 2H11/2 and 4S3/2 excited states is only several hundred wavenumbers (cm−1), the lower energy 4S3/2 state could easily thermally populate the upper 2H11/2 state. Therefore, the populations of the 2H11/2 and 4S3/2 states are in thermal equilibrium and the relative intensity of these two excited states will vary as a function of temperature. This ratio can therefore be exploited for temperature sensing applications and is generally referred to as the Luminescence Intensity Ratio (LIR) technique. Thus for thermometry applications, the relative intensity ratio between the 2H11/2 → 4I15/2 and 4S3/2 → 4I15/2 emission bands could be calculated based on the Boltzmann distribution
| 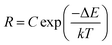 | (1) |
Where
R represents the LIR of the
2H
11/2 →
4I
15/2 and
4S
3/2 →
4I
15/2,
C is a constant, Δ
E is the energy gap between the two excited states (
2H
11/2 and
4S
3/2),
k is the Boltzmann constant, and
T is the absolute temperature.
Fig. 3A presents two upconversion emission spectra (excited at 980 nm) of the UCNPs at two different temperatures, 20 and 60 °C. Comparing both distinct bands between 515–535 nm and 535–570 nm, the ratio increases with temperature, and this ratio increment follows a linear relation with temperature, as can be observed in the graph in
Fig. 3B. In this case, the experimental determination of this ratio yields a direct temperature measurement. This increase in the ratio between the corresponding emitting peak intensities could therefore be employed for thermal sensing.
9,40 Afterwards, based on this ratio, the same procedure was followed to estimate the temperature of the GNR@SiO
2@UCNPs hybrid nanocomposite sample.
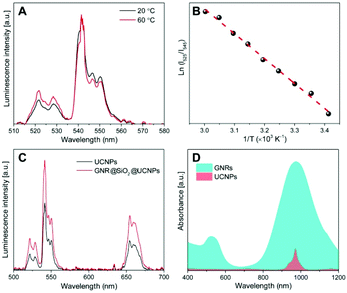 |
| Fig. 3 (A) Normalized upconversion luminescence spectra of UCNPs at two different temperatures. (B) The temperature dependence of the ratio calculated from the luminescence spectra obtained at various temperatures. Dots are experimental results and the red line is the best linear fit. (C) Upconversion luminescence spectra of UCNPs and GNR@SiO2@UCNPs. (D) Normalized absorption spectra of UCNPs and GNRs. | |
From the detailed analysis of data in Fig. 3C, the ratio of the upconversion intensity between the upconverted 515–535 nm and 535–570 nm emissions of the GNR@SiO2@UCNPs nanocomposite clearly increases when compared with that of the bare UCNPs at the same conditions. The thermal change of the GNR@SiO2@UCNPs nanocomposites under NIR excitation was determined by the previously obtained linear regression equation for the NaGdF4:Er3+, Yb3+ UCNPs. Under NIR excitation of 0.8 W the temperature in the excitation area of 0.4 mm in diameter, changed by approximately 1.0 and 7.3 °C for UCNPs alone and GNR@SiO2@UCNPs nanocomposites, respectively (Fig. S4†). In turn, this shows that the UCNPs attached to the surface of GNRs can be successfully used for real-time temperature sensing at the nanoscale, particularly for precise control of photothermal effect. Meanwhile, it is worth noting that the nanocomposites are quite stable after laser excitation, since the same luminescence intensity and heating properties could be observed after several cycles of laser irradiation, which demonstrates that UCNPs are not lost from the surface of GNR@SiO2 and no morphological changes occur to the GNRs (they retain their rod-like morphology). In this nanocomposite, the GNRs could therefore easily be used as nanoscale heaters when excited at 980 nm, while the UCNPs decorated on the surface of the SiO2 shell effectively behave as thermal sensors. Moreover, the total emission from the Er3+ ions in the GNR@SiO2@UCNPs nanocomposite was enhanced when compared with bare UCNPs. This is due to the fact that when the SPR of the GNRs overlaps with the excitation of the UCNPs (2F5/2 from Yb3+ and 4I11/2 from Er3+ at 980 nm), as shown in Fig. 3D, the energy harnessed by the plasmonic nanostructures (GNRs) could be transferred to the electrons of the Ln3+ dopants in the UCNPs, resulting in an increase in their absorption cross-section.25,41 Further to this, the SPR can affect the upconversion luminescence depending on the relative position of the SPR from the excitation or emission of UCNPs as well as the distance separating the UCNPs from the metal surface, that is to say, the thickness of the spacer layer between the UCNPs and the surface of the GNRs.42 There are three competitive effects that contribute to this behavior: the plasmonic field causing luminescence enhancement, the radiative decay rate from coupling of the upconversion emission with the SPR of the GNRs resulting in luminescence enhancement and the non-radiative energy transfer resulting in luminescence quenching. In this study, the SPR (980 nm) of the GNRs was far away from the upconversion emission, therefore, the radiative decay rate played a negligible role. If the fluorescent species (UCNPs) are close to the metal surface, the upconversion luminescence decreases, as the non-radiative energy transfer is dominant. With the increase of the distance between the two species (i.e. the thickness of the spacer layer in this case SiO2), the plasmonic field enhancement becomes dominant leading to the observed increase of the UCNP luminescence.
