DOI:
10.1039/C7NJ03138F
(Paper)
New J. Chem., 2018,
42, 574-586
A saccharinate-bridged palladacyclic dimer with a Pd–Pd bond: experimental and molecular docking studies of the interaction with DNA and BSA and in vitro cytotoxicity against human cancer cell lines†
Received
25th August 2017
, Accepted 28th November 2017
First published on 28th November 2017
Abstract
A new palladacyclic dimer [Pd2((C,N)L)2(μ-Sac)2] (1), in which L: C14H11NBr and sac: the saccharinate ligand, has been synthesized and completely characterized. X-ray crystallography has been used to determine the single crystal structure of this Pd(II) complex. In this dimer, two palladium(II) centers are bridged by a saccharinate anion, which is coordinated to the cyclopalladated units as a bidentate (N- and carbonyl O-atoms) ligand. According to DNA binding studies (UV-Vis spectroscopy, emission titration and viscosity measurements), the Pd(II) complex interacts with calf-thymus DNA (CT-DNA) through a groove binding mode with a binding affinity on the order of 105. Furthermore, UV-Vis and fluorescence emission spectroscopy have been used to monitor the binding of the complex to bovine serum albumin (BSA). The complex is mainly located in site I of the protein, based on the competitive experiments using Warfarin, Ibuprofen and Digoxin as site markers. The results of molecular docking confirmed the experimental data. Finally, the in vitro cytotoxicity of sodium saccharin, ligand LH (C14H12NBr), complex 1 and cisplatin against cervical cancer (HeLa), lung cancer (A549) and breast cancer (MCF-7) cell lines has been studied. The complexation process has significantly improved the anticancer activity, as the IC50 values show. Furthermore, complex 1 has been tested against NIH normal fibroblast cells. Therefore, based on the SI definition, 1 can be assigned as a selective compound against cancer cells.
1. Introduction
DNA and proteins are usually regarded as the main molecular targets for drugs, and many drugs exert their effects through binding to DNA or proteins. This is the basis for the design and discovery of novel and more efficient drugs.1–3 Therefore, the synthesis and interaction of metal complexes with DNA and proteins have been interesting research areas. Metal complex interactions with specific DNA sequences have been investigated in order to understand the mechanism of tumor inhibition, and to design new drugs and recognize specific sites or conformations targeted on DNA.4
Generally speaking, the three main modes of non-covalent interactions between most compounds and DNA are intercalative binding, groove binding and electrostatic attraction. Intercalation takes place when small molecules intercalate inside the nucleic acid base pairs, distorting the DNA backbone conformation. Major and minor groove binding involves hydrogen bonding or van der Waals interaction of the small molecules with the nucleic acids with no considerable distortion of the DNA backbone. Electrostatic interactions may occur between the negatively charged DNA helix phosphate backbone and small molecules with positive charges.5–8
Moreover, the development of metal-based therapeutic agents and the analysis of drug–protein interactions affecting the absorption, distribution, metabolism and excretion characteristics of drugs have been simultaneously concentrated on. Given its considerable binding properties, serum albumin plays an essential role in drug delivery as a drug carrier. The high cost of human serum albumin (HSA) has made the cheaper bovine serum albumin (BSA) widely applicable as an important model protein with regard to the interactions between compounds and serum albumins.9 The binding mode of interaction between proteins and drugs is basically of non-covalent nature. Thus, it is necessary to investigate drug–protein interactions to understand the mechanism of drug action and to design new drugs.10,11
The serious side effects of cisplatin, a principal metal-based drug, which targets DNA and its analogues, including nephrotoxicity and drug resistance by the tumor cells have motivated the discovery of more efficient cytotoxic complexes with other transition metals and novel ligands. The structural similarities and significant overlap of the coordination chemistry of platinum and palladium closely relate palladium-based complexes to their platinum analogues. The slow dissociation pattern of platinum complexes in comparison with palladium (105 times faster) makes them more applicable. The development of palladium complexes with slower rates of hydrolysis caused by the discrete choice of ligands in palladium-based anticancer drugs has recently been focused on. Cyclopalladated complexes containing a strong C–M s-bond, which can overcome all these problems, have proven to be very promising in this regard.12–17
Since coordinated ligands can modify key parameters such as reactivity and lipophilicity, they play a significant role in the anticancer activity of metallodrugs.18 The coordination of biologically active organic molecules such as ligands to the transition metal ions has been shown to be promising in this field due to the unique capability of these molecules to bind with different biological targets.19 Saccharin (1,2-benzisothiazoline-3-(2H)one 1,1-dioxide or o-sulphobenzimide) and its water soluble alkali and alkaline earth salts are presently the most widely used non-caloric artificial sweeteners throughout the world.20 The first row transition metal derivatives of saccharin are protease inhibitors.21 A superoxide dismutase-like activity has been reported for these and other metal saccharinates.22 Saccharin anions have various polyfunctional ligands due to the presence of several potential donor sites such as nitrogen, and one carbonyl and two sulfonyl oxygen atoms.23 Saccharin complexes have recently been found to act efficiently as biologically active agents.24,25 A strong anticancer activity against MCF-7 and MDA-MB-231 human breast cancer cell lines has been shown by the [Pd(sac)(tpy)](sac)·4H2O (sac: a saccharinate and tpy: 2,2':6′,2′′-terpyridine) palladium(II) complex by the induction of apoptosis via cell death receptors in vitro.26 Furthermore, trans-[Pt(2-hmpy)2(sac)2]·3H2O (2-hmpy: 2-(hydroxymethyl)pyridine) gives rise to relatively strong anti-growth effects against PC3 for lung cancer cells.27 Excellent anti-proliferative effects against HeLa cells of human cervical cancer have been exhibited by another platinum(II) complex with saccharin, K[Pt(sac)3(H2O)]. An IC50 value of 6.8 μM is comparable to that obtained for cisplatin.28
The synthesis, structural characterization and biological properties of a palladacyclic dimer with saccharinate bridges [Pd2((C,N)L)2(μ-sac)2] (1), in which L: C14H11NBr, LH: C14H12NBr (Scheme 1) and sac: the saccharinate ligand, are reported. The investigation of the biological characteristics of the complex has focused on (i) the binding properties with calf-thymus DNA (CT-DNA) studied using electronic absorption titration, fluorescence spectroscopy and viscosimetric measurements and (ii) the affinity for bovine serum albumin (BSA) investigated using UV-Vis and fluorescence spectroscopy. The binding constants (K) and number of binding sites (n) have also been calculated. In addition, molecular docking studies were carried out to obtain detailed binding information of the complex with DNA and BSA. Ultimately, an MTT assay has been used to evaluate the cytotoxic activity of the compounds against cervical cancer (HeLa), lung cancer (A549), breast cancer (MCF-7) and normal fibroblast cells (NIH).
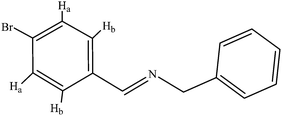 |
| Scheme 1 The molecular structure of the ligand (LH). | |
2. Experimental section
2.1. Materials
All solvents and chemicals were purchased from commercial sources and used without any further purification. Reagent grade calf-thymus DNA (CT-DNA), BSA and methylene blue (MB) were obtained from Sigma Aldrich Chemical Co.
The CT-DNA solutions gave a UV absorbance ratio (A260/A280) of over 1.8, showing the purity of the DNA.29 Extra pure solvents used in the syntheses and physical measurements were supplied by Merck Chemical Co. The tris(hydroxymethyl)-aminomethane (Tris) buffer used was of analytical reagent grade and was purchased from Merck Chemical Co. Doubly distilled deionized water was used in all solution preparations. CT-DNA and BSA stock solutions were prepared by dissolving them in 5 mM Tris buffer and 50 mM NaCl at pH 7.2. Absorption spectroscopy was used to determine the DNA concentration per nucleotide with a molar absorption coefficient of ε = 6600 M−1 cm−1 at 260 nm.30,31
2.2. Methods and instrumentation
An FT-IR JASCO 680-PLUS spectrophotometer was used to record Fourier transform infrared spectra in the 400–4000 cm−1 region using KBr pellets. Electronic absorption spectra were recorded using a JASCO 7580 UV-Vis-NIR double beam spectrophotometer in a 1 cm path length cell. A Cary Eclipse fluorescence spectrophotometer was used to record fluorescence spectra in solution. A Bruker spectrometer was used to record NMR spectra at 400.13 MHz (1H) and 100.61 MHz 13C {1H}. A Leco, CHNS-932 apparatus was used to carry out elemental analyses. A Cannon Fenske routine viscometer was used to perform viscometric titrations at a constant temperature of 298.0 (±0.1) K in a thermostatic water bath.
