DOI:
10.1039/C7NJ03615A
(Paper)
New J. Chem., 2018,
42, 613-618
A one-step synthesized acridine-based fluorescent chemosensor for selective detection of copper(II) ions and living cell imaging†
Received
21st September 2017
, Accepted 17th November 2017
First published on 20th November 2017
Abstract
Herein, the easily approachable N,N′-(acridine-3,6-diyl)dipicolinamide (ACC) prepared by only one step was evaluated as an efficient and selective chemosensor for the selective detection of copper ions (Cu2+). The fluorescence sensing behavior of ACC toward metal cations was investigated in an HEPES buffer aqueous solution. The fluorescence emission was significantly quenched in the presence of Cu2+ cations, whereas other metal cations showed a low interference. The limit of detection was found to be 1.2 × 10−7 M. The recognizing mechanism of ACC towards Cu2+ has been investigated by 1H NMR, HR-MS analysis, and density functional theory (DFT) calculations. Moreover, this sensor was verified to have low cytotoxicity and good imaging characteristics for Cu2+ in living cells; this suggested that ACC was a potential probe for the detection of Cu2+in vivo.
Introduction
Copper(II) ion (Cu2+), as the third abundant essential transition metal ion in human body, plays essential roles in various fundamental physiological processes in organisms.1,2 The imbalance of cellular Cu2+ homeostasis has been reported to result in many serious diseases such as Alzheimer's disease,3 Parkinson disease,4 Prion disease,5,6 Huntington disease,7 and Menkes and Wilson disease.8,9 Moreover, recent studies have indicated that it can regulate tumor cell growth by inducing oncogenic BRAF mutations.10,11 Therefore, detection of the trace amounts of Cu2+ in biological systems has attracted widespread attention.
The fluorescence sensing strategy has been proven to be the most important approach for the detection and imaging of relevant biological molecules due to its high sensitivity, easy operation, visual simplicity, instantaneous response, and real-time detection. In the past few decades, a vast majority of fluorescent chemosensors for Cu2+ detection displaying turn-off response have been reported such as quinolines,12–20 curcumins,21–23 calixarenes,24–33 and rhodamines.34–46 These structurally intricate chemosensors needed tailor-made, complicated synthetic methods. Acridine derivatives have indicated extraordinary optical properties, and studies on their application for cancer therapy have been reported,47–51 but they rarely include metal ion detections.52–57
In the recent past, a number of studies have described the advances of fluorescein probes regarding the synthesis methods, optical properties, possible mechanisms, and applications.58 Herein, we have developed an easily approachable acridine derivative, which has been synthesized from very simple materials in only one-step. The newly synthesized acridine proved to be a sensitive and selective sensor for Cu2+ detection both under simulated physiological conditions (10 mM HEPES buffer, pH 7.0) and in cells. The binding model of ACC and Cu2+ was investigated by 1H NMR, HRMS analysis, and DFT calculations. Moreover, the proposed mechanism by which Cu2+ quenched the fluorescence of ACC was the chelation-enhanced fluorescence quenching (CHEQ) effect.59–62 Remarkably, this sensor showed very low cytotoxicity, which made its application in vivo possible.
Experimental
Materials and general methods
All chemicals and reagents, such as picolinic acid (98%, Energy), proflavine hemisulfate (97%, Energy), 2-(7-aza-1H-benzotriazole-1-yl)-1,1,3,3-tetramethyluronium hexafluorophosphate (HATU, 99.5%, Energy), ethyldiisopropylamine (DIEA, 99.5%, Energy), and 4-(2-hydroxyethyl)-1-piperazineethanesulfonic acid (HEPES, 99%, Alfa) were obtained commercially and used without further purification. Solutions of the metal ions were prepared from MgCl2·6H2O, MnCl2·4H2O (Fuchen Chemical), NiCl2·6H2O, CoCl2·6H2O (Sigma aldrich), KCl, NaCl (Sangan Biotech), HgNH2Cl, PbCl2, CrCl3·6H2O, ZnCl2·6H2O, CaCl2·4H2O, CuCl2·3H2O, BaCl2, AlCl3·9H2O, FeCl3·9H2O, and SnCl2 (Aladin). Anions solutions (0.001 M) of sodium salts (NO2−, HPO42−, S2O52−, ClO2−, Cl−, OH−, I−, SO42−, H2PO4−, and S2−) were purchased from Energy. All the organic solvents were purchased from Adamas. All solutions were prepared using ultrapurified water (18.4 MΩ cm) deionized by a Milli-QSP reagent water system (Millipore). Nuclear magnetic resonance (NMR) spectra were obtained using a Bruker Ultrashield TM 400 PLUS spectrometer. UV-vis spectroscopy spectra were acquired using the SCINCOS-4100 UV/vis spectrophotometer. Fluorescence spectra were obtained using a Thermo Scientific Lumina spectrofluorometer. Mass spectrum (MS) was obtained using a Waters Q-TOF premier mass spectrometer. Melting points were determined using a Thomas-Hoover capillary melting point apparatus. Microplate Reader was obtained from TECAN Infinite M1000PRO.