Characterization of ZnPc loaded GNR@SiO2@UCNPs and photodynamic effect
The photosensitizer ZnPc was selected as candidate to be loaded into the mesoporous silica and to deliver the desired photodynamic effect. A concentrated solution of ZnPc was mixed together with the GNR@SiO2@UCNPs until the ZnPc was absorbed into the porous SiO2. The ZnPc loading capacity was determined by the UV-VIS spectra and reached 1.8% (w/w). Meanwhile, the luminescence intensity of ZnPc loaded GNR@SiO2@UCNPs under NIR laser irradiation was also investigated and it was observed that a quenching of the luminescence occurred after ZnPc was loaded into the GNR@SiO2@UCNPs nanocomposite (Fig. 4A). In fact, the red emission (4F9/2 → 4I15/2) of the GNR@SiO2@UCNPs from Er3+ decreased by 54%, which was significantly more than the decrease in green emission (29%). This quenching resulted from efficient energy transfer between the UCNPs (energy donors) and the ZnPc molecules (energy acceptors) due to the favorable spectral overlap between the red emission of the UCNPs at 660 nm and the absorption of ZnPc at 665 nm (Fig. 4B). In addition, the ratio of the green emission bands discussed above remains almost unchanged after ZnPc loading in the GNR@SiO2@UCNPs nanocomposites indicating that the LIR technique can still be used for thermal sensing after loading.
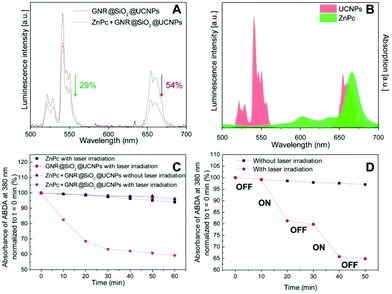 |
| Fig. 4 (A) Upconversion luminescence spectra of GNR@SiO2@UCNPs and ZnPc loaded GNR@SiO2@UCNPs under 980 nm laser irradiation. (B) Upconversion emission spectrum of NaGdF4:Er3+, Yb3+ UCNPs and UV-VIS absorption spectrum of ZnPc, indicating the partial spectral overlap between the ZnPc absorption and the UCNP emission. (C) Production of 1O2 under consumption of ABDA for different samples over time. (D) Production of 1O2 under consumption of ABDA in the absence and presence of laser irradiation. | |
The capacity of ROS production, in particular singlet oxygen (1O2), was assessed using ABDA as a probe molecule. As shown in Fig. 4C, three control groups were studied, ABDA incubated with ZnPc loaded GNR@SiO2@UCNPs without NIR laser irradiation (blue triangles), ZnPc molecules with NIR laser irradiation (black squares), and GNR@SiO2@UCNPs alone with NIR laser irradiation (red circles). No significant changes are observed for these control groups over time, which indicates that no 1O2 generation occurred. However, for ABDA incubated with ZnPc loaded GNR@SiO2@UCNPs following NIR laser irradiation, the absorbance of ABDA decreased significantly demonstrating that both ZnPc molecules and GNR@SiO2@UCNPs nanocomposites as well as 980 nm laser irradiation are necessary for the generation of 1O2. To further confirm that 1O2 production is triggered by laser irradiation, as shown in Fig. 4D, the suspension of ZnPc loaded GNR@SiO2@UCNPs was exposed to laser irradiation cycles of 10 minutes and the results clearly revealed that the 1O2 generation was reduced when the laser was switched off. Similar results were observed when the laser cycling was repeated. This enhanced 1O2 production upon laser irradiation could be attributed to the photodynamic process: UCNPs were excited by laser irradiation, and the emitted luminescence was absorbed by ZnPc molecules in the pores, subsequently generating reactive and cytotoxic oxygen species (ROS) that can eventually be exploited for cancer treatment.