2.3. Syntheses
2.3.1. Synthesis of the ligand LH: C14H12NBr.
Benzylamine (273 μL, 2.5 mmol) was slowly added to 4-bromobenzaldehyde (0.462 g, 2.5 mmol) dissolved in methanol. The resulting clear solution was stirred at an ambient temperature for 24 h. The ligand was obtained as a white precipitate after evaporating the solvent. (Yield: 93.7%.)
Anal. calc. for C14H12BrN (%) C, 61.33; H, 4.41; N, 5.11. Found C, 61.23; H, 4.62; N, 4.97. IR (KBr, cm−1) ν(C–HPh) = 3081–3027, ν(C–H alph) = 2873–2851, ν(C
N) = 1644, ν(C
C aromatics) = 1483. 1H NMR (DMSO-d6, ppm): δ = 4.77 (s, 2H, CH2), 7.24–7.38 (m, 5H, CHph), 7.66 (d, 2H, Hb), 7.73 (d, 2H, Ha), 8.5 (s, 1H, HC
N). 13C {1H} NMR (DMSO-d6, ppm): 63.89 (CH2), 124.21, 126.8, 127.88, 128.34, 129.8, 131.69, 135.16, 139.36 (aromatic rings), 160.69 (HC
N).
2.3.2. Synthesis of complex 1: [Pd2((C,N)L)2(μ-sac)2].
A solution of Pd(OAc)2 (0.025 g, 0.1 mmol) in toluene (5 mL) was added dropwise to the ligand LH (0.06 g, 0.2 mmol) dissolved in toluene (10 mL). The resulting yellow solution was refluxed at 60 °C for 24 h to obtain complex (a) [Pd2((C,N)L)2(μ-OAc)2]. (Yield: 86.2%.)
IR (KBr, cm−1) ν(C–HPh) = 3083–3029, ν(C–H alph) = 2954–2852, ν(C
N) = 1608, ν(μ-OAc) = 1577, 1418 cm−1.
Excess NaCl was then added to a suspension of (a) in acetone/water solvent and the resulting mixture was stirred for 24 h at ambient temperature. The yellow precipitate formed was filtered, washed with water and then air-dried to obtain complex (b) [Pd2((C,N)L)2(μ-Cl)2]. (Yield: 89.9%.)
IR (KBr, cm−1) ν(C–HPh) = 3080–3024, ν(C–H alph) = 2956–2850, ν(C
N) = 1608 cm−1.
AgNO3 (0.017 g, 0.1 mmol) was added to a suspension of dimer (b) (0.042 g, 0.05 mmol) in acetone (15 mL) in the dark. Having been stirred for 2 h, the white AgCl precipitate formed was filtered from the solution. Sodium saccharin salt (0.024 g, 0.1 mmol) was then added to the filtrate and the resulting mixture was stirred for 24 h at ambient temperature. The solvent was removed to about 2 mL and n-hexane was added to precipitate a yellow solid of complex 1. Yellow crystals of 1 were obtained from the CH2Cl2:n-hexane solvent mixture. (Yield: 73.7%.)
Anal. calc. for C42H30Br2N4O6Pd2S2 (%) C, 44.9; H, 2.69; N, 4.99; S, 5.71. Found C, 45.23; H, 2.72; N, 4.84; S, 5.83. IR (KBr, cm−1) ν(C–HPh) = 3086–3028, ν(C–H alph) = 2955, 2853, ν(C
N) = 1620, ν(C
O) = 1669, ν(S
O) =1154, 1287 cm−1. 1H NMR (DMSO-d6, ppm): δ = 4.5 (s, 2H, CH2), 6.88-7.35 (m, 8H, aromatic L), 7.71–7.91 (m, 4H, the saccharin bridge), 8.34 (s, 1H, HC
N). 13C {1H} NMR (DMSO-d6, ppm): 59.8 (CH2), 120.2, 123.4, 123.45, 123.5, 127, 127.5, 128, 128.2, 128.4, 128.6, 129.6, 131, 133.1, 133.3, 137, 137.4, 142.5, 145.4 (aromatic rings), 154 (C–Pd), 165.6 (HC
N), 177 (C
O).
2.4. Crystallography
An Agilent SuperNova single crystal diffractometer (Cu K(α) radiation) was used to perform the X-ray diffraction experiments at 100 K. A multi-faceted crystal model based on the expressions derived by R.C. Clark and J.S. Reid was used to do the analytical numerical absorption correction.32 The structures were solved and refined using direct methods by the SHELXS97 and SHELXL (Sheldrick 2008) programs. Hydrogen atoms were added in the calculated positions and were riding on their respective carbons during the refinement.
2.5. DNA binding studies
A CT-DNA stock solution was prepared in Tris–HCl buffer (5 mM Tris–HCl, 50 mM NaCl at pH 7.2). A concentrated stock solution of the complex was prepared in DMSO solvent and then diluted suitably using Tris–HCl buffer at pH 7.2 to obtain the required concentrations in all the experiments. The ultimate DMSO concentration never exceeded 0.1% v/v. UV spectroscopy was used to study the interaction of 1 with CT-DNA, to investigate the possible binding mode to DNA and to calculate the binding constant to DNA (Kb). Absorption titration experiments were carried out at a constant complex concentration (60 μM) and various DNA concentrations (0.0–120 μM) in Tris-buffer. Furthermore, the complex 1 binding mode was further investigated using viscosity measurements. Sample viscosities were evaluated using the mean values of three replicate measurements. The relative specific viscosity (η/η0)1/3 values, in which η0 and η are the specific viscosity contributions of DNA in the absence (η0) and presence of each compound (η0), respectively, were plotted against r (r = [complex]/[DNA]).33 The relative viscosities were calculated using the relation η = (t − t0)/t0, in which t0 and t represent the flow times of the blank buffer and the DNA containing solutions, respectively.34
Fluorescence spectroscopy has been used to perform a competitive study of the complex with MB to examine the complex capability to displace MB from its DNA–MB complex. The DNA–MB solution ([DNA]/[MB] = 5) was excited at 630 nm in the absence and presence of different complex concentrations for MB displacement.35
2.6. Protein binding studies
A BSA stock solution was prepared by dissolving an appropriate quantity of BSA in the buffer solution (containing 5 mM Tris–HCl/50 mM NaCl at pH 7.2) and stored at 4 °C for further use. The BSA concentration was obtained by UV-Vis absorption spectroscopy using ε280 = 44
300 M−1 cm−1.36
The absorption titration experiments were carried out by keeping the BSA concentration constant (6 μM) while adding various complex concentrations (0.0–12 μM). Equal amounts of 1 solution were added to both the BSA and the reference solutions during the measurement of the absorption spectra to eliminate the absorbance due to the complex. In the tryptophan fluorescence quenching experiment, quenching of the tryptophan residues of BSA37 was performed by keeping the BSA concentration constant (6 μM) while varying the complex (quencher) concentration (0.0–12 μM), giving solutions with different molar ratios of the quenchers to BSA. Following each addition of the quencher, the fluorescence spectra were recorded at an excitation wavelength of 280 nm and an emission wavelength of 343 nm in the fluorometer.
In order to identify the binding location of 1 on BSA, site competitive replacement experiments were carried out in the presence of three site markers (Warfarin, Ibuprofen and Digoxin) using fluorescence titration methods. Equal BSA and site marker concentrations (6 μM) were used and 1 was then added gradually. The fluorescence spectra were recorded at 298 K with an excitation wavelength of 280 nm and emission in the 285–500 nm range.
2.7. Molecular docking
Molecular docking was used in the investigation of the binding mode and intermolecular interactions of the complex with DNA and BSA. The AutoDock 4.2 package by the Lamarckian genetic algorithm (LGA) was applied to perform molecular docking studies.38,39 The PDB format of 1 was obtained by converting the CIF file using Mercury software. The initial BSA structure was taken from the Protein Data Bank (PDB ID : 4F5S) at a resolution of 2.47 Å. Moreover, the d(CGCGAATTCGCG)2 DNA sequence was supplied by the Protein Data Bank (PDB ID : 3U2N) at a resolution of 1.25 Å. The chain (A) of BSA and all the hetero atoms including water molecules were removed during DNA and BSA input file preparation. Blind docking was first used to find the appropriate binding site for the complex interaction with DNA and BSA.40,41 Focus docking was then performed on the best location. DNA and BSA systems were restrained to a grid box with dimensions of 60 × 60 × 60 Å3 and a 0.375 Å grid spacing, in which almost the entire macromolecules were involved. All other parameters were maintained at their default values.
