DOI:
10.1039/C7NJ03562D
(Paper)
New J. Chem., 2018,
42, 1092-1098
Copper nanoparticles grafted on carbon microspheres as novel heterogeneous catalysts and their application for the reduction of nitrophenol and one-pot multicomponent synthesis of hexahydroquinolines†
Received
18th September 2017
, Accepted 4th December 2017
First published on 4th December 2017
Abstract
Novel carbon microsphere-supported metallic copper nanoparticles (Cu-NP/C) were synthesized using a low-cost and facile method based on carbonising a styrene-based, chelate-forming cation exchange resin loaded with Cu2+ ions. The metal–organic framework served as both copper and carbon source. Cu-NP/C microspheres were characterized by XRD, Raman, SEM, TGA, EDAX, and BET surface area analyser and were employed as heterogeneous catalysts for the reduction of 4-nitrophenol to 4-aminophenol by NaBH4 and for the synthesis of medicinally significant hexahydroquinoline derivatives based on the one-pot, multi-component reaction of aldehydes, dimedone, ethyl acetoacetate, and ammonium acetate. The sustainable and cheap starting material, the relatively easy synthetic procedure, the catalytic efficiency, and the easy separation and reusability of Cu-NP/C microspheres open the door for their wide-scale application.
Introduction
Catalysis is at the heart of chemical transformations; therefore, there are continuous efforts to develop new catalysts and increase catalytic performance for various applications. Metallic nanoparticles (NPs) have become essential tools for many chemical processes employed in industry and academia during recent decades.1–5 NPs possess high specific surface area, various shapes and sizes, and high reactivity, and they often exhibit activity different from that of the corresponding bulk materials, due to their distinctive quantum properties. Handling of NPs, however, is not easy, because NPs are often prone to self-aggregation, and it is difficult to separate them from reaction media because the classical, convenient filtration methods are not applicable. One economical way to overcome or minimise these limitations is to anchor NPs on supports, with the latter also serving as modifiers of catalytic properties. Nowadays, economic considerations and health issues are gaining more and more importance, and the development of catalytic NPs that are inexpensive, non- or minimally-poisonous, highly active, selective, stable, robust, and easily separable from a reaction mixture is highly desirable. Cu NPs provide a very promising alternative to heavy metal NP catalysis,1 and the aim of the present work is to provide a novel route to carbon microsphere-supported Cu NPs and to test their catalytic activity in two selected reactions, namely, the reduction of 4-nitrophenol (4NP) and one-pot multicomponent synthesis of hexahydroquinolines. The combination of reactive NPs with microsized support is important for keeping the high activity of NPs while providing a possibility for easy catalyst handling and separation. We show in this paper that both Cu NPs and carbon support can be simultaneously and economically prepared by carbonising copper ion-loaded, styrene-based cation exchange resins without using any additional reducing agent. Several carbon-supported Cu-based NPs have been prepared previously using wet-chemical, photochemical, electrochemical, and microwave methods, as well as thermal treatments, and their synthesis was reviewed last year.1
The reduction of 4NP by NaBH4 to 4-aminophenol (4AP) is an industrially important reaction6 and a widely used model reaction to test the catalytic performance of metal nanocatalysts, due to the following reasons: (1) 4AP is the sole product of this reaction. (2) The reaction does not proceed at ambient temperature without a catalyst. (3) The reaction can be easily observed by a colour change from yellow (4NP) to colourless (4AP) and monitored by UV/vis spectroscopy following the decrease of the absorption band of 4-nitrophenolate anion at 400 nm.6,7 The reaction was shown to follow pseudo-first-order kinetics in the presence of a large excess of NaBH4, and the catalytic rate could be evaluated using first-order kinetics with respect to the 4-nitrophenolate concentration.6–9
Hexahydroquinolines (HHQs) are biologically active compounds and find applications in medicinal chemistry as anticancer, antimicrobial, antihypertensive, and antimalarial agents.10–14 HHQs, in general, are synthesized by the four-component Hantzsch's condensation reaction, involving various aldehydes, dimedone, ethyl acetoacetate, and ammonium acetate. Several homogeneous and heterogeneous catalysts such as inorganic salts, Bronsted and Lewis acids, ionic liquids, metal oxides, and zeolites were reported to be active in the condensation reaction.15–21 Many of these methods, however, involve synthetic problems associated with difficult separation processes and expensive, hardly available, or non-recoverable catalysts, expensive reagents, or long reaction time. Considering the widespread applications of HHQ derivatives, there is a need to develop new and efficient catalysts for the green synthesis of these heterocyclic compounds. Therefore, our aim was to develop a facile and cheap catalyst system for Hantzsch's HHQ synthesis by selecting nanosized copper as the catalytically active metal and carbon microspheres as the catalyst support.