In vitro cytotoxicity and imaging
To study the potential application of our multifunctional nanoplatforms for in vitro studies at the cellular level, both their cytotoxicity and capacity for cell labelling and imaging were studied. Firstly, the cytotoxicity of the UCNPs, GNR@SiO2 and GNR@SiO2@UCNPs was investigated by MTT assay on HeLa cervical cancer cells. The result in Fig. 5 demonstrates that there is no significant decrease in the cell viability of the UCNPs and GNR@SiO2 samples at a concentration of 50 μg ml−1. Compared with UCNPs and GNR@SiO2, a slightly higher cytotoxicity was observed after cell incubation with the GNR@SiO2@UCNPs nanocomposites; a surviving fraction of ca. ∼96.3% was observed after incubation for 24 h at a concentration of 50 μg ml−1. This slightly lower viability may be attributed to the positively APTES capped GNR@SiO2 nanostructures, which could lead to the cellular cytotoxicity in terms of causing mitochondrial and lysosomal damage.43,44
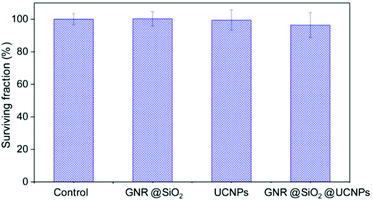 |
| Fig. 5 Percent survival of HeLa cancer cells incubated for a period of 24 h with GNR@SiO2, UCNPs and GNR@SiO2@UCNPs. Each point corresponds to the mean value ± SD from three different experiments. | |
Once the low cytotoxicity of the multifunctional platforms was evidenced, we proceeded with cell imaging studies. In doing so, HeLa cells were incubated with a solution containing the GNR@SiO2@UCNPs nanocomposites. Incubation time was set to 24 h. After incubation, cells were washed and placed in the confocal microscope. The 980 nm focal plane was set to match the axial location of the HeLa cells and these were scanned while recording the emission spectra in the 500–580 nm spectral range. A typical bright field (BF) optical image of an individual HeLa cell is included in Fig. 6A. The spatial variation of the green fluorescence intensity obtained when the 980 nm laser was scanned over the HeLa cell of Fig. 6A is shown in Fig. 6B. When both fluorescence and BF optical images are merged (see Fig. 6C) it is clear that the GNR@SiO2@UCNPs nanocomposites are incorporated within the HeLa cell since the luminescence signal is very well localized inside the cell. This suggests that the GNR@SiO2@UCNPs nanocomposites have been accumulated at a well-defined location inside the cell. This is in good agreement with previous work.45 It has been stated that agglomeration of UCNPs inside HeLa cells could take place for long incubation times (as in our case). This effect is a consequence of the intracellular transportation of nutrients from the cellular membrane to the Golgi apparatus. To verify that the green emission collected was generated by the GNR@SiO2@UCNPs nanocomposites, the emission spectra were recorded at two different locations inside the HeLa cell. Results are shown in Fig. 6D. When compared with the emission spectra included in Fig. 3 and 4, it is clearly observed that the fluorescence image was generated by the GNR@SiO2@UCNPs nanocomposites. Control experiments, by using HeLa cells incubated in a medium dispersion without GNR@SiO2@UCNPs nanocomposites were also conducted, revealing the complete absence of fluorescence contrast (see Fig. 6E–G).