2.8. The cell proliferation assay
The growth inhibitory effect of the compounds against HeLa, A549 and MCF-7 cancer cell and NIH normal cell lines was measured using a 3-(4,5-dimethylthiazol-2-yl)-2,5-diphenyltetrazoliumbromide (MTT) assay.42 A predetermined concentration of tumor cells was seeded into the wells of a 96-well plate using different compound concentrations (0–100 μmol dm−3) and incubated at 37 °C with 5% CO2 and 95% humidity for 48 h. 10 μL of MTT solution (5 mg mL−1) was then added to each well and the plates were incubated for an additional 4 h at 37 °C. The insoluble formazan produced was finally dissolved by adding dimethyl sulfoxide (DMSO) (100 μL per well). Having completely dissolved the dye, the optical density (OD) was recorded at 570 nm with a reference wavelength of 630 nm in an enzyme-linked immunosorbent assay (ELISA) reader (Bio-Tek ELx808, USA). The percentage inhibition of cells exposed to various treatments was calculated as follows: % Inhibition = 100 − [(test OD/non-treated OD) × 100]. Non-treated cultures in all experiments were composed of only DMSO solvent at a concentration equal to those in the test wells. The graph of inhibition percentage against different concentrations was used to determine the half maximal inhibitory concentration (IC50) values.43,44 The selectivity index (SI) was also measured based on the IC50 ratio of normal NIH and cancer (HeLa, A549 and MCF-7) cells. The SI values indicate selectivity of the sample to the tested cell lines. Samples with SI values more than 2 were considered to achieve high selectivity.45
3. Results and discussion
3.1. Synthesis and spectroscopic characterization
The ligand (LH: C14 H12NBr) was reacted with palladium acetate (Pd(OAc)2) (2
:
1 molar ratio) in toluene at 60 °C to yield the [Pd2((C,N)L)2(μ-OAc)2] dimer (a) containing acetate bridges. The [Pd2((C,N)L)2(μ-Cl)2] chloro-bridged dimer (b) was isolated on the addition of NaCl to an acetone/water solvent mixture of (a) in good yield. (b) was reacted with AgNO3 and sodium saccharin to form [Pd2((C,N)L)2(μ-sac)2] (1) (Scheme 2).
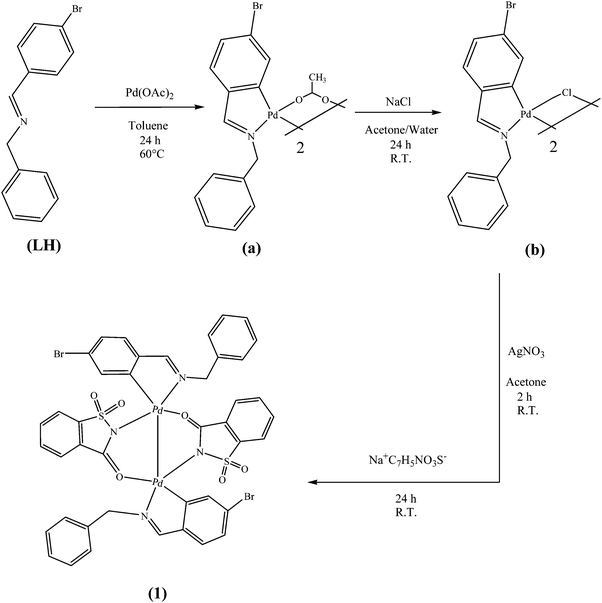 |
| Scheme 2 Synthetic route for the preparation of the Pd(II) complexes. | |
The complexes isolated as yellow solids were stable at room temperature and soluble in chlorinated solvents such as CH2Cl2 and CHCl3 and in polar, aprotic solvents such as DMSO. The elemental analysis of 1 is in good agreement with the calculated values. The formation of the isolated complexes has been confirmed on the basis of the characteristic peaks in the IR spectra. A significant band at 1644 cm−1 in the IR spectrum of the ligand is due to (C
N) stretching vibrations. ν(C
N) has shifted to a lower frequency in the complexes, indicating the binding of the imine nitrogen atom to the metal ion.46 The characteristic bands associated with the bridging acetato ligand at 1577 and 1418 cm−1 disappear when (a) is converted to (b).47 The symmetric and asymmetric (SO2) stretching absorptions of sac appear as strong absorptions at about 1154 and 1287 cm−1, respectively. The positions of the peaks corresponding to SO2 vibrations in the complex are almost similar to those of sodium saccharinate monohydrate. This suggests that the sulfonyl groups of the sac ligands are not involved in coordination with the Pd(II) ions.48 The ν(C
O) vibration, usually characterized by an extremely good group frequency, has often been exploited for structural studies. This mode generates a sharp band at 1642 cm−1 in sodium saccharinate,49 which shifts to lower frequencies when the carbonyl group participates in metal bonding. The ν(C
O) frequency does not correlate with the coordination mode of the ligand although an approximate ν(C
O) frequency limit for the distinction between the N-coordinated and uncoordinated saccharinates in solid metal complexes is suggested to be 1650 cm−1
50 due to intra- and intermolecular interactions.51 In this complex, ν(C
O) appears at 1669 cm−1.
A singlet signal corresponding to methylene protons is observed at 4.7 ppm in the 1H NMR spectrum of the ligand LH (Fig. S1, ESI†), which indicates that these two protons are equal and resonate independently of other nuclei. These two nuclei have become slightly shielded due to complex formation and resonate at 4.5 ppm (Fig. S2, ESI†). The aromatic protons of the ligand (Ha and Hb) appear as two doublets at 7.66 and 7.73 ppm, and the non-equivalency of these protons causes splitting and formation of doublets. These protons have resonated at a lower frequency (6.88–7.35 ppm) in the 1H NMR spectrum of the complex. In addition, a singlet is observed at 8.5 ppm in the ligand spectrum, which corresponds to the resonance of imine protons of the LH. This signal has also shifted to a lower frequency (8.34 ppm) due to complex formation, as observed in Fig. S2 (ESI†). The 1H NMR spectrum of the complex shows a multiplet at 7.71–7.91 ppm, which is not observed in the spectrum of the ligand. This signal is associated with the aromatic hydrogens of the sac ligand, which have resonated at lower frequencies compared with free saccharinates. The 13C NMR spectrum of the ligand (Fig. S3, ESI†) shows an aliphatic carbon at 63.9 ppm, eight types of aromatic carbon in the range of 124.21–139.36 ppm and an imine carbon at 160.69 ppm. In the 13C NMR spectrum of complex 1 (Fig. S4, ESI†), the signal associated with the aliphatic carbon appears at 59.8 ppm and those of aromatic carbons are observed in the range of 120.2–145.4 ppm. In addition, the three peaks at 154, 165.6 and 177 correspond to the orthopalladated carbon, imine carbon of the ligand L and carbonyl carbon of the sac ligand, respectively. Only one set of signals is observed in the 1H- and 13C{1H} NMR spectra of 1 indicating that the cyclopalladated dimer consists of only one geometrical isomer in solution.47
3.2. Molecular structure of 1
Single crystal X-ray diffraction has been used in the characterization of complex 1 in the solid phase. Slow diffusion of hexane into a CH2Cl2 solution gave suitable 1 crystals. Fig. 1 shows an ORTEP view of the dinuclear complex 1.