Synthesis of N,N′-(acridine-3,6-diyl)dipicolinamide chemosensor (ACC)
ACC was prepared in one step from the low-cost commercial proflavine hemisulfate, as shown in Scheme 1. A condensing reagent was used to perform the amidation reaction. Herein, 0.260 g (0.5 mmol) proflavine hemisulfate, 0.150 g (1.2 mmol) picolinic acid, 0.165 g (1.25 mmol) DIEA, and 10 mL anhydrous N,N-dimethylformamide were added to a 25 mL necked flask equipped with a magnetic stirring bar. After the solution was stirred for 30 min at room temperature, 0.762 g (2.0 mmol) HATU was added, and the mixture was stirred at room temperature overnight. Then, 15 mL water was added to terminate the reaction, and a precipitate was formed. The filtered residue was redissolved and purified by silica gel chromatography (VDCM
:
VMeOH = 40
:
1), a pale yellow solid product was obtained (0.11 g, yield: 50%, m.p. 236–237 °C). 1H NMR (400 MHz, DMSO-d6) δ 11.18 (s, 2H), 9.16 (s, 1H), 8.93 (s, 2H), 8.75–8.74 (m, 2H), 8.18–8.13 (m, 4H), 8.06 (dt, J = 1.6 Hz, J = 7.6 Hz, 2H), 8.01 (dd, J = 1.6 Hz, J = 9.0 Hz, 2H), 7.70–7.67 (m, 2H). 13C NMR (100 MHz, DMSO-d6) δ 164.06, 149.63, 149.04, 138.72, 130.53, 127.90, 123.29, 122.73, 122.22. HRMS calculated for C25H18N5O2 [ACC + H]+: 420.1455. Found 420.1445.
 |
| Scheme 1 Schematic for the synthesis of ACC. | |
Cell cytotoxicity assay
The inhibitory effect of ACC on the growth of HeLa tumor cells was evaluated by 3-(4,5-dimethylthiazol-2-yl)-2,5-diphenyltetrazolium bromide (MTT) assays. HeLa cells cultured in DMEM with 10% fetal bovine serum (FBS) were seeded at a density of about 1 × 105 cells per well. After the cells were incubated in 5% CO2 at 37 °C for 16 h, ACC with the final concentration of 125 μM, 62.5 μM, 31.25 μM, 15.63 μM, 7.81 μM, and 3.91 μM was added to the culture medium, and the cells were cultured for another 48 h.
Then, the freshly prepared MTT was added to each well with a final concentration of 0.5 mg mL−1, and the plates were incubated at 37 °C for 4 h. Subsequently, the supernatant was removed, and 100 μL of DMSO was added to each well. The absorbance of the mixed solution was measured at 490 nm wavelength using a microplate reader. The MTT assay was conducted at least three times.
Confocal imaging
Stock solutions of ACC (20 μM) in DMSO and Cu2+ (20 μM) in the HEPES buffer (10 mM, pH 7.0) were pre-prepared. HeLa cells were adherent cultured in confocal dishes (35 mm dish, 20 dimension) for 18 h at 37 °C first and then incubated with 20 μM (final concentration) ACC for 2 h. Then, Cu2+ cation (1 equiv.) was added to the dish and then incubated for another 30 min. The cells were washed three times with sterile PBS buffer before being imaged by a confocal laser scanning microscope.