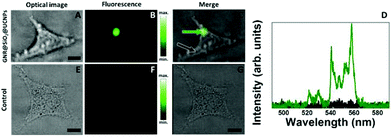 |
| Fig. 6 (A) BF optical image of a HeLa cancer cell incubated for a period of 24 h with GNR@SiO2@UCNPs. (B) Fluorescence image obtained by scanning the 980 nm laser spot over the HeLa cell in (A) and by recording the emitted intensity in the 500–580 nm spectral range. (C) Merge of optical and fluorescence images denoting accumulation of GNR@SiO2@UCNPs inside the HeLa cell. (D) Emission spectra as obtained at two different locations inside the cell denoted by green and black arrows in (C). (E), (F), and (G) are the BF optical image, microluminescence map, and merged image of a HeLa cell for control, respectively. Scale bars: 10 μm. | |
Conclusions
In summary, a GNR@SiO2@UCNPs nanocomposite was synthesized and characterized. We studied the photothermal generation as well as the luminescence properties of the GNR@SiO2@UCNPs and observed that after NIR excitation at 980 nm, significant heating was produced from the plasmonic GNRs at the core of the nanocomposite. It was determined that the ratio of the intensities of the green upconverted emission between the 2H11/2 → 4I15/2 and 4S3/2 → 4I15/2 transitions from the UCNPs functionalized on the surface of the mesoporous SiO2 shell surrounding the GNR cores changed as a function of temperature and could be exploited for thermal sensing resulting in a nanocomposite with both photothermal generation capabilities and real time temperature monitoring. Moreover, the total luminescence intensity of the UCNPs was increased in the presence of GNRs (compared to the UCNPs alone) and this enhancement was attributed to the overlap of the excitation of the UCNPs with the SPR of the GNRs at 980 nm as well to the mesoporous SiO2 between the UCNPs and GNR acting as a spacer to mitigate any non-radiative energy transfer between the plasmonic GNR and the luminescent UCNPs. Thus, the luminescence of the UCNPs was influenced by the localized electromagnetic field created by the GNRs, which leads to the observed enhancement in the luminescence intensity. ZnPc was selected as a photosensitizer to load into the mesoporous SiO2 layer and a quenching of the UCNPs was observed following loading of the pores. This demonstrated that the emitted upconverted luminescence was effectively absorbed by the ZnPc molecules, which subsequently generated the ROS. Finally, in vitro cell experiments were performed to assess the cytotoxicity of GNRs@SiO2@UCNPs. Results showed the absence of any cytotoxicity on the incubated cells thus determining that they were excellent biocompatible nanocomposites. Similarly, cell labeling experiments were performed and it was determined that the GNR@SiO2@UCNPs nanocomposites accumulated at a well-defined location inside the HeLa cells and were imaged via the upconverted green luminescence from inside the cells. These results confirm that GNR@SiO2@UCNPs have the potential to be used as NIR-excited multifunctional nanoplatforms for theranostic applications, specifically due to their photothermal and photodynamic capabilities.
Conflicts of interest
There are no conflicts to declare.
Acknowledgements
F. V. and F. R. acknowledge funding from the Natural Sciences and Engineering Research Council (NSERC Discovery Grants) of Canada and Fonds de Recherche du Québec-Nature et technologies (FRQNT) for supporting this research. F. V. also is grateful to the Fondation Sibylla Hesse for funding. Y. H. acknowledges financial support from the Merit Scholarship Program for Foreign Students from the Ministère de l’Éducation, du Loisir et du Sport du Québec. F. R. is grateful to the Canada Research Chairs program for partial salary support and acknowledges NSERC for an EWR Steacie Memorial Fellowship. F. R. is also grateful to the Government of China for a Chang Jiang Scholar Short Term Award and to Sichuan Province for a 1000 talent short term award. L. L.-P., P. H.-G., and D. J. acknowledge the Spanish Ministerio de Educación y Ciencia (MAT2013-47395-C4-1-R and MAT2016-75362-C3-1-R) and the EU Framework Programme (COST Action CM1403) for supporting this research. L. L.-P. also thanks the Universidad Autónoma de Madrid for the “Formación de Personal Investigador (FPI-UAM)” program. P. H.-G. is grateful to the Spanish Ministerio de Economia y Competitividad (MINECO) for the Juan de la Cierva program.
Notes and references
- J. Zhou, Q. Liu, W. Feng, Y. Sun and F. Y. Li, Chem. Rev., 2015, 115, 395–465 CrossRef CAS PubMed.
- G. Y. Chen, H. L. Qju, P. N. Prasad and X. Y. Chen, Chem. Rev., 2014, 114, 5161–5214 CrossRef CAS PubMed.