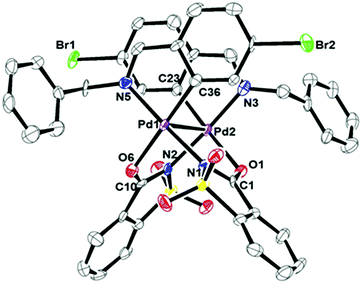 |
| Fig. 1 A partially labelled ORTEP view of the molecular structure of 1. | |
Table 1 summarizes the relevant crystallographic data and structural refinement details. Table 2 lists the selected bond lengths and angles. This compound was crystallized in the monoclinic space group I2/c with Z = 8. The coordination number around each palladium atom is four in this complex, indicating that the Pd(II) atom is bonded to an ortho-carbon atom and the imino nitrogen atom of L, an amido nitrogen and a carboxylate oxygen of the sac ligand in the distorted square planar geometry for each palladium atom with a Pd–Pd bond between them. The metal–metal bond length in this compound (2.9 Å) is less than the sum of the van der Waals radii for Pd (3.26 Å),52 consistent with the previously reported -NCO-bridged palladium dimers.53,54 The sums of the angles around the palladium atoms are 360.42 and 357.02° for Pd1 and Pd2, respectively, with the distortion most noticeable in the somewhat reduced “bite” angle of the metalated moiety. The restraints of the five-membered rings force the bond angles C36–Pd1–N5 and C23–Pd2–N3 to be 81.4(2) and 81.7(2)°, respectively. The planar coordination around d8 metallic centers often appears distorted in crystal structures. The distortion shows two ‘‘ideal’’ limiting cases: deviation towards tetrahedral coordination and distortion towards square pyramidal coordination.55 In complex 1, w1 and w2 are 2.33 and 4.24° for Pd1 and −0.11° and −6.22° for Pd2, respectively. These values are associated with tetrahedral distortion from the ideal square planar geometry. The Pd–Cpalladate distances (1.982(6) and 1.962(6) Å) are shorter than the predicted value of 2.081 Å (based on the sum of the covalent radii for C(sp2) and Pd, which are 0.771 and 1.31 Å, respectively).56 However, they are similar to those observed in a related orthopalladated complex.57,58 As the molecular structure shows, the sac ligand bridges the two palladium(II) centers and is coordinated to the cyclopalladated units as a bidentate (N- and carbonyl O-atoms) ligand. The Pd1–N1 and Pd2–N2 bond lengths (2.055(5) and 2.035(5) Å), respectively, are longer than the Pd1–N5 and Pd2–N3 distances (2.039(5) and 2.020(5) Å, respectively). This lengthening shows a weakening of the Pd–Nsac bond as a result of the great trans influence of the L imino nitrogen. Furthermore, the Pd–O and C
O bond lengths (due to the sac ligand) in this compound lie in the ranges obtained for related complexes.53,54,59
Table 1 crystallographic data and structure refinement details for complex 1
1
|
Empirical formula |
C84H60Br4N8O12Pd4S4 |
Formula weight |
2246.88 |
T/K |
100(1) |
Crystal system |
Monoclinic |
Space group |
I2/c |
a/Å |
43.3833(10) |
b/Å |
9.7679(2) |
c/Å |
38.6961(7) |
α/° |
90 |
β/° |
94.968(2) |
γ/° |
90 |
V/Å3 |
16336.4(6) |
Crystal dimensions/mm3 |
0.13 × 0.12 × 0.09 |
Z
|
8 |
μ/(mm−1) |
10.80 |
D
calc/Mg m−3 |
1.827 |
F
000
|
8832 |
Θ range/° |
4.1–68.8 |
Independent reflections |
14 931 |
Data/restraints/parameters |
12 923/0/1040 |
Goodness-of-fit on F2 |
0.966 |
Final R indices |
R
1 = 0.0542, wR2 = 0.1481 |
R indices (all data) |
R
1 = 0.0638, wR2 = 0.1564 |
Largest difference peak and hole/e Å−3 |
1.74, −1.71 |
CCDC |
1522173
|
Table 2 Selected bond lengths (Å) and angles (°) for complex 1
Atoms |
Bond lengths |
Pd1–N5 |
2.039(5) |
Pd2–C23 |
1.962(6) |
Pd1–N1 |
2.055(5) |
Pd2–N3 |
2.020(5) |
Pd1–O6 |
2.176(4) |
Pd2–N2 |
2.035(5) |
Pd1–C36 |
1.982(6) |
Pd2–O1 |
2.168(4) |
O6–C10 |
1.258(7) |
O1–C1 |
1.257(7) |
Pd1–Pd2 |
2.9076(5) |
|
|
|
Bond angles |
C36–Pd1–O6 |
173.3(2) |
O6–Pd1–Pd2 |
83.22(10) |
N5–Pd1–O6 |
97.00(18) |
C23–Pd2–N3 |
81.7(2) |
N1–Pd1–O6 |
87.82(17) |
C23–Pd2–N2 |
94.0(2) |
C36–Pd1–N5 |
81.4(2) |
N3–Pd2–N2 |
175.7(2) |
N5–Pd1–Pd2 |
105.36(13) |
C23—Pd2–O1 |
170.95(19) |
N1–Pd1–Pd2 |
78.71(13) |
N3–Pd2–O1 |
94.64(18) |
3.3. DNA binding studies
3.3.1. Electronic absorption titration.
An effective technique to study the interaction of CT-DNA with the metal complexes is electronic absorption spectrometry. The intensity of the ligand centered π–π* absorption band (235 and 253 nm) decreases when DNA is added to 1 incrementally (Fig. 2). The observed hypochromic change with no shift in the UV spectrum of the complex suggests that it binds to DNA via the groove binding mode.60,61 The Wolfe-Shimmer equation was used to calculate the intrinsic binding constant, Kb, for the complex: | [DNA]/(εa − εf) = [DNA]/(εb − εf) + 1/(Kb(εb − εf)) | (1) |
where [DNA] is the DNA concentration and εa, εf and εb correspond to Aobs/[complex], extinction coefficients of the free complex and the complex in the fully bound form, respectively. The slope and the intercept of the linear fit of a plot of [DNA]/(εa − εf) vs. [DNA] provide 1/(εb − εf) and 1/Kb(εb − εf) (Fig. 2, inset). The ratio of the slope to the intercept can be used to obtain the intrinsic binding constant (Kb).62 A Kb value of 1.05 × 105 M−1 was obtained for 1 using the above equation. The Kb value is close to that of the previously reported palladium(II) saccharinate complexes.63
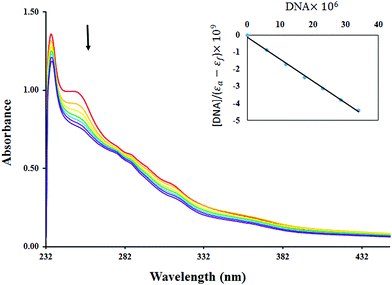 |
| Fig. 2 Electronic spectra of 1 in buffer solution (5 mM Tris–HCl/10 mM NaCl at pH 7.2) upon the addition of CT-DNA. [Complex] = (6 × 10−5 M), [DNA] = (0–6 × 10−4 M). The arrow indicates the decrease in absorption intensity upon increasing the DNA concentration. Inset: Plots of [DNA]/(εa − εf) vs. [DNA] for the titration of 1 with CT-DNA. | |
The free energy (ΔG) of the compound–DNA complex was calculated from the values of the binding constant (Kb) using the following equation:
| ΔG = −RT ln Kb | (2) |
Binding constants are measures of the compound–DNA complex stability whereas free energy is an indication of the spontaneity/non-spontaneity of compound–DNA binding. Free energies of 1 were evaluated as a negative value (−6.85 kcal mol−1), indicating the spontaneity of compound–DNA interaction.