In this paper, we present a novel method for synthesizing copper NP-decorated carbon microspheres, their characterisation, including the determination of their phase composition and morphology, and their application as heterogeneous catalysts in 4NP reduction and HHQ synthesis.
Results and discussion
Synthesis and characterization of Cu-NP/C microspheres
In order to synthesize Cu NP-loaded carbon microspheres (Cu-NP/C), a commercial styrene-based chelate-forming ion exchanger resin containing iminodiacetate (–CH2N(CH2COOH)2) functional groups with a binding capacity of 1 mol divalent metal cation per 1 dm3 resin was selected as both the carbon source and Cu ion binder (see Experimental section). We expected that the carbonisation of copper ion-loaded resin produces not only the carbon support but also a reductive environment for the formation of metallic copper. Since copper ions were distributed in the resin according to iminodiacetate functional groups, the formation of only small Cu particles was expected, due to diffusion at the carbonisation temperature. We note that iminodiacetate chelating functional groups selectively bind one equivalent of Cu(II) ions (see Scheme S1, ESI†). To find information about the stability and decomposition of loaded resin, thermogravimetric (TG) analysis was carried out. This latter indicated resin decomposition between 180 and 450 °C and a complete weight loss of 68.2% up to 900 °C (see Fig. S1, ESI†). Therefore, carbonisation of the resin was investigated in the 500–1000 °C temperature range in 100 °C increments, and the effect of heating time was studied by performing experiments for 2, 4, and 8 hours. The Cu content of microspheres prepared by carbonisation at 600 °C for 4 hours was determined to be 8.1% using ICP-OES after digesting microspheres in hot chromic acid. The Cu content on these microspheres’ surfaces was measured to be 0.2% after dissolving surface Cu in 34% aqueous nitric acid.
The phase composition of synthesized Cu-NP/C microspheres was determined by powder X-ray diffraction (XRD). Representative XRD diffractograms are shown in Fig. 1. The XRD study unambiguously indicated that only metallic copper was formed as the sole crystalline phase during carbonisation between 600 and 1000 °C, and besides Cu, a small amount of Cu2O appeared at 500 °C carbonisation (8, 6, and 3 at% of total Cu content, applying a heating time of 2, 4, and 8 hours, respectively; see Table S1, ESI†). The average crystallite size of copper particles was calculated to be 15 nm at 500 °C using the Scherrer equation, and it increased gradually up to 35 nm with increasing carbonisation temperature (see Table S1, ESI†). EDAX investigations indicated the presence of copper on the surface or close to the surface of microspheres (see Fig. S2, ESI†). No graphitisation was observed by XRD.
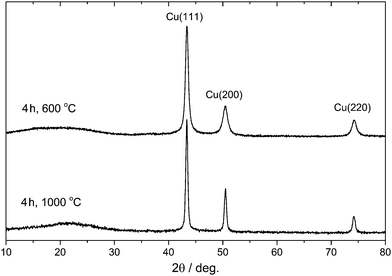 |
| Fig. 1 Powder X-ray diffractogram of selected Cu-NP/C microspheres (carbonisation time and temperature are indicated). | |
Raman spectroscopy confirmed the carbonisation of the resin (Fig. S3, ESI†), and selected spectra are shown in Fig. 2. The appearance of characteristic D, D′, G, and 2D Raman bands at 1329–1347, 1162–1240 (shoulder), 1588–1599, and 2660–2700 cm−1, respectively, as well as the D/G band intensity ratio, are characteristic of amorphous carbon.22 The graphitisation of the carbonaceous material, according to Raman spectra, is very low, in agreement with XRD. Raman bands characteristic of amorphous carbon appear on top of a broad background luminescence at 500 °C carbonisation, indicating imperfect carbonization at this temperature. This background, however, gradually decreases and disappears with increasing carbonisation time and temperature, respectively (Fig. 2 and Fig. S3, ESI†). The measured Raman intensity, in general, decreases with increasing carbonisation temperature, which indicates gradual replacement of sp3 carbon atoms by sp2 carbon atoms. Raman spectra of microspheres obtained by carbonisation between 600 and 1000 °C for 4 and 8 hours are very similar at the given temperature, indicating similar structural organisation of carbon microsphere surfaces.