- F. Wang, D. Banerjee, Y. S. Liu, X. Y. Chen and X. Liu, Analyst, 2010, 135, 1839–1854 RSC.
- F. Auzel, Chem. Rev., 2004, 104, 139–174 CrossRef CAS PubMed.
- H. Dong, L. D. Sun and C. H. Yan, Nanoscale, 2013, 5, 5703–5714 RSC.
- J. A. Capobianco, F. Vetrone, T. D'Alesio, G. Tessari, A. Speghini and M. Bettinelli, Phys. Chem. Chem. Phys., 2000, 2, 3203–3207 RSC.
- J. Zhou, Z. Liu and F. Y. Li, Chem. Soc. Rev., 2012, 41, 1323–1349 RSC.
- E. Hemmer, A. Benayas, F. Légaré and F. Vetrone, Nanoscale Horiz., 2016, 1, 168–184 RSC.
- F. Vetrone, R. Naccache, A. Zamarron, A. J. de la Fuente, F. Sanz-Rodriguez, L. M. Maestro, E. M. Rodriguez, D. Jaque, J. G. Sole and J. A. Capobianco, ACS Nano, 2010, 4, 3254–3258 CrossRef CAS PubMed.
- D. Jaque and F. Vetrone, Nanoscale, 2012, 4, 4301–4326 RSC.
- D. Jaque, L. Martínez Maestro, B. del Rosal, P. Haro-Gonzalez, A. Benayas, J. L. Plaza, E. Martín Rodrígueza and J. García Solé, Nanoscale, 2014, 6, 9494–9530 RSC.
- L. Cheng, C. Wang, L. Z. Feng, K. Yang and Z. Liu, Chem. Rev., 2014, 14, 10869–10939 CrossRef PubMed.
- C. D. Brites, P. P. Lima, N. J. O. Silva, A. Millán, V. S. Amaral, F. Palacio and L. D. Carlos, Nanoscale, 2012, 4, 4799–4829 RSC.
- P. K. Jain, X. H. Huang, I. H. El-Sayed and M. A. El-Sayed, Acc. Chem. Res., 2008, 41, 1578–1586 CrossRef CAS PubMed.
- N. S. Abadeer and C. J. Murphy, J. Phys. Chem. C, 2016, 120, 4691–4716 CAS.
- A. M. Alkilany, L. B. Thompson, S. P. Boulos, P. N. Sisco and C. J. Murphy, Adv. Drug Delivery Rev., 2012, 64, 190–199 CrossRef CAS PubMed.
- X. H. Huang, S. Neretina and M. A. El-Sayed, Adv. Mater., 2009, 21, 4880–4910 CrossRef CAS PubMed.
- Z. J. Zhang, J. Wang and C. Y. Chen, Theranostics, 2013, 3, 223–238 CrossRef CAS PubMed.
- G. Baffou, R. Quidant and C. Girard, Appl. Phys. Lett., 2009, 94, 153109 CrossRef.
- A. M. Alkilany, P. K. Nagaria, C. R. Hexel, T. J. Shaw, C. J. Murphy and M. D. Wyatt, Small, 2009, 5, 701–708 CrossRef CAS PubMed.
- E. Locatelli, I. Monaco and M. C. Franchini, RSC Adv., 2015, 5, 21681–21699 RSC.
- Z. J. Zhang, L. M. Wang, J. Wang, X. M. Jiang, X. H. Li, Z. J. Hu, Y. H. Ji, X. C. Wu and C. Y. Chen, Adv. Mater., 2012, 24, 1418–1423 CrossRef CAS PubMed.
- S. Shen, H. Y. Tang, X. T. Zhang, J. F. Ren, Z. Q. Pang, D. G. Wang, H. L. Gao, Y. Qian, X. G. Jiang and W. L. Yang, Biomaterials, 2013, 34, 3150–3158 CrossRef CAS PubMed.
- A. J. Gormley, N. Larson, S. Sadekar, R. Robinson, A. Ray and H. Ghandehari, Nano Today, 2012, 7, 158–167 CrossRef CAS PubMed.
- W. Park, D. Lu and S. Ahn, Chem. Rev., 2015, 44, 2940–2962 RSC.