3.3.2. Fluorescence studies of competitive DNA binding.
Emission titration was performed for further confirmation of the interaction between the complex and CT-DNA. The fluorescence measurement of the complex with or without CT-DNA at room temperature in aqueous solutions showed no emission band. Thus, the emission spectra do not directly show the binding of the compound with the CT-DNA. A competitive binding experiment was carried out using methylene blue (MB) as a probe to further study the interaction mode of the complex with DNA. MB is a planar cationic dye, which is well-known for independent intercalation into CT-DNA. To investigate the interaction of the complexes with DNA, a competitive MB displacement assay was performed.64 It has been previously reported that the fluorescence intensity of the MB-DNA system increases by adding certain complexes.65–67 The complexes are thus bound to the DNA base pairs via a classical intercalation mode while replacing MB.68 The DNA induced emission intensity at 680 nm (630 nm excitation) decreased upon the addition of 1 to DNA pretreated with MB (Fig. 3) because this complex binds to DNA via non-covalent groove binding.69
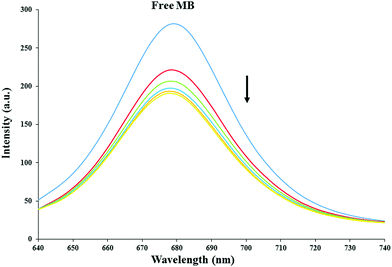 |
| Fig. 3 Emission spectra of the DNA–MB system in the presence of 1, [DNA] = 5 × 10−5 M, [complex] = 0–2 × 10−5 M, [MB] = 1 × 10−5 M. The arrow indicates the decrease in emission intensity upon increasing the complex concentration. | |
3.3.3. Viscosity measurements.
Viscosity measurements were carried out by keeping the DNA concentration constant and changing the concentration of 1 (Fig. 4). DNA viscosity is sensitive to DNA length changes. The relation between the relative viscosity (η/η0) and DNA length (L/L0) is L/L0 = (η/η0)1/3, in which L0 and L are the apparent molecular lengths in the absence and presence of the compound, respectively.70 The viscosity of the DNA solution significantly increases when a complex binds to DNA via a classical intercalative mode since DNA base pairs are separated to accommodate the binding complex, giving rise to the lengthening of the DNA helix and subsequent increase in DNA viscosity.71 However, a small molecule, which binds exclusively in the DNA grooves under the same conditions, causes little or no change to the DNA solution viscosity.34 These results again suggest the interaction of 1 with the groove of DNA in accordance with the above experimental results.
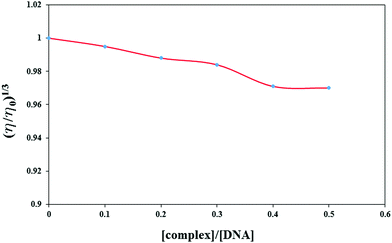 |
| Fig. 4 The effect of increasing amounts of 1 on the viscosity of CT-DNA (5 × 10−5 M) in 5 mM Tris buffer (r = 0, 0.1, 0.2, 0.3, 0.4 and 0.5). | |
3.4. Protein binding experiments
Proteins are major targets for therapeutically active complexes. Since the absorption, metabolism and distribution of the drugs are greatly affected by drug–protein interactions,72 the investigation of drug–protein interaction is an active field of interest in terms of understanding the mechanism of drug action and the possibility of designing new and useful medicines. Consequently, UV-Vis and fluorescence techniques were used to investigate the binding properties of 1 with BSA.
3.4.1. Electronic absorption titration.
Protein structural changes and protein drug complex formation can be understood by UV-Vis absorption spectroscopy. The BSA absorption spectra in the absence and presence of different concentrations of 1 were recorded at ambient temperature (Fig. 5). BSA has two main absorption bands: one located in the 210–240 nm range, which is the skeleton absorption peak and the other at 280 nm associated with the π → π* transition of the aromatic amino acids (Trp, Tyr, and Phe).73 The addition of 1 obviously decreases the BSA absorption peak at 228 nm which shifted toward longer wavelengths, indicating a perturbation of the α-helix caused by a specific interaction between the complex and BSA.74 In addition, the maximum absorption changes at 280 nm show that 1 interacts with the BSA molecule and the microenvironment of the three aromatic acid residues changes in the presence of the complex.75
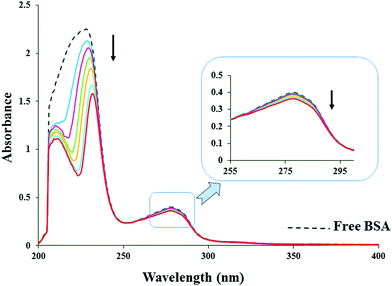 |
| Fig. 5 UV absorption spectra of [BSA] = (6 × 10−6 M) in the absence and presence of different concentrations of 1 (0–6 × 10−5 M). | |
3.4.2. Fluorescence quenching studies.
There are three fluorophores in BSA, tryptophan, tyrosine and phenylalanine residues. The intrinsic BSA fluorescence is usually contributed by tryptophan alone since phenylalanine has a very low quantum yield and tyrosine fluorescence is almost completely quenched if it is ionized.76 BSA has a strong fluorescence emission peak at 345 nm (excited at 280 nm) (Fig. 6). The BSA fluorescence intensity regularly decreases with increased concentrations of 1, whereas the emission maximum wavelengths and shape of the peaks remain almost unchanged. This indicates that 1 binds to BSA and quenches its intrinsic fluorescence. After correction for the inner-filter effect using eqn (3):77 | Icorr = Iobse(Aex+Aem)/2 | (3) |
where Icorr and Iobs are the corrected and measured fluorescence, respectively, and Aex and Aem are the absorbance of the complex at the excitation and emission wavelengths, respectively, the corrected fluorescence quenching data were analyzed using the Stern–Volmer equation:78 | 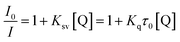 | (4) |
where I0 and I are the fluorescence intensities in the absence and presence of the complex, respectively, Ksv is the Stern–Volmer quenching constant, [Q] is the quencher concentration, kq is the bimolecular quenching rate constant and τ0 is the average lifetime of the fluorophore without a quencher. Ksv can be obtained from the slope of a plot of I0/I vs. [Q] (Fig. 6 insets). The Ksv value for 1 is 1.08 × 105 M−1 in this work. Since the τ0 value for tryptophan fluorescence in proteins is 2 × 10−8 s,79 the quenching rate constant, kq, can be calculated using the following equation:
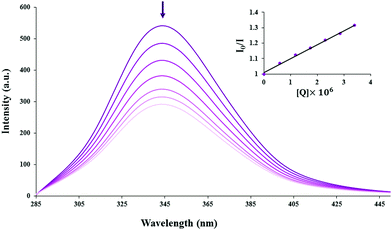 |
| Fig. 6 Emission spectra of BSA upon the titration of 1. [BSA] = (6 × 10−6 M), [complex] = (0–6 × 10−5). The arrow shows the change upon increasing the complex concentration. Inset: Plots of I0/I vs. [Q] × 106. | |
The quenching constant (kq) obtained for 1 was 5.4 × 1012 M−1 s−1. Quenching mechanisms are generally classified as dynamic and static. Dynamic quenching is the process in which the fluorophore and the quencher come into contact during the transient existence of the excited state. Static quenching refers to the formation of a fluorophore quencher complex in the ground state. The kq value (ca. 1012 M−1 s−1) of complex 1 is greater than the maximum scatter collision quenching constant, i.e. 2.0 × 1010 M−1 s−1. This indicates the existence of a static interaction between BSA and the added complex.80,81
If similar and independent binding sites are assumed in the biomolecule for the static binding constant (Kb), the quenching interaction and the number of binding sites (n) can be calculated using the following equation:82
| 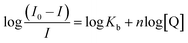 | (6) |
where
Kb and
n are the binding constant and number of binding sites, respectively. Plots of log[(
I0 −
I)/
I]
vs. log[Q] are linear (
Fig. 7). The intercept and slope of such plots can be used to calculate
Kb. Values of
n close to 1 indicate that there is a single class of binding site for the complex on BSA (
Table 3). The binding free energy of
1 to BSA is −8.06 kcal mol
−1.
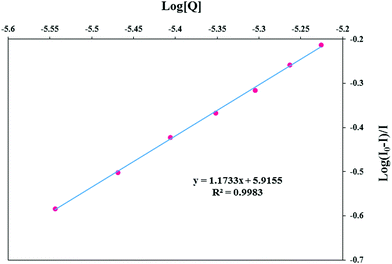 |
| Fig. 7 Scatchard plots of log[(I0 − I)/I] vs. log[Q] for the determination of the complex–BSA binding constant and the number of binding sites on BSA for 1. | |
Table 3 Estimated binding constants for site marker competitive experiments of the complex–BSA system