Results and discussion
Spectroscopic properties indicating the sensitivity and selectivity of ACC for Cu2+
The UV/vis absorption spectra of ACC obtained in the presence of several transition metal ions are shown in Fig. 1a. The responses of the ACC solution to a variety of cations, including Na+, K+, Cu2+, Mg2+, Ca2+, Mn2+, Co2+, Ba2+, Ni2+, Zn2+, Hg2+, Pb2+, Cd2+, Al3+, Fe3+, and Cr3+, were investigated in the HEPES buffer solution. After the addition of different metal ions, ACC showed no significant changes in its absorption spectra band except for Cu2+. The addition of Cu2+ caused the absorption wavelength of ACC to shift to 425 nm from 400 nm with the increasing intensity; this might result from the chelation of Cu2+ and ACC. To further explore the selectivity of ACC towards metal ions, fluorescence spectra were also obtained under the same conditions. The results, as shown in Fig. 1b, indicated that Cu2+ was the only cation that led to fluorescence quenching. The efficient quenching of Cu2+ suggested that ACC showed a specific response to Cu2+ probably due to the chelation-enhanced fluorescence quenching (CHEQ) effect. The reason for fluorescence quenching was the formation of a non-fluorescent complex due to the CHEQ behavior of ACC in the presence of Cu2+; this behavior was attributed to be due to the paramagnetic effect from spin–orbit coupling of Cu2+.59–62
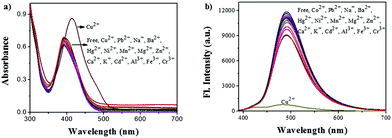 |
| Fig. 1 (a) UV-vis absorption spectra of ACC (40 μM, HEPES buffer, pH 7.0) in the presence of Cu2+ and other cations (100 μM) including Na+, K+, Mg2+, Ca2+, Mn2+, Co2+, Ba2+, Ni2+, Zn2+, Hg2+, Pb2+, Cd2+, Al3+, Fe3+, and Cr3+. (b) Fluorescence emission spectra of ACC (20 μM) with the addition of various other common metal cations (100 μM) in an HEPES (10 mM, pH 7.0) aqueous solution. | |
A fluorescence titration experiment of ACC (10 μM) with Cu2+ (0–100 μM) in HEPES buffer solutions (10 mM, pH 7.0) was also carried out. As shown in Fig. 2a, ACC exhibited strong fluorescence emission at 491 nm. Upon incremental addition of Cu2+, the fluorescence emission was decreased gradually. The plot of the fluorescence intensity versus Cu2+ (1–16 μM) exhibited a good linear relationship with the R2 value of 0.9911. (Fig. 2b). The binding association constant (Ka) for ACC–Cu2+ was determined by the Benesi–Hildebrand equation63,64 and calculated to be 1.6 × 105 L mol−1. The limit of detection (LOD) was found to be 0.12 μM by the reported equation LOD = 3k/s,65 where s is the standard deviation of the blank measurement, and k is the slope between the intensity versus Cu2+ concentration. The LOD of ACC was much lower than the recommended maximum contaminant level (MCL) for Cu2+ in drinking water (31 μM), as defined by the World Health Organization (WHO).66,67 The low detection limit and good linear correlation indicated that ACC was able to selectively and qualitatively detect Cu2+ in the buffer system as well.
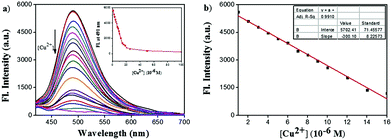 |
| Fig. 2 (a) Fluorescence spectra of ACC (10 μM) obtained with the addition of various concentrations of CuCl2 [0, 1, 2, 3, 4, 5, 6, 7, 8, 9, 10, 12, 14, 16, 18, 20, 25, 50, and 100 μM] in an HEPES (10 mM, pH 7.0) aqueous solution with an excitation at 370 nm. (b) Changes in the fluorescence intensity of ACC at 491 nm upon the addition of Cu2+ (1–16 μM). The line shown is a line-fitted curve. | |
To estimate the specificity of ACC, competitive experiments were carried out (Fig. 3). The fluorescence of ACC (10 μM) in the HEPES buffer solution with or without selected metal ions (50 μM) (Cu2+, K+, Na+, Zn2+, Hg2+, Ca2+, Mg2+, Mn2+, Co2+, Ba2+, Pb2+, Ni2+, Cr3+, Al3+, and Fe3+) was first evaluated, and only Cu2+ induced obvious fluorescence changes of ACC at 491 nm. As shown in Fig. 3a, the introduction of Cu2+ into the ACC metal ion solution could efficiently induce the decrease in fluorescence intensity. When ACC (10 μM) was treated with 50 μM of Cu2+ and then other competitive metal ions (50 μM) were added, very low interference in the fluorescence spectra was observed (Fig. 3b). These results indicated that this fluorescence quenching was not interfered by other metal ions; this revealed that ACC possessed high selectivity of the system for Cu2+ cation over other metal ions.