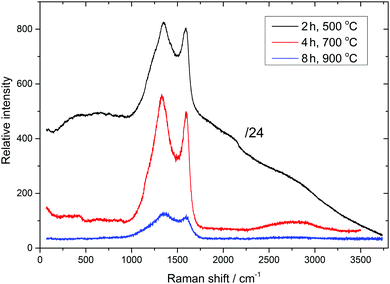 |
| Fig. 2 Raman spectra of selected Cu-NP/C microspheres (carbonisation time and temperature are indicated, see also Fig. S3, ESI†). | |
The shape and surface morphology of synthesized microspheres were studied by scanning electron microscopy (SEM). Cu-NP/C microspheres inherited the spherical morphology of ion exchange resins (see Fig. S4, ESI†), but their diameter decreased to half, 300–500 μm, due to shrinkage during the heat treatment. According to SEM, Cu NPs were distributed all over the surface of carbon microspheres. After some microspheres were broken in a mortar, SEM confirmed the distribution of Cu NPs inside the microspheres, too. Tiny Cu NPs are clearly visible on the surface of microspheres obtained by carbonisation at 500 and 600 °C, but they tend to be embedded into the carbon matrix at higher temperatures. A carbon collar forms around Cu NPs at about 800 °C, and NPs disappear in a seemingly fluffy carbon matrix at higher temperatures (Fig. 3 and Fig. S5–S7, ESI†). SEM investigations thus suggest that Cu-NP/C microspheres prepared at around 600 °C are the most promising candidates for catalytic applications. Cu NPs are known to be slowly oxidized by oxygen in air,1 and it was fortuitous for this study that microspheres synthesized two years ago and stored in a closed container but under air could be reinvestigated by XRD. The old samples prepared at 600 °C indicated the partial oxidation of copper (20 wt% of Cu was oxidized to Cu2O, possibly the surface content), but microspheres prepared at 1000 °C were practically unchanged (see Fig. S8 and S9, ESI†). This confirmed that Cu NPs were embedded in the carbon matrix and protected from air in the case of high-temperature synthesis. The specific surface area of microspheres was determined using nitrogen adsorption measurements and found to be between 3 and 9 m2 g−1 (Table S1, ESI†). The total pore volume for the sample prepared by carbonisation at 600 °C for 4 hours was measured to be only 0.0017 cm3 g−1, and the average pore width was estimated to be 2.2 nm. The measured nitrogen sorption isotherms suggested poorly developed micro- and mesopores (see Fig. S10, ESI†).
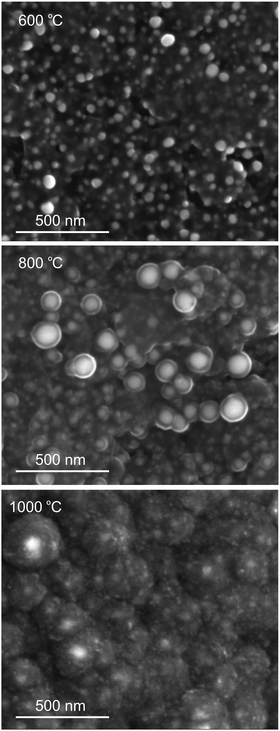 |
| Fig. 3 SEM image of the Cu-NP/C microsphere surface obtained by carbonisation of the copper-loaded ion exchange resin at 600, 800, and 1000 °C for 8 h (see also Fig. S5–S7, ESI†). | |
Catalytic activity of Cu-NP/C microspheres for the reduction of 4-nitrophenol
The Cu-NP/C sample prepared by carbonisation at 600 °C for 4 hours was selected for catalytic efficiency evaluation. 4NP was treated with a large excess of NaBH4 in water and in the presence of various amounts of catalysts (initial concentrations of 4NP and NaBH4 were 0.1 mM and 10 mM, respectively) at 20 °C, and the reaction was monitored by UV/vis spectroscopy. The characteristic absorption band of nitrophenolate anion appeared at 400 nm. Upon addition of Cu-NP/C microspheres, the absorption band decreased with time, and that of 4AP at 300 nm increased. The 4NP concentration decrease versus reaction time is shown in Fig. 4. No reaction between 4NP and NaBH4 was observed in the absence of Cu-NP/C microspheres (Fig. 4). When 4NP and NaBH4 solutions were mixed and the catalyst was added, an induction time of about 9 min was observed for the reaction. However, when the catalyst was added to the 4NP solution, stirred for 10 minutes, and the NaBH4 solution was consecutively added, the reaction proceeded without any induction time. The induction time of the 4NP and NaBH4 reaction was noted previously6,9 