System |
K
b (M−1) |
n
|
R
|
Complex–BSA |
8.23 × 105 |
1.17 |
0.9983 |
Complex–BSA–Warfarin |
6.66 × 104 |
0.98 |
0.9980 |
Complex–BSA–Ibuprofen |
7.18 × 105 |
1.16 |
0.9992 |
Complex–BSA–Digoxin |
7.94 × 105 |
1.20 |
0.9960 |
3.4.3. The fluorescence resonance energy transfer (FRET) between the complex 1 and BSA.
Energy transfer between two molecules is an important physical phenomenon of considerable interest for understanding some biological systems.83 The mechanism of fluorescence resonance energy transfer involves a donor fluorophore in an excited electronic state, which may transfer its excitation energy to the nearby acceptor chromophore in a non-radiative fashion through long-range dipole–dipole interactions.84 The effective energy transfer from a protein residue (donor) to a complex (acceptor) occurs under the following pre-conditions: (i) the donor can produce fluorescence light; (ii) the overlap between the fluorescence emission spectrum of the donor and the UV-Vis absorption spectrum of the acceptor is enough; (iii) the donor and the acceptor must be in close proximity to each other (typically 1 to 10 nanometers).85 The overlap of the absorption spectrum of 1 with the fluorescence emission spectrum of BSA in the wavelength range of 285–500 nm is shown in Fig. 8. The energy transfer efficiency (E) is related not only to the donor–acceptor distance (r), but also to the critical energy transfer distance (R0). This is described by eqn (7):86 | 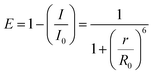 | (7) |
where E is the energy transfer efficiency, I0 and I are the fluorescence intensities of BSA in the absence and presence of the complex, r is the acceptor–donor distance and R0 is the critical energy transfer distance when the transfer efficiency is 50%. The value for R0 can be calculated using eqn (8):87 | R60 = 8.79 × 10−25K2N−4ΦJ | (8) |
where K2 is the dipole orientation factor, N is the refractive index of the medium, Φ is the fluorescence quantum yield of the donor and J is the spectral overlap integral between the fluorescence emission spectrum of the donor and the absorption spectrum of the acceptor which is calculated as:87 | 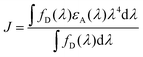 | (9) |
where fD(λ) is the donor emission spectrum and εA is the acceptor molar extinction coefficient normally obtained from an absorption spectrum. In the present case, K2 = 2/3, N = 1.336 and Φ = 0.15. Hence, using eqn (7)–(9) above, the following parameters can be calculated: J = 6.372 × 10−14 cm3 M−1, R0 = 3.47 nm, E = 0.38 and r = 3.76 nm for 1. The obtained value for the distance between the complex and BSA, r < 8 nm, and 0.5R0 < r < 1.5R0, indicates that the energy transfer from BSA to the complex occurred with high probability. The binding of the complex to BSA was formed through energy transfer, which quenched the fluorescence of BSA molecules, implying the presence of static quenching interaction.88 Furthermore, the greater r value compared with R0 confirms the static quenching mechanism.89
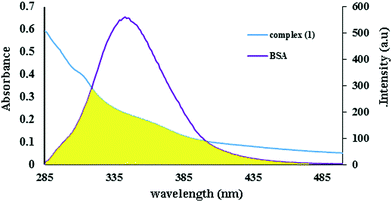 |
| Fig. 8 The spectral overlaps of the absorption spectra of 1 with the fluorescence spectra of BSA. | |
3.4.4. Site selective binding.
BSA protein contains three domains (I–III) with two subdomains (A and B) on each domain.90 Sites I, II and III of serum albumin exhibit attraction towards Warfarin, Ibuprofen and Digoxin, respectively, according to Sudlow et al.91 Competitive experiments were carried out using Warfarin, Ibuprofen and Digoxin to recognize the specificity of drug binding. The concentration ratio of BSA and the probe was 1
:
1 (6 × 10−6 M: 6 × 10−6 M) in these experiments (Fig. 9). The fluorescence intensity significantly decreased upon the addition of site markers into BSA solution. Plots of log[(I0 − I)/I] vs. log[complex] in the presence of site markers were prepared to compare the influence of Warfarin, Ibuprofen and Digoxin on the complex binding to BSA (Table 3). The binding constant for the complex–BSA system is 8.23 × 105 M−1 and it is surprisingly variable in the presence of Warfarin. This shows the simultaneous competition of Warfarin and 1. Thus, 1 binds to site I of serum albumin. This observation is further supported by docking studies, as will be discussed later.
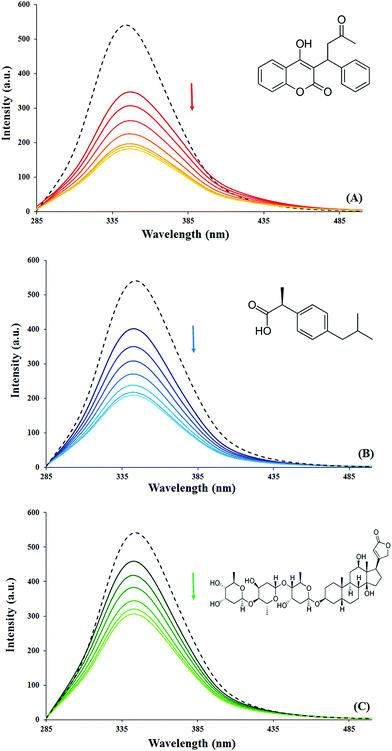 |
| Fig. 9 Influence of selected site markers; (A) Warfarin, (B) Ibuprofen and (C) Digoxin on the fluorescence of 1 bound to BSA. [BSA] = [site markers] = 6 × 10−6 M; [complex] = (0–6 × 10−5 M). Insets: Molecular structures of site markers. | |
3.5. Molecular docking
3.5.1. Docking with DNA.
Molecular docking is generally an attractive procedure for understanding drug–DNA interactions in rational drug design and discovery. Complex 1 has been successively docked with DNA to predict the appropriate binding sites in this study. The results show that the binding sites were obtained with the best binding free energy (ΔGb), (−6.5 kcal mol−1) for the interaction of 1 with DNA. This shows that the results obtained in the absorption titration experiments (−6.85 kcal mol−1) are reasonably comparable with those calculated using the computational method. According to Fig. 10, 1 interacts with DNA at the minor groove. In addition, this is stabilized by hydrogen bonding between the sulfonyl oxygen of the sac ligand and a guanine base of the DNA.
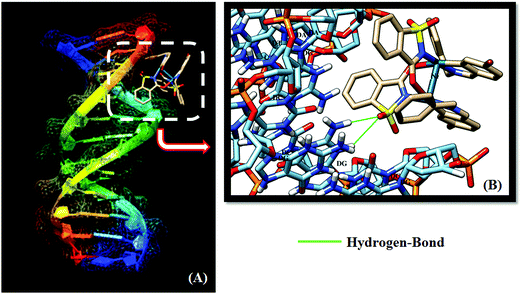 |
| Fig. 10 (A) The interaction of the complex with DNA using the Autodock 4.2 docking software. (B) The bases of DNA interactions with 1 in the active site. | |
3.5.2. Docking with BSA.
The molecular docking technique was also used to study the drug–protein interactions of 1 with the most probable site of BSA and verify our experimental results. The best value of ΔGb for the interaction of the complex with BSA was found to be −7.64 kcal mol−1. The obtained value is in agreement with the binding free energy obtained from the experimental value of Kb (−8.05 kcal mol−1). Fig. 11 shows the docked conformations. 1 binds to BSA through residues such as Trp 134, Pro 281, Leu 282, Leu 283, Glu 284, His 18, Leu 154 and Glu 17, as observed.
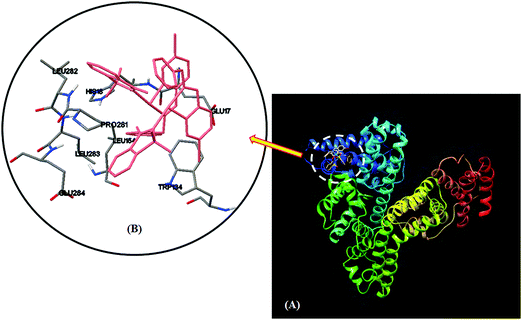 |
| Fig. 11 (A) Results of the docking procedure of 1 in the interaction with BSA. (B) The BSA amino acid residues interacting with the complex, which results in quenching. | |
3.6.