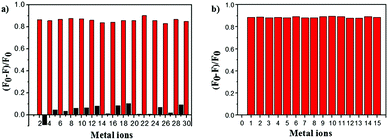 |
| Fig. 3 (a) The fluorescence intensity at 491 nm of ACC (10 μM) with other metal ions (50 μM) in the HEPES buffer (10 mM, pH 7.0) and then with addition of 50 μM of Cu2+. 1, free; 2, free + Cu2+; 3, K+; 4, K+ + Cu2+; 5, Na+; 6, Na+ + Cu2+; 7, Zn2+; 8, Zn2+ + Cu2+; 9, Hg2+; 10, Hg2+ + Cu2+; 11, Ca2+; 12, Ca2+ + Cu2+; 13, Mg2+; 14, Mg2+ + Cu2+; 15, Mn2+; 16, Mn2+ + Cu2+; 17, Co2+; 18, Co2+ + Cu2+; 19, Ba2+; 20, Ba2+ + Cu2+; 21, Pb2+; 22, Pb2+ + Cu2+; 23, Ni2+; 24, Ni2+ + Cu2+; 25, Cr3+; 26, Cr3+ + Cu2+; 27, Al3+; 28, Al3+ + Cu2+; and 29, Fe3+; 30, Fe3+ + Cu2+; (b) the fluorescence intensity at 491 nm of ACC (10 μM) with the addition of 50 μM of Cu2+ and then addition of other metal ions (50 μM) in the HEPES buffer (10 mM, pH 7.0). 0, free; 1, free + Cu2+; 2, Cu2+ + K+; 3, Cu2+ + Na+; 4, Cu2+ + Zn2+; 5, Cu2+ + Hg2+; 6, Cu2+ + Ca2+; 7, Cu2+ + Mg2+; 8, Cu2+ + Mn2+; 9, Cu2+ + Co2+; 10, Cu2+ + Ba2+; 11, Cu2+ + Pb2+; 12, Cu2+ + Ni2+; 13, Cu2+ + Cr3+; 14, Cu2+ + Al3+; and 15, Cu2+ + Fe3+. | |
To evaluate the effect of anions on the detection efficiency, we investigated the binding behavior of the metal-based chemosensor (ACC–Cu2+ complex) towards various anions including NO2−, HPO42−, S2O52−, ClO2−, Cl−, OH−, I−, SO42−, H2PO4−, and S2− (Fig. 4a). The results showed that these anions except S2− had barely any interference in the detection of Cu2+, and S2− recovered the fluorescence intensity of the ACC–Cu2+ system, which was consistent with previously reported studies.68,69 A competitive experiment was also carried out to estimate the specificity of ACC towards Cu2+ in the presence of other anions. As shown in Fig. 4b, very low interference in the fluorescence emission was observed in the presence of other anions, but S2− would impact its fluorescence intensity. In this strategy, sulfide ion can coordinate with copper ions to form more stable copper sulfide precipitates, followed by the release of the free ACC.70
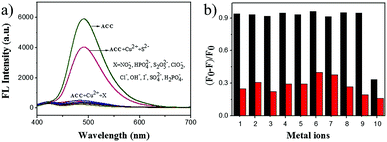 |
| Fig. 4 (a) Fluorescence spectra obtained for ACC (10 μM) and Cu2+ (50 μM) upon the addition of various anions (50 μM), and (b) the fluorescence intensity at 491 nm for ACC (10 μM) upon the addition of various anions (1, NO2−; 2, HPO42−; 3, S2O52−; 4, ClO2−; 5, Cl−; 6, OH−; 7, I−; 8, SO42−; 9, H2PO4−; 10, S2−;) and then addition of Cu2+ (50 μM) in the HEPES buffer (10 mM, pH 7.0) aqueous solution. | |
The effect of pH on the detection of Cu2+ by ACC was evaluated as well, which proved to be within an appropriate range for biological application. The spectra showed that in the HEPES buffer solution, the suitable pH range for Cu2+ determination was pH 5.0–8.0, which was the most common pH environment in nature and daily life. Only when the pH was lower than 4.0 or higher than 9.0, ACC lot its detection for Cu2+ at 491 nm, as shown in Fig. S4 (ESI†). The detrimental effect of the acidic condition on ACC might be partially due to the basic pyridine group of ACC, the lone pair electron of which was easily protonated into salt under acidic conditions. On the other hand, under basic conditions (pH > 8), the ACC/Cu2+ complex solution led to a gradual reduction in fluorescence that might form a more stable Cu(II) hydroxide complex in these alkaline mixed solutions.71,72
Possible sensing mechanism of ACC with Cu2+
To investigate the binding model of ACC and Cu2+, 1H NMR titration experiments were carried out in DMSO-d6 (Fig. 5). The cation-induced chemical shift changes were measured with the variation of the mole ratio (Cu2+/ACC, 0–3 equiv.). Cu2+ is a paramagnetic cation and can affect the proton signals; this leads to the tardily disappeared split of the peaks. With the addition of Cu2+, all the chemical shift of the aromatic hydrogens (ArH) slightly moved downfield. Obviously, the H signal of the amide (–CONH–) became broader and weaker. These results indicated that the nitrogen of pyridine and nitrogen of the amide might have participated in the binding with Cu2+, which was in accordance with previous reports.73,74
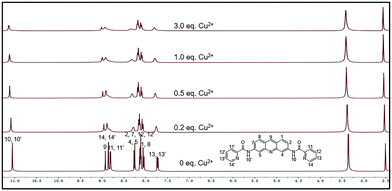 |
| Fig. 5
1H NMR (400 M) titration analysis of (1) ACC, (2) ACC + 0.2 equiv. Cu2+, (3) ACC + 0.5 equiv. Cu2+, (4) ACC + 1.0 equiv. Cu2+, and (5) ACC + 3.0 equiv. Cu2+ in DMSO-d6. | |
To figure out the binding model of Cu2+ and ACC, ESI mass spectra of the ACC–Cu2+ complexes (Fig. 6) were obtained and analyzed. The cluster peaks at 420.1440 and 481.0604 corresponded to [ACC + H]+ (calcd = 420.1455) and [2ACC + 2Cu2+]2+/2 (calcd = 481.0595), respectively. This results further suggested that the binding stoichiometry of ACC–Cu2+ was 2
:
2.