and was explained by a slow nanoparticle surface reconstruction in the presence of 4NP.9 Our observations are in line with this. In the presence of a large excess of NaBH4, the 4NP reduction reaction is ruled by first-order kinetics, indicated by the linear correlation of −ln(ct/c0) versus time (min) plots, where c is the 4NP concentration at the given time (see Fig. S11, ESI†). The apparent rate constant, kapp, can be determined from the slope of the fitted line. Increasing the amount of the catalyst used, the rate constant increased due to the increasing number of active sites for the reaction. The ratio of the rate constant over the total weight of the Cu-NP/C catalyst, k = kapp/mcat, where m is the mass of catalyst used, was determined to be between 68 and 100 min−1 g−1. In separate experiments, Cu NPs were removed from the microsphere surface using 1
:
1 diluted nitric acid. These ‘bare’ carbon microspheres did not catalyse the 4NP reduction, confirming the importance and catalytic activity of Cu NPs in 4NP reduction. Cu-NP/C microspheres prepared by carbonisation at 1000 °C for 4 hours were also investigated, but negligible catalytic activity was observed (k = 4 min−1 g−1; Fig. S12, ESI†), which is in line with SEM investigations indicating embedding of Cu NPs in the carbon matrix at high temperature.
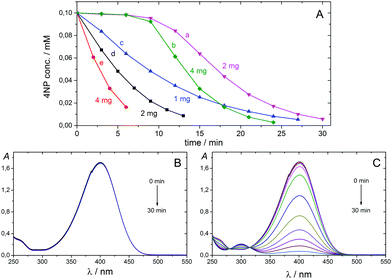 |
| Fig. 4 (A) Concentration of 4NP versus time for the 4NP reduction by NaBH4 in the presence of Cu-NP/C microspheres (amount of catalyst used is indicated; initial 4NP and NaBH4 concentrations are 0.1 and 10 mM; catalyst was added to mixed solutions of 4NP and NaBH4 (a and b); 4NP solution was stirred with the catalyst for 10 min before adding NaBH4 solution (c–e), temperature = 20 °C). Time dependent evolution of UV/vis spectra of 4NP anion in the absence (B) and the presence (C) of Cu-NP/C catalyst showing the reduction of 4NP by NaBH4. | |
Catalytic activity of Cu-NP/C microspheres in the one-pot multi-component synthesis of hexahydroquinolines
The catalytic activity of Cu-NP/C microspheres prepared by carbonisation at 600 °C for 4 hours was investigated for the synthesis of HHQs via the four-component Hantzsch's condensation reaction (Scheme 1). The reaction was performed on a preparative scale, and thus was not monitored by spectroscopy. The reaction mixture was worked up using standard preparative methods, and isolated yields were determined in all cases. Instead of varying, the reaction time was fixed to two hours, and conclusion on catalytic activity was made on the basis of isolated product yields. For reactions with apparently lower rate, reaction time was increased in selected cases. In order to study the effect of solvent and catalyst concentration on product yield, the reaction between benzaldehyde (1 mmol), dimedone (1 mmol), ethyl acetoacetate (1.2 mmol) and ammonium acetate (1.5 mmol) was selected. The effect of solvent on the rate of reaction was studied in the presence of 5 mg Cu-NP/C catalyst (ca. 5 wt% of benzaldehyde used). Among various screened solvents, namely, methanol, ethanol, isopropanol, 1,2-dichloroethane (DCE), THF, dioxane, and MeCN, ethanol was found to be the best in the studied model reaction concerning the yield of 2-amino-4-phenyl-3-cyano-7,7-dimethyl-5-oxo-1,4,5,6,7,8-hexahydroquinoline (Table 1). Without catalyst, the yield of the reaction was very low, below 5% for each solvent. Using ethanol as the most appropriate solvent, the amount of catalyst was tested, and 10 mg catalyst was found to be sufficient to complete the Hantzsch's transformation within 2 hours (Table 1).