- F. Zhang, G. B. Braun, Y. F. Shi, Y. C. Zhang, X. H. Sun, N. O. Reich, D. Y. Zhao and G. Stucky, J. Am. Chem. Soc., 2010, 132, 2850–2851 CrossRef CAS PubMed.
- W. Ge, X. R. Zhang, M. Liu, Z. W. Lei, R. J. Knize and Y. L. Lu, Theranostics, 2013, 3, 282–288 CrossRef CAS PubMed.
- R. Lv, P. Yang, B. Hu, J. Xu, W. Shang and J. Tian, ACS Nano, 2017, 11, 1064–1072 CrossRef CAS PubMed.
- A. Skripka, R. Marin, A. Benayas, P. Canton, E. Hemmer and F. Vetrone, Phys. Chem. Chem. Phys., 2017, 19, 11825–11834 RSC.
- R. Lv, P. Yang, F. He, S. Gai, G. Yang, Y. Dai, Z. Hou and J. Lin, Biomaterials, 2015, 63, 115–127 CrossRef CAS PubMed.
- G. Valduga, E. Reddi, S. Garbisa and G. Jori, Int. J. Cancer, 1998, 75, 412–417 CrossRef CAS PubMed.
- C. N. Zhou, S. J. Chi, J. S. Deng, J. L. Liang, G. Jori and C. Milanesi, J. Photochem. Photobiol., B, 1996, 33, 219–223 CrossRef CAS.
- Y. Huang, E. Hemmer, F. Rosei and F. Vetrone, J. Phys. Chem. B, 2016, 120, 4992–5001 CrossRef CAS PubMed.
- B. Nikoobakht and M. A. El-Sayed, Chem. Mater., 2003, 15, 1957–1962 CrossRef CAS.
- T. Zhao, H. Wu, S. Q. Yao, Q. H. Xu and G. Q. Xu, Langmuir, 2010, 26, 14937–14942 CrossRef CAS PubMed.
- F. Denizot and R. Lang, J. Immunol. Methods, 1986, 89, 271–277 CrossRef CAS PubMed.
- H. X. Mai, Y. W. Zhang, R. Si, Z. G. Yan, L. D. Sun, L. P. You and C. H. Yan, J. Am. Chem. Soc., 2006, 128, 6426–6436 CrossRef CAS PubMed.
- X. Ye, L. Jin, H. Caglayan, J. Chen, G. Xing, C. Zheng, V. Doan-Nguyen, Y. Kang, N. Engheta, C. R. Kagan and C. B. Murray, ACS Nano, 2012, 6, 2804–2817 CrossRef CAS PubMed.
- I. Gorelikov and N. Matsuura, Nano Lett., 2008, 8, 369–373 CrossRef CAS PubMed.
- Y. Huang, F. Rosei and F. Vetrone, Nanoscale, 2015, 7, 5178–5185 RSC.
- M. Saboktakin, X. C. Ye, U. K. Chettiar, N. Engheta, C. B. Murray and C. R. Kagan, ACS Nano, 2013, 7, 7186–7192 CrossRef CAS PubMed.
- M. Saboktakin, X. Ye, S. J. Oh, S. H. Hong, A. T. Fafarman, U. K. Chettiar, N. Engheta, C. B. Murray and C. R. Kagan, ACS Nano, 2012, 6, 8758–8766 CrossRef CAS PubMed.
- E. Frohlich, Int. J. Nanomed., 2012, 7, 5577–5591 CrossRef PubMed.
- S. Bhattacharjee, L. H. J. de Haan, N. M. Evers, X. Jiang, A. T. M. Marcelis, H. Zuilhof, I. M. C. M. Rietjens and G. M. Alink, Part. Fibre Toxicol., 2010, 7, 25 CrossRef PubMed.
- F. Vetrone, R. Naccache, A. J. de la Fuente, F. Sanz-Rodriguez, A. Blazquez-Castro, E. M. Rodriguez, D. Jaque, J. G. Sole and J. A. Capobianco, Nanoscale, 2010, 2, 495–498 RSC.
Footnote |
† Electronic supplementary information (ESI) available: Supplementary figures. See DOI: 10.1039/c7nr05499h |
|
This journal is © The Royal Society of Chemistry 2018 |
Click here to see how this site uses Cookies. View our privacy policy here.