In vitro selective cancer cell cytotoxic activity
The MTT dye reduction method, as a widely accepted bioassay, was used in the evaluation of the in vitro cytotoxic activity of sodium saccharin, LH and 1 against HeLa cervical cancer, A549 lung cancer and MCF-7 breast cancer cells. Furthermore, the activity of complex 1 was tested against NIH normal fibroblast cells. Cytotoxicity is the degree to which an agent exhibits a specific destructive action on certain cells and is the quality of toxicity to cells. Cells were incubated in the presence of increasing concentrations of the complexes for 48 h after which the anti-proliferation activity was measured according to the procedure described in the Materials and Methods section. Cisplatin, a common anticancer drug, was used for comparison purposes under identical experimental conditions. 1 showed significant anti-proliferation activity against the cancer cell lines in the 0–100 μmol dm−3 range (Fig. S5, ESI†). Table 4 shows the cytotoxicity data, expressed as IC50 values, which are the concentrations of a compound inhibiting 50% of the cell growth. The in vitro cytotoxicity of 1 against the MCF-7 and A549 cell lines is higher than that of the widely used drug, cisplatin, under identical conditions, according to the IC50 values. The complexation process, on the other hand, significantly improves the anticancer activity. These results suggest that this complex may be a potential anticancer agent and further investigation is necessary to achieve better efficiency and determine the mode of action. Complex 1 exhibits low cytotoxic activity (IC50: 165 μM) against normal NIH cells after 48 h (Fig. S6, ESI†), although cisplatin as a standard cytotoxic compound showed significant toxicity. The selective effect of the compound was verified by SI values which were 7.43, 7.14, and 5.42 for MCF-7, A-549, and HeLa cell lines, respectively, in comparison with the normal NIH cells. Therefore based on the SI definition, complex 1 can be assigned as a selective compound against cancer cells. The results were supported by other studies in which more cytotoxicity was found towards cancer cells compared with the normal cells92–97 with regards the sensitivity of cancer cells towards the cytotoxic compounds.
Table 4 selective cytotoxicity data, expressed as IC50 (μM), of the compounds against cancer and normal cell lines
Compounds |
IC50 (μM)  SD |
HeLa |
A549 |
MCF-7 |
NIH |
Sodium saccharin |
>200 |
>200 |
>200 |
— |
Ligand LH |
>200 |
93.8 ± 4 |
>200 |
— |
Complex 1 |
30.4 ± 2 |
23.1 ± 1 |
22.2 ± 1 |
165 ± 1 |
Cisplatin |
17.6 ± 1 |
24.3 ± 1 |
26.3 ± 1 |
38.67 ± 4 |
4. Conclusion
A new Pd(II) complex has been synthesized and characterized using conventional spectroscopic techniques in this study. Single crystal X-ray diffraction studies have also been done to determine the molecular structures of the complex, showing that the Pd(II) ion is in a distorted square planar coordination environment. The DNA binding properties of the complex have been investigated using fluorescence spectroscopy, electronic absorption and viscosity measurements. The results suggest that 1 interacts with the CT-DNA via groove binding. The results also show that this compound binds to BSA and changes its structure, and the quenching of BSA fluorescence by the complex is of static type. Moreover, the bound complex is mainly located in site I, based on the competitive site marker experiments. Molecular docking studies verified the interaction mode of 1 with DNA and BSA. In addition, the in vitro anticancer activity of the free ligands and Pd(II) complex against the three cancer cell lines shows that 1 has more toxicity than the free ligands. The IC50 values indicate that the complex is a potential anticancer candidate against MCF-7 and A549 cell lines, but no significant viability reductions were observed in normal cells.
Conflicts of interest
There are no conflicts to declare.
Acknowledgements
We are grateful to the Isfahan University of Technology (IUT) for financial support.
References
- N. P. Barry and P. J. Sadler, Chem. Commun., 2013, 49, 5106–5131 RSC.
- G. Sava, G. Jaouen, E. A. Hillard and A. Bergamo, Dalton Trans., 2012, 41, 8226–8234 RSC.
- K. Zheng, F. Liu, X.-M. Xu, Y.-T. Li, Z.-Y. Wu and C.-W. Yan, New J. Chem., 2014, 38, 2964–2978 RSC.
- C. Rajarajeswari, R. Loganathan, M. Palaniandavar, E. Suresh, A. Riyasdeen and M. A. Akbarsha, Dalton Trans., 2013, 42, 8347–8363 RSC.
- M. A. Husain, S. U. Rehman, H. M. Ishqi, T. Sarwar and M. Tabish, RSC Adv., 2015, 5, 64335–64345 RSC.
- T. Sarwar, H. M. Ishqi, S. U. Rehman, M. A. Husain, Y. Rahman and M. Tabish, Int. J. Biol. Macromol., 2017, 98, 319–328 CrossRef CAS PubMed.
- M. Sirajuddin, S. Ali and A. Badshah, J. Photochem. Photobiol., B, 2013, 124, 1–19 CrossRef CAS PubMed.
- T. Sarwar, M. A. Husain, S. U. Rehman, H. M. Ishqi and M. Tabish, Mol. Biosyst., 2015, 11, 522–531 RSC.
- S. Dasmandal, A. Kundu, S. Rudra and A. Mahapatra, RSC Adv., 2015, 5, 79107–79118 RSC.
- Y. Bai, S. Sun, H. Zhang and T. Zhao, Anal. Methods, 2013, 5, 7036–7041 RSC.
- J. Haribabu, K. Jeyalakshmi, Y. Arun, N. S. Bhuvanesh, P. T. Perumal and R. Karvembu, RSC Adv., 2015, 5, 46031–46049 RSC.
- A. R. Kapdi and I. J. Fairlamb, Chem. Soc. Rev., 2014, 43, 4751–4777 RSC.
- L. Kelland, Nat. Rev. Cancer, 2007, 7, 573–584 CrossRef CAS PubMed.
- Y. P. Ho, S. C. Au-Yeung and K. K. To, Med. Res. Rev., 2003, 23, 633–655 CrossRef CAS PubMed.
- M. M. Shoukry, A. A. Shoukry and M. N. Hafez, J. Coord. Chem., 2010, 63, 652–664 CrossRef CAS.
- K. Karami, M. Hosseini-Kharat, H. Sadeghi-Aliabadi, J. Lipkowski and M. Mirian, Eur. J. Med. Chem., 2014, 73, 8–17 CrossRef CAS PubMed.
- E. G. Rodrigues, L. S. Silva, D. M. Fausto, M. S. Hayashi, S. Dreher, E. L. Santos, J. B. Pesquero, L. R. Travassos and A. C. Caires, Int. J. Cancer, 2003, 107, 498–504 CrossRef CAS PubMed.
- B. B. Zmejkovski, A. Savić, J. Poljarević, N. Pantelić, S. Aranđelović, S. Radulović, S. Grgurić-Šipka, G. N. Kaluđerović and T. J. Sabo, Polyhedron, 2014, 80, 106–111 CrossRef CAS.
- G. Gasser, I. Ott and N. Metzler-Nolte, J. Med. Chem., 2010, 54, 3–25 CrossRef PubMed.
- D. J. Ager, D. P. Pantaleone, S. A. Henderson, A. R. Katritzky, I. Prakash and D. E. Walters, Angew. Chem., Int. Ed., 1998, 37, 1802–1817 CrossRef CAS.
- C. T. Supuran, G. Loloiu and G. Manole, Rev. Roum. Chim., 1993, 38, 115 CAS.
- M. C. Apella, R. Totaro and E. J. Baran, Biol. Trace Elem. Res., 1993, 37, 293–299 CrossRef CAS PubMed.
- V. T. Yilmaz, S. Guney and C. Thöne, Z. Anorg. Allg. Chem., 2002, 628, 1406–1410 CrossRef CAS.
- E. J. Baran and V. T. Yilmaz, Coord. Chem. Rev., 2006, 250, 1980–1999 CrossRef CAS.
- Y. I. Binev, C. T. Petkov and L. Pejov, Spectrochim. Acta, Part A, 2000, 56, 1949–1956 CrossRef.
- E. Ulukaya, F. Ari, K. Dimas, E. I. Ikitimur, E. Guney and V. T. Yilmaz, Eur. J. Med. Chem., 2011, 46, 4957–4963 CrossRef CAS PubMed.
- F. Ari, N. Aztopal, C. Icsel, V. T. Yilmaz, E. Guney, O. Buyukgungor and E. Ulukaya, Bioorg. Med. Chem., 2013, 21, 6427–6434 CrossRef CAS PubMed.
- M. Cavicchioli, A. C. Massabni, E. E. Castellano, L. P. Sabeh and C. M. Costa-Neto, Inorg. Chim. Acta, 2007, 360, 3055–3060 CrossRef CAS.
- J. Marmur, J. Mol. Biol., 1961, 3, 208 CrossRef CAS.