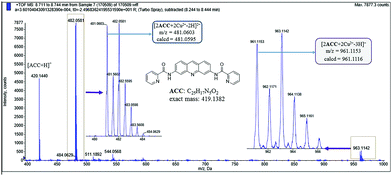 |
| Fig. 6 ESI mass spectra of ACC in the presence of Cu(NO3)2 (10 equiv.), indicating the formation of a 2 : 2 ACC–Cu2+ complex. | |
To further investigate the mechanism of the fluorescence quenching by the energy or charge transfer model, density functional theory (DFT) calculations were carried out using a Gaussian 09 program.75–77 The optimized structures of ACC and ACC–Cu2+ complex are shown in Fig. 7. The band gap between HOMO (−0.013 eV) and LUMO (0.048 eV) of ACC was calculated as 0.061 eV. On the other hand, the band gap between HOMO (−0.311 eV) and LUMO (0.057 eV) of the ACC–Cu2+ complex had increased to 0.367 eV as compared to that of ACC. From this calculation, the energy increase in the HOMO level was more remarkable than that in the LUMO level; these results indicated that LUMO was more stabilized than HOMO. The energy of the HOMO of ACC–Cu2+ was more stabilized than that of ACC, but the stability of the LUMO of ACC–Cu2+ and ACC was almost approximate. Therefore, the conversion of ACC to ACC–Cu2+ becomes easier by the broadening the energy gap between HOMO and LUMO due to the formation of a stable ACC–Cu2+ complex, which is similar to that reported by in Fang and Kim.75,77
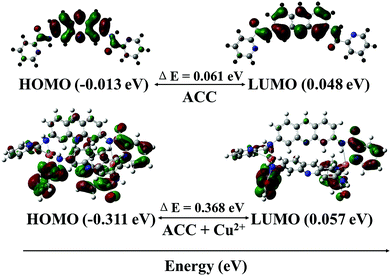 |
| Fig. 7 Frontier molecular orbital profiles of ACC–Cu2+ based on DFT (B3LYP/6-31G*) calculations. | |
Potential applicability of ACC in cell imaging
Our sensor exhibited extraordinary selectivity and efficiency for Cu2+ detection in both water and buffer system. To further evaluate whether it can be used in biological samples, the cytotoxicity assay is initiated (Fig. S5, ESI†). Speculating that the viability of the untreated HeLa cells was 100%, the viability of the cells incubated with a series of different concentrations (0–500 μM) of ACC for 48 h at 37 °C was found to be higher than 90%; this suggested that ACC had low cytotoxicity and could serve as a potential probe for living cell imaging. Encouraged by the abovementioned results, confocal fluorescence microscopy was employed to explore the detection ability of ACC for Cu2+. HeLa cells were incubated with 10 μM ACC at 37 °C for 2 h and then washed with 1× PBS buffer three times to remove excess ACC. As shown in Fig. 8a–c, blue intracellular fluorescence could be observed with an excitation of 405 nm blue light. Moreover, we could preliminarily decide that the probe displayed its specificity to localize in cytoplasm (Fig. 8b). In contrast, HeLa cells were cultured with 10 μM Cu2+ cations (Fig. 8e). The results obtained suggested that ACC could enter the cells and could be applied for the detection of Cu2+ in living cells.
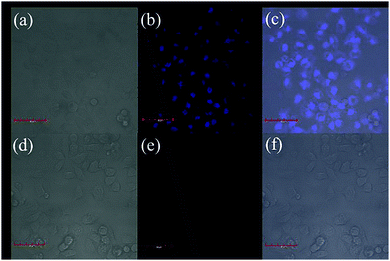 |
| Fig. 8 Confocal fluorescence images of the living HeLa cells. (a–c) Confocal microscopy images of the HeLa cells stained with 10 μM of ACC; (d–f) confocal microscopy images of the HeLa cells stained with 10 μM of ACC and Cu2+. | |
Conclusions
In conclusion, we have described the synthesis, properties, and cellular application of the chemosensor ACC. This acridine derivative can selectively and sensitively detect Cu2+ over other common metal ions in the HEPES buffer, leading to obvious fluorescence quenching. The behaviors of ACC, such as engaging in a 100% aqueous medium at physiological pH, displaying a low detection limit, indicating a higher selectivity towards Cu2+, and being applied to cell imaging, indicate that this accessible, low budget, and practical chemosensor has great potential for the detection of Cu2+ in living biosystems.
Conflicts of interest
There are no conflicts of interest to declare.
Acknowledgements
We gratefully acknowledge the National Natural Science Foundation of China (No. 21402105) and Shenzhen Municipal government SZSITIC (No. JCYJ20160301153753269, JCYJ20160301153959476, JCYJ20160324163734374).
References
- J. A. Cowan, Biochimie, 1997, 80, 347–348 Search PubMed.
- W. H. Bannister, Biochem. Mol. Biol. Educ., 1992, 20, 62–63 Search PubMed.