 |
| Scheme 1 Synthesis of hexahydroquinolines using Cu-NP/C catalyst. | |
Table 1 Solvent screening and optimisation of catalyst concentration
Entrya |
Conditions |
Catalyst (mg) |
Isolated yield (%) |
See Scheme 1; Ar = phenyl; reagents: benzaldehyde (1 mmol), dimedone (1 mmol), ethyl acetoacetate (1.2 mmol), ammonium acetate (1.5 mmol), and solvent (2 mL). See Fig. S13–S15, ESI, for MS and NMR spectra.
|
1 |
THF, reflux, 5 h |
5 |
38 |
2 |
Dioxane, reflux, 5 h |
5 |
58 |
3 |
DCE, reflux, 3 h |
5 |
63 |
4 |
MeCN, reflux, 5 h |
5 |
74 |
5 |
iPrOH, reflux, 3 h |
5 |
76 |
6 |
MeOH, reflux, 2 h |
5 |
82 |
7 |
EtOH, reflux, 2 h |
5 |
87 |
8 |
EtOH, reflux, 2 h |
10 |
92 |
9 |
EtOH, reflux, 2 h |
15 |
94 |
10 |
EtOH, reflux, 2 h |
20 |
94 |
In order to test the general applicability of Cu-NP/C catalyst for HHQ synthesis, the reaction of various ortho, meta, and para substituted benzaldehydes, two of them synthesized for the first time, and thiophene-2-aldehyde with dimedone, ethyl acetoacetate, and ammonium acetate was studied under the optimized conditions (ethanol, reflux, 10 mg catalyst/1 mmol aldehyde). The catalyst was found to be efficient and provided excellent yields (82–94%) for the investigated derivatives (Table 2). A plausible and stepwise mechanism for the four-component reaction is suggested in Scheme S2 (ESI†), analogous to that in ref. 15. It involves four possible steps: (1) The catalyst enhances the electrophilicity of the carbonyl carbon of aldehyde, which reacts with the enolic form of dimedone to form arylidene intermediate (I). (2) Ethyl acetoacetate reacts with ammonium acetate to form β-enaminoester (II). (3) Arylidene intermediate (I) reacts with β-enaminoester (II), followed by (4) proton transfer and cyclization.
Table 2 Yields of various hexahydroquinolines using the Cu-NP/C catalyst
Entrya |
Ar group |
Isolated yield (%) |
See Scheme 1 and Table S2, ESI; conditions: reflux, 2 h, ethanol, 20 mg catalyst, aldehyde (2 mmol), dimedone (2 mmol), ethyl acetoacetate (2.4 mmol) and ammonium acetate (3 mmol), solvent 5 mL. See Fig. S13–S34, ESI, for MS and NMR spectra.
|
11 |
Phenyl |
92 |
12 |
2,4-Dichlorophenyl |
88 |
13 |
2-Chloro-4-fluorophenyl |
86 |
14 |
4-Chlorophenyl |
94 |
15 |
2-Fluorene |
90 |
16 |
4-Methoxyphenyl |
89 |
17 |
2-Hydroxyphenyl |
87 |
18 |
3-Hydroxyphenyl |
89 |
19 |
3-Nitrophenyl |
91 |
20 |
4-Nitrophenyl |
88 |
21 |
4-Bromophenyl |
87 |
22 |
2-Thiophenyl |
82 |
The reusability of the catalyst is important for practical applications, and it was studied by selecting the reaction between benzaldehyde, dimedone, ethyl acetoacetate and ammonium acetate, as well as the most favorable reaction conditions (ethanol, reflux, 10 mg catalyst/1 mmol aldehyde) and 2 hours of reaction time. Experiments revealed that the Cu-NP/C catalyst could be consecutively reused four times without significant loss of catalytic activity (Fig. 5). The slight decrease in yield of the corresponding product, 2-amino-4-phenyl-3-cyano-7,7-dimethyl-5-oxo-1,4,5,6,7,8-hexahydroquinoline, can be attributed to a small catalyst loss during its recovery and deactivation. The efficiency of the Cu-NP/C catalyst is compared to that of a number of previously reported heterogeneous catalysts in Table S3, ESI.†
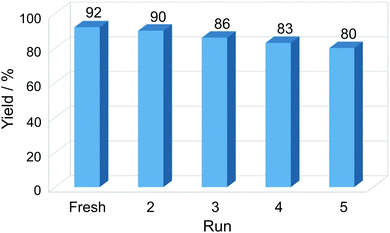 |
| Fig. 5 Graphical representation of the reusability of the Cu-NP/C catalyst. | |
Experimental
General information
Chemicals were reagent grade from commercial sources and used without further purification. Identification of synthesized hexahydroquinolines was based on melting point and spectroscopic measurements. Melting points were measured in open capillaries using an Optics technology melting point apparatus and were uncorrected. 1H NMR, 13C NMR, and DEPT spectra were obtained on Bruker Avance 250 and 400 spectrometers and referenced to tetramethylsilane (TMS). Infrared (IR) spectra were recorded on a Shimadzu FTIR spectrometer (Prestige IR21) using neat samples. Samples were analyzed for exact mass on a mass analyzer (Waters-TQD).