- M. Reichmann, S. Rice, C. Thomas and P. Doty, J. Am. Chem. Soc., 1954, 76, 3047–3053 CrossRef CAS.
- P. Sathyadevi, P. Krishnamoorthy, N. S. Bhuvanesh, P. Kalaiselvi, V. V. Padma and N. Dharmaraj, Eur. J. Med. Chem., 2012, 55, 420–431 CrossRef CAS PubMed.
- R. Clark and J. Reid, Acta Crystallogr., Sect. A: Found. Crystallogr., 1995, 51, 887–897 CrossRef.
-
V. A. Bloomfield, D. M. Crothers and I. Tinoco, Physical chemistry of nucleic acids, Harper & Row, New York, 1974 Search PubMed.
- R. Esteghamat-Panah, H. Hadadzadeh, H. Farrokhpour, J. Simpson, A. Abdolmaleki and F. Abyar, Eur. J. Med. Chem., 2017, 127, 958–971 CrossRef CAS PubMed.
- N. Nanjundan, P. Selvakumar, R. Narayanasamy, R. A. Haque, K. Velmurugan, R. Nandhakumar, T. Silambarasan and R. Dhandapani, J. Photochem. Photobiol., B, 2014, 141, 176–185 CrossRef CAS PubMed.
- E. Alarcon, A. Aspée, M. Gonzalez-Bejar, A. Edwards, E. Lissi and J. Scaiano, Photochem. Photobiol. Sci., 2010, 9, 861–869 CAS.
- N. S. Quiming, R. B. Vergel, M. G. Nicolas and J. A. Villanueva, J. Health Sci., 2005, 51, 8–15 CrossRef CAS.
- G. M. Morris, R. Huey, W. Lindstrom, M. F. Sanner, R. K. Belew, D. S. Goodsell and A. J. Olson, J. Comput. Chem., 2009, 30, 2785–2791 CrossRef CAS PubMed.
- G. M. Morris, D. S. Goodsell, R. S. Halliday, R. Huey, W. E. Hart, R. K. Belew and A. J. Olson, J. Comput. Chem., 1998, 19, 1639–1662 CrossRef CAS.
- C. Hetényi and D. van der Spoel, Protein Sci., 2002, 11, 1729–1737 CrossRef PubMed.
- C. Hetényi and D. van der Spoel, FEBS Lett., 2006, 580, 1447–1450 CrossRef PubMed.
- N. Bordbar, M. H. Karimi and Z. Amirghofran, Cell. immunol., 2012, 280, 44–49 CrossRef CAS PubMed.
- M. Asadi, Z. Asadi, L. Zarei, S. B. Sadi and Z. Amirghofran, Spectrochim. Acta, Part A, 2014, 133, 697–706 CrossRef CAS PubMed.
- M. Asadi, Z. Asadi, S. B. Sadi, L. Zarei, F. M. Baigi and Z. Amirghofran, Spectrochim. Acta, Part A, 2014, 122, 118–129 CrossRef CAS PubMed.
- A. Koch, P. Tamez, J. Pezzuto and D. Soejarto, J. Ethnopharmacol., 2005, 101, 95–99 CrossRef CAS PubMed.
- M. A. Ali, A. H. Mirza, R. J. Butcher, M. T. H. Tarafder, T. B. Keat and A. M. Ali, J. Inorg. Biochem., 2002, 92, 141–148 CrossRef.
- K. Karami, M. H. Kharat, C. Rizzoli and J. Lipkowski, J. Organomet. Chem., 2013, 728, 16–22 CrossRef CAS.
- V. T. Yilmaz, S. Caglar and W. T. Harrison, Z. Anorg. Allg. Chem., 2004, 630, 948–951 CrossRef CAS.
- O. Quinzani, S. Tarulli, O. Piro, E. J. Baran and E. Castellano, Z. Naturforsch., B: Chem. Sci., 1997, 52, 183–187 CrossRef CAS.
- P. Naumov and G. Jovanovski, Curr. Org. Chem., 2001, 5, 1059–1077 CrossRef CAS.
- P. Naumov and G. Jovanovski, J. Mol. Struct., 2001, 563, 335–339 CrossRef.
- J. Luo, J. R. Khusnutdinova, N. P. Rath and L. M. Mirica, Chem. Commun., 2012, 48, 1532–1534 RSC.
- M. D. Santana, R. García-Bueno, G. García, G. Sánchez, J. García, A. R. Kapdi, M. Naik, S. Pednekar, J. Pérez and L. García, Dalton Trans., 2012, 41, 3832–3842 RSC.
- J. L. Serrano, J. Pérez, L. García, G. Sánchez, J. García, K. Tyagi and A. Kapdi, RSC Adv., 2012, 2, 12237–12244 RSC.
- J. Pérez, L. García, E. Pérez, J. L. Serrano, J. F. Martínez, G. Sánchez, G. López, A. Espinosa, M. Liu and F. Sanz, New J. Chem., 2003, 27, 1490–1496 RSC.
-
L. Pauling, The nature of the chemical bond and the structure of molecules and crystals: an introduction to modern structural chemistry, Cornell University Press, 1960 Search PubMed.
- J. G. Delis, P. G. Aubel, K. Vrieze, P. W. van Leeuwen, N. Veldman and A. L. Spek, Organometallics, 1997, 16, 4150–4160 CrossRef CAS.
- K. Karami, M. Hosseini-Kharat, C. Rizzoli, H. Tavakol and J. Lipkowski, J. Organomet. Chem., 2014, 752, 152–160 CrossRef CAS.
- P. Naumov, G. Jovanovski, S.-Z. Hu, I.-H. Suh, I. A. Razak, S. Chantrapromma, H.-K. Fun and S. W. Ng, Acta Crystallogr., Sect. A: Found. Crystallogr., 2001, 57, 1016–1019 CAS.
- R. Loganathan, S. Ramakrishnan, M. Ganeshpandian, N. S. Bhuvanesh, M. Palaniandavar, A. Riyasdeen and M. A. Akbarsha, Dalton Trans., 2015, 44, 10210–10227 RSC.
- K. Karami, M. Alinaghi, Z. Amirghofran and J. Lipkowski, Inorg. Chim. Acta, 2017 DOI:10.1016/j.ica.2017.02.027.
- A. Wolfe, G. H. Shimer Jr and T. Meehan, Biochemistry, 1987, 26, 6392–6396 CrossRef CAS PubMed.
- C. Icsel and V. T. Yilmaz, DNA Cell Biol., 2013, 32, 165–172 CrossRef CAS PubMed.
- Z. Shokohi-pour, H. Chiniforoshan, A. A. Momtazi-borojeni and B. Notash, J. Photochem. Photobiol., B, 2016, 162, 34–44 CrossRef CAS PubMed.
- L. Z. Zhang and P. Cheng, J. Inorg. Biochem., 2004, 98, 569–574 CrossRef CAS PubMed.
- M. Bordbar, M. Tabatabaee, M. Alizadeh-Nouqi, Z. Mehri-Lighvan, H. R. Khavasi, A. YeganehFaal, F. Fallahian and M. Dolati, J. Iran. Chem. Soc., 2016, 1–8 Search PubMed.
- M. Bordbar, M. Tabatabaee, A. Yeganeh Faal, Z. Mehri Lighvan and R. Fazaeli, Synth. React. Inorg. Met.-Org. Chem., 2015, 45, 1882–1888 CrossRef CAS.
- L. Z. Zhang and P. Cheng, Inorg. Chem. Commun., 2004, 7, 392–394 CrossRef CAS.
- K. Karami, Z. M. Lighvan, S. A. Barzani, A. Y. Faal, M. Poshteh-Shirani, T. Khayamian, V. Eigner and M. Dušek, New J. Chem., 2015, 39, 8708–8719 RSC.
- M. Anjomshoa, H. Hadadzadeh, S. J. Fatemi and M. Torkzadeh-Mahani, Spectrochim. Acta, Part A, 2015, 136, 205–215 CrossRef CAS PubMed.
- W. Villarreal, L. Colina-Vegas, C. Rodrigues de Oliveira, J. C. Tenorio, J. Ellena, F. C. Gozzo, M. R. Cominetti, A. G. Ferreira, M. A. B. Ferreira and M. Navarro, Inorg. Chem., 2015, 54, 11709–11720 CrossRef CAS PubMed.
- N. Wang, L. Ye, B. Zhao and J. Yu, Braz. J. Med. Biol. Res., 2