- K. J. Barnham, C. L. Masters and A. I. Bush, Nat. Rev. Drug Discovery, 2004, 3, 205–214 CrossRef CAS PubMed.
- K. M. Davies, J. F. Mercer, N. Chen and K. L. Double, Clin. Sci., 2016, 130, 565–574 CrossRef PubMed.
- A. J. Mcdonald, J. P. Dibble, E. G. B. Evans and G. L. Millhauser, J. Biol. Chem., 2014, 289, 803–813 CrossRef CAS PubMed.
- D. R. Brown and H. Kozlowski, Dalton Trans., 2004, 1907–1917 RSC.
- G. Xiao, Q. Fan, X. Wang and B. Zhou, Proc. Natl. Acad. Sci. U. S. A., 2013, 110, 14995–15000 CrossRef CAS PubMed.
- D. J. Waggoner, T. B. Bartnikas and J. D. Gitlin, Neurobiol. Dis., 1999, 6, 221–230 CrossRef CAS PubMed.
- B. Levinson, J. Gitschier, C. Vulpe, S. Whitney, S. Yang and S. Packman, Nat. Genet., 1993, 3, 6 CrossRef CAS PubMed.
- D. C. Brady, M. S. Crowe, M. L. Turski, G. A. Hobbs, X. Yao, A. Chaikuad, S. Knapp, K. Xiao, S. L. Campbell and D. J. Thiele, Nature, 2014, 509, 492–496 CrossRef CAS PubMed.
- S. Ishida, P. Andreux, C. Poitryyamate, J. Auwerx and D. Hanahan, Proc. Natl. Acad. Sci. U. S. A., 2013, 110, 19507–19512 CrossRef CAS PubMed.
- J. Yang, Z.-L. Yuan, G.-Q. Yu, S.-L. He, Q.-H. Hu, Q. Wu, B. Jiang and G. Wei, J. Fluoresc., 2016, 26, 43–51 CrossRef CAS PubMed.
- L.-Q. Li, Synth. React. Inorg., Met.-Org., Nano-Met. Chem., 2016, 46, 1854–1856 CrossRef CAS.
- H.-J. Kim, J. Lumin., 2016, 176, 335–341 CrossRef CAS.
- Y. Zhu, H. Li, G. Yan, B. Shi, Y. Zhang, Q. Lin, H. Yao and T. Wei, RSC Adv., 2015, 5, 49953–49957 RSC.
- C. Zhou, Y. Song, N. Xiao, Y. Li and J. Xu, J. Fluoresc., 2014, 24, 1331–1336 CrossRef CAS PubMed.
- X. Yao, Y.-Y. Guo, J.-X. Ru, C. Xu, Y.-M. Liu, W.-W. Qin, G.-L. Zhang, X.-L. Tang and W.-S. Liu, Sens. Actuators, B, 2014, 198, 20–25 CrossRef CAS.
- G. J. Park, I. H. Hwang, E. J. Song, H. Kim and C. Kim, Tetrahedron, 2014, 70, 2822–2828 CrossRef CAS.
- L. Zhang, J. Sun, S. Liu, X. Cui, W. Li and J. Fang, Inorg. Chem. Commun., 2013, 35, 311–314 CrossRef CAS.
- C. Gao, X. Liu, X. Jin, J. Wu, Y. Xie, W. Liu, X. Yao and Y. Tang, Sens. Actuators, B, 2013, 185, 125–131 CrossRef CAS.
- Q. Zhang, C. Zhang, Z. Li, J. Ge, C. Li, C. Dong and S. Shuang, RSC Adv., 2015, 5, 95054–95060 RSC.
- K. S. Park, Y. Seo, M. K. Kim, K. Kim, Y. K. Kim, H. Choo and Y. Chong, Org. Biomol. Chem., 2015, 13, 11194–11199 CAS.
- M. Caselli, E. Ferrari, C. Imbriano, F. Pignedoli, M. Saladini and G. Ponterini, J. Photochem. Photobiol., A, 2010, 210, 115–124 CrossRef CAS.
- M. Nemati, R. Hosseinzadeh, R. Zadmard and M. Mohadjerani, Sens. Actuators, B, 2017, 241, 690–697 CrossRef CAS.
- D. H. Xie, X. J. Wang, C. Sun and J. Han, Tetrahedron Lett., 2016, 57, 5834–5836 CrossRef CAS.
- B. Khan, M. R. Shah, D. Ahmed, M. Rabnawaz, I. Anis, S. Afridi, T. Makhmoor and M. N. Tahir, J. Hazard. Mater., 2016, 309, 97–106 CrossRef CAS PubMed.
- S. Erdemir, B. Tabakci and M. Tabakci, Sens. Actuators, B, 2016, 228, 109–116 CrossRef CAS.
- S. Elçin, H. Deligöz, A. A. Bhatti, M. Oguz, S. Karakurt and M. Yilmaz, Sens. Actuators, B, 2016, 234, 345–352 CrossRef.