Synthesis of Cu-NP/C microspheres
A styrene-based VARION BIM-7 commercial chelate-forming ion exchanger resin, containing 7% divinylbenzene crosslinker, 2% acrylonitrile modifier, and iminodiacetate (–CH2N(CH2COOH)2) functional groups with a binding capacity of 1 mol divalent metal cation per 1 dm3 resin was used as the starting material. The resin was saturated with Cu2+ ions using aqueous copper sulphate (CuSO4) solution according to the procedure described in our previous studies.22,23 The exchanged resin was first dried in air and then placed in a drying box at 120 °C for 1 day. The dried resin was carbonized at 500, 600, 700, 800, 900, and 1000 °C for 2, 4, and 8 h in a high-purity dry nitrogen stream using an electric furnace. The furnace was heated to the desired temperature in 30 min, then cooled down to room temperature naturally after heat treatment.
Catalyst characterization
X-ray powder diffraction measurements were done on a Model PW 3710/PW 1050 Bragg–Brentano diffractometer using Cu-Kα radiation (λ = 1.541862 Å). Raman spectra were recorded on a HORIBA JobinYvonLabRAM HR confocal Raman microscope using He–Ne excitation (632 nm) and a laser power of 0.1 mW. BET specific surface area was determined using the volumetric method and nitrogen gas at liquid nitrogen temperature, using an ASDI RXM-100 catalyst characterization instrument. Samples were pre-treated in vacuum at 300 °C for 2 hours. TG measurements were performed on a modified Perkin-Elmer TGS-2 Thermo balance. A sample in a platinum sample pan was heated at 10 °C min−1 up to 900 °C under nitrogen atmosphere. Scanning electron microscopy (SEM) and EDAX analysis were performed using an FEI Quanta 3D high-resolution microscope. Resolution of the instrument was ≤ 1.2 nm at 30 keV accelerating voltage and in high vacuum. Spectrophotometric measurements were done using a Perkin-Elmer UV/vis Lambda 35 spectrometer applying a split width of 1 nm. Cu content was measured by inductively coupled plasma optical emission spectrometry (ICP-OES) using a Spectro Genesis instrument.
Reduction of 4-nitrophenol (4NP)
Two stock solutions were prepared by dissolving 4NP and NaBH4 separately in distilled water. A quartz cuvette with an optical path length of 1 cm was consecutively charged with the catalyst (1, 2, or 4 mg), 1.5 cm3 0.2 mM 4NP solution (stirred for 0 or 10 min before adding NaBH4), and 1.5 cm3 20 mM NaBH4 solution. Concentrations of 4NP and NaBH4 in the initial solution were 0.1 mM and 10 mM, respectively. The reaction mixture was stirred and monitored by UV/vis spectroscopy following the absorption band of 4NP anion at 400 nm. The stirring was stopped while recording the UV/vis spectrum.
General procedure for the synthesis of Hantzsch's hexahydroquinoline derivatives
A mixture of aldehyde (2 mmol), dimedone (2 mmol), ethyl acetoacetate (2.4 mmol), ammonium acetate (3 mmol) and Cu-NP/C catalyst (20 mg) in ethanol (5 mL) was refluxed for 2 h. The progress of the reaction was monitored by TLC (30% ethyl acetate: n-hexane). After completion of the reaction, the reaction mass was diluted with hot ethanol (10 mL) and filtered off to separate the catalyst. The filtrate was concentrated on a rotary evaporator to obtain the crude product, which was purified by recrystallization from ethanol to afford the corresponding pure hexahydroquinolines. The recovered catalyst was washed several times with hot ethanol, dried in an electric oven at 120 °C for about 30 minutes, and reused several times when it was required. Spectral data of newly synthesized compounds are as follows.