- N. Kumar, Q. X. Pham, A. Depauw, M. Hémadi, N. T. Haduong and J. P. Lefevre, New J. Chem., 2017, 41, 7162–7170 RSC.
- I. Leray and B. Valeur, Eur. J. Inorg. Chem., 2009, 3525–3535 CrossRef CAS.
- Z. Xu, S. Kim, H. N. Kim, S. J. Han, C. Lee, J. S. Kim, X. Qian and J. Yoon, Tetrahedron Lett., 2007, 48, 9151–9154 CrossRef CAS.
- J. S. Kim and D. T. Quang, Chem. Rev., 2007, 107, 3780–3799 CrossRef CAS PubMed.
- M. Panchal, M. Athar, P. C. Jha, A. Kongor, V. Mehta and V. Jain, New J. Chem., 2017, 41, 5125–5132 RSC.
- D. Xue, C. Zheng, S. Qu, G. Liao, C. Fan, G. Liu and S. Pu, Luminescence, 2017, 32, 652–660 CrossRef CAS PubMed.
- Y. Wang, H. Q. Chang, W. N. Wu, X. J. Mao, X. L. Zhao, Y. Yang, Z. Q. Xu, Z. H. Xu and L. Jia, J. Photochem. Photobiol., A, 2017, 335, 10–16 CrossRef CAS.
- V. F. Traven, N. A. Pozharskaya, N. P. Solovjova, R. A. Novikov, M. G. Medvedev, V. V. Chernyshev, S. M. Dolotov and I. V. Ivanov, Dyes Pigm., 2017, 136, 612–618 CrossRef CAS.
- H. Y. Lee, K. M. K. Swamy, Y. J. Ji, G. Kim and J. Yoon, Sens. Actuators, B, 2013, 182, 530–537 CrossRef CAS.
- L. Lv and Q. Diao, Spectrochim. Acta, Part A, 2017, 179, 221–226 CrossRef CAS PubMed.
- T. Li, Y. Yang, P. Zhou, Z. Peng, J. Qin, L. Liu and Y. Wang, Polyhedron, 2017, 128, 154–159 CrossRef CAS.
- K. Huang, Y. Yue, X. Jiao, C. Liu, Q. Wang, S. He, L. Zhao and X. Zeng, Dyes Pigm., 2017, 143, 379–386 CrossRef CAS.
- Y. Yang, C. Y. Gao, N. Zhang and D. Dong, Sens. Actuators, B, 2016, 222, 741–746 CrossRef CAS.
- X. F. Zhang and Y. K. Zhang, Dyes Pigm., 2015, 120, 265–270 CrossRef CAS.
- Z. Xu, L. Zhang, R. Guo, T. Xiang, C. Wu, Z. Zheng and F. Yang, Sens. Actuators, B, 2011, 156, 546–552 CrossRef CAS.
- C. Yu, J. Zhang, R. Wang and L. Chen, Org. Biomol. Chem., 2010, 8, 640–647 Search PubMed.
- Y. Zhou, F. Wang, Y. Kim, S. J. Kim and J. Yoon, Org. Lett., 2009, 11, 4442–4445 CrossRef CAS PubMed.
- M. Wang, F. Yan, Y. Zou, L. Zou, L. Chen, N. Yang and X. Zhou, Sens. Actuators, B, 2014, 192, 512–521 CrossRef CAS.
- Z. Yuan, S. Chen, C. Chen, J. Chen, C. Chen, Q. Dai, C. Gao and Y. Jiang, Eur. J. Med. Chem., 2017, 138, 1135–1146 CrossRef CAS PubMed.
- D. Li, Z. Yuan, S. Chen, C. Zhang, L. Song, C. Gao, Y. Chen, C. Tan and Y. Jiang, Bioorg. Med. Chem., 2017, 25, 3437–3446 CrossRef CAS PubMed.
- Z. Cui, S. Chen, Y. Wang, C. Gao, Y. Chen, C. Tan and Y. Jiang, Eur. J. Med. Chem., 2017, 136, 372–381 CrossRef CAS PubMed.
- W. Zhang, B. Zhang, W. Zhang, T. Yang, N. Wang, C. Gao, C. Tan, H. Liu and Y. Jiang, Eur. J. Med. Chem., 2016, 116, 59–70 CrossRef CAS PubMed.
- Z. Cui, X. Li, L. Li, B. Zhang, C. Gao, Y. Chen, C. Tan, H. Liu, W. Xie, T. Yang and Y. Jiang, Bioorg. Med. Chem., 2016, 24, 261–269 CrossRef CAS PubMed.
- F. Luzardo, N. Alvarez, C. Kremer, A. S. S. de. Camargo and J. S. Gancheff, Spectrochim. Acta, Part A, 2017, 183, 45–52 CrossRef CAS PubMed.
- Y. Dai, K. Xu, Q. Li, C. Wang, X. Liu and P. Wang, Spectrochim. Acta, Part A, 2016, 157, 1–5 CrossRef CAS PubMed.
- S. Chemate and N. Sekar, RSC Adv., 2015, 5, 27282–27289 RSC.
- X.-J. Jiang, Y. Fu, L.-H. Xu, H.-L. Lu, S.-Q. Zang, M.-S. Tang and T. C. W. Mak, Sens. Actuators, B, 2014, 202, 388–394 CrossRef CAS.
- Y. Wang, L. L. Shi, H. H. S. Sun, Z. B. Shang, J. B. Chao and W. J. Jin, J. Lumin., 2013, 139, 16–21 CrossRef CAS.
- C. Bazzicalupi, A. Bencini, I. Matera, S. Puccioni and B. Valtancoli, Inorg. Chim. Acta, 2012, 381, 162–169 CrossRef CAS.
- F. Yan, K. Fan, Z. Bai, R. Zhang, F. Zu, J. Xu and X. Li, TrAC, Trends Anal. Chem., 2017, 97, 15–35 CrossRef CAS.
- M. Qin, D. Jin, W. Che, Y. Jiang, L. Zhang, D. Zhu and Z. Su, Inorg. Chem. Commun., 2017, 75, 25–28 CrossRef CAS.
- Y. Li, M. Sun, K. Zhang, Y. Yan, K. Lei, L. Wu, H. Yu and S. Wang, Sens. Actuators, B, 2017, 243, 36–42 CrossRef CAS.
- Y. S. Lipatov, L. F. Kosyanchuk, N. V. Kozak, N. V. Yarovaya and G. Y. Menzheres, Polym. Sci., Ser. A, 2006, 48, 388–394 CrossRef.
- J. P. Holland, P. J. Barnard, S. R. Bayly, H. M. Betts, G. C. Churchill, J. R. Dilworth, R. Edge, J. C. Green and R. Hueting, Eur. J. Inorg. Chem., 2008, 1985–1993 CrossRef CAS.
- Y. Zhang, M. Li, Q. Niu, P. Gao, G. Zhang, C. Dong and S. Shuang, Talanta, 2017, 171, 143–151 CrossRef CAS PubMed.
- H. A. Benesi and J. H. Hildebrand, J. Am. Chem. Soc., 1949, 71, 2703–2707 CrossRef CAS.
- C. Chen, H. Liu, B. Zhang, Y. Wang, K. Cai, Y. Tan, C. Gao, H. Liu, C. Tan and Y. Jiang, Tetrahedron, 2016, 72, 3980–3985 CrossRef CAS.
- H. Liu, F. Wu, B. Zhang, C. Tan, Y. Chen, G. Hao, Y. Tan and Y. Jiang, RSC Adv., 2016, 6, 77508–77514 RSC.
- WHO, 2009.
- S. Palanisamy, L.-Y. Lee, Y.-L. Wang, Y.-J. Chen, C.-Y. Chen and Y.-M. Wang, Talanta, 2016, 147, 445–452 CrossRef CAS PubMed.
- M.-Q. Wang, K. Li, J.-T. Hou, M.-Y. Wu, Z. Huang and X.-Q. Yu, J. Org. Chem., 2012, 77, 8350–8354 CrossRef CAS PubMed.
- L. J. Tang, M. J. Cai, Z. L. Huang, K. L. Zhong, S. H. Hou, Y. J. Bian and R. Nandhakumar, Sens. Actuators, B, 2013, 185, 188–194 CrossRef CAS.
- L. M. Berreau, S. Mahapatra, J. A. Halfen, V. G. Young and W. B. Tolman, Inorg. Chem., 1996, 35, 6339–6342 CrossRef CAS.
- J. J. Lee, Y. W. Choi, G. R. You, S. Y. Lee and C. Kim, Dalton Trans., 2015, 44, 13305–13314 RSC.
- H. Seo, M. An, B. Y. Kim, J. H. Choi and A. Helal, Tetrahedron, 2017, 73, 4684–4691 CrossRef CAS.
- X. Zhu, Q. Lin, J. C. Lou, T. T. Lu, Y. M. Zhang and T. B. Wei, New J. Chem., 2015, 39, 7206–7210 RSC.
- W. Cao, X. J. Zheng, C. Feng and L. P. Jin, Dalton Trans., 2015, 44, 5191–5196 RSC.
- J. M. Rowland, M. M. Olmstead and P. K. Mascharak, Inorg. Chem., 2000, 39, 5326–5332 CrossRef CAS PubMed.
- H. S. Jung, P. S. Kwon, J. W. Lee, J. I. Kim and C. S. Hong, J. Am. Chem. Soc., 2009, 131, 2008–2012 CrossRef CAS PubMed.
Footnotes |
† Electronic supplementary information (ESI) available. See DOI: 10.1039/c7nj03615a |
‡ The two authors contributed equally to the work. |
|
This journal is © The Royal Society of Chemistry and the Centre National de la Recherche Scientifique 2018 |
Click here to see how this site uses Cookies. View our privacy policy here.