Ethyl-4-(2-chloro-4-fluorophenyl)-1,4,5,6,7,8-hexahydro-2,7,7-trimethyl-5-oxoquinoline-3-carboxylate (Table 2, entry 13).
M.p.: 180–181 °C; 1H NMR (DMSO-d6): δ = 0.8 (s, 3H), 1.19 (t, 3H), 1.9 (dd, 1H), 1.99 (s, 3H), 2.1 (dd, 1H), 2.2 (s, 3H), 2.3 (dd, 1H), 2.4 (dd, 1H), 3.9 (q, 2H), 5.12 (s, 1H), 7.05 (dd, 1H), 7.13 (dd, 1H), 7.25 (dd, 1H), 9.1 (s, 1H) ppm; 13C NMR (DMSO-d6): δ = 14.1, 18.2, 26.4, 29.0, 31.9, 34.5, 50.2, 58.9, 103.0, 109.4, 113.8, 115.6, 132.5, 141.6, 145.2, 149.7, 158.6, 161.0, 166.6, 193.9 ppm; IR(neat): 3270, 2902, 1780, 1670, 1604, 1060, 826, 890 cm−1; mass (m/z): 392 (M + 1)+.
Ethyl-4-(9H-fluoren-2-yl)-1,4,5,6,7,8-hexahydro-2,7,7-trimethyl-5-oxoquinoline-3-carboxylate (Table 2, entry 15).
M.p.: 224–225 °C; 1H NMR (DMSO-d6) δ = 0.9 (s, 3H), 1.0 (s, 3H), 1.19 (s, 3H), 1.98 (dd, 1H), 2.2 (dd, 1H), 2.3 (s, 3H), 2.5 (dd, 1H), 2.6 (dd, 1H), 3.8 (q, 2H), 4.0 (s, 2H), 4.9 (s, 1H), 7.19–7.35 (m, 4H), 7.5 (dd, 1H), 7.6–7.8 (dd, 2H), 9.1 (s, 1H) ppm; 13C NMR (DMSO-d6): δ = 14.1, 18.3, 26.4, 29.1, 32.1, 36.1, 36.3, 50.3, 59.0, 103.8, 110.1, 119.3, 119.6, 124.3, 125.0, 126.2, 126.4, 126.6, 138.8, 141.0, 142.4, 142.8, 144.8, 146.8, 149.4, 166.9, 194.3 ppm; IR(neat): 3279, 2957, 1703, 1649, 1210, 1072 cm−1; mass (m/z): 428 (M + 1)+.
Conclusions
In conclusion, we have developed a pyrolytic method for the synthesis of novel copper nanoparticle/carbon microsphere (Cu-NP/C) composites from Cu-loaded, iminodiacetate-functionalized, styrene–divinylbenzene copolymer (ion exchanger). The method is simple, cost-effective, and easy to scale-up for large-scale production. Microspheres have been characterized for their phase composition and surface morphology using various methods. Based on these observations, microspheres obtained by carbonising the ion exchanger at 600 °C for 4 hours have been selected for catalytic evaluation. The selected Cu-NP/C microspheres have demonstrated excellent catalytic activity for the reduction of 4-nitrophenol and for the one-pot, four-component synthesis of medicinally important hexahydroquinolines. Due to their facile and economical synthesis, reusability, activity, separability, and eco-friendliness, Cu-NP/C microspheres are expected to replace expensive noble metals in certain catalytic applications.
Conflicts of interest
There are no conflicts to declare.
Acknowledgements
We thank T. Váczi, I. Kovács, G. Varga, Sz. Klébert and Z. May for their assistance in instrumental measurements.
Notes and references
- M. B. Gawande, A. Goswami, F.-X. Felpin, T. Asefa, X. Huang, R. Silva, X. Zou, R. Zboril and R. S. Varma, Chem. Rev., 2016, 116, 3722 CrossRef CAS PubMed
.
- L. He, F. Weniger, H. Neumann and M. Beller, Angew. Chem., Int. Ed., 2016, 55, 12582 CrossRef CAS PubMed
.
- M. I. Din and R. Rehan, Anal. Lett., 2017, 50, 50 CrossRef CAS
.
- Y. Liu, G. Zhao, D. Wang and Y. Li, Natl. Sci. Rev., 2015, 2, 150 CrossRef
.
- G. Prieto, H. Tüysüz, N. Duyckaerts, J. Knossalla, G.-H. Wang and F. Schüth, Chem. Rev., 2016, 116, 14056 CrossRef CAS PubMed
.
-
J. Noh and R. Meijboom, in Reduction of 4-Nitrophenol as a Model Reaction for Nanocatalysis, ed. A. K. Mishra, Application of Nanotechnology in Water Research, Scrivener Publishing LLC, 2014, ch. 13, pp. 333–406 Search PubMed
.
- P. Zhao, X. Feng, D. Huang, G. Yang and D. Astruc, Coord. Chem. Rev., 2015, 287, 114 CrossRef CAS
.
- D. Rambabu, C. P. Pradeep, Pooja and A. Dhir, New J. Chem., 2015, 39, 8130 RSC
.
- S. Wunder, F. Polzer, Y. Lu, Y. Mei and M. Ballauff, J. Phys. Chem. C, 2010, 114, 8814 CAS
.
- S. C. Jadhavar, H. M. Kasraliker, S. V. Goswami and S. R. Bhusare, Heterocycl. Lett., 2016, 6, 717 CAS
.
- N. R. Mohamed, N. Y. Khaireldin, A. F. Fahmy and A. A. El-Sayed, J. Heterocycl. Chem., 2013, 50, 1264 CrossRef CAS
.
- H. M. Faidallah, S. A. F. Rostom, A. M. Asiri, K. A. Khan, M. F. Radwan and H. Z. Asfour, J. Enzyme Inhib. Med. Chem., 2013, 28, 123 CrossRef CAS PubMed
.
- X. H. Yang, G. M. Xiao, Z. M. Wang, Y. H. Zhou and G. D. Feng, Adv. Mater. Res., 2012, 581-582, 7 CrossRef CAS
.
- M. Vanaerschot, L. Lucantoni, T. Li, J. M. Combrinck, A. Ruecker, T. R. S. Kumar, K. Rubiano, P. E. Ferreira, G. Siciliano, S. Gulati, P. P. Henrich, C. L. Ng, J. M. Murithi, V. C. Corey, S. Duffy, O. J. Lieberman, M. I. Veiga, R. E. Sinden, P. Alano, M. J. Delves, K. L. Sim, E. A. Winzeler, T. J. Egan, S. L. Hoffman, V. M. Avery and D. A. Fidock, Nat. Microbiol., 2017, 2, 1403 CrossRef CAS PubMed
.
- S. Sobhani, F. Zarifi and J. Skibsted, New J. Chem., 2017, 41, 6219 RSC
.
- E. Tabrizian and A. Amoozadeh, J. Chin. Chem. Soc., 2017, 64, 331 CrossRef CAS
.
- S.-J. Yu, S. Wu, X.-M. Zhao and C.-W. Lu, Res. Chem. Intermed., 2017, 43, 3121 CrossRef CAS
.
- O. Goli-Jolodar, F. Shirini and M. Seddighi, RSC Adv., 2016, 6, 26026 RSC
.
- M. Abaszadeh, M. Seifi and A. Asadipour, Synth. React. Inorg., Met.-Org., Nano-Met. Chem., 2016, 46, 512 CrossRef CAS
.
- A. Khazaei, A. R. Moosavi-Zare, H. Afshar-Hezarkhani and V. Khakyzadeh, RSC Adv., 2014, 4, 32142 RSC
.
- L. Nagarapu, M. D. Kumari, N. V. Kumari and S. Kantevari, Catal. Commun., 2007, 8, 1871 CrossRef CAS
.
- T. Pasinszki, M. Krebsz, L. Kótai, I. E. Sajó, Z. Homonnay, E. Kuzmann, L. F. Kiss, T. Váczi and I. Kovács, J. Mater. Sci., 2015, 50, 7353 CrossRef CAS
.
- L. Kótai, T. Pasinszki, Zs. Czégény, Sz. Bálint, I. Sajó, Z. May, P. Németh, Z. Károly, P. K. Sharma, V. Sharma and K. K. Banerji, Eur. Chem. Bull., 2012, 1, 398 Search PubMed
.
Footnote |
† Electronic supplementary information (ESI) available: Figures containing results of TG, EDAX, Raman, SEM, sorption, NMR, MS, and UV measurements; plot of 4NP reduction kinetics; schemes for the synthesis of microspheres and mechanism of polyhydroquinoline formation; tables containing XRD and BET results, and melting points. See DOI: 10.1039/c7nj03562d |
|
This journal is © The Royal Society of Chemistry and the Centre National de la Recherche Scientifique 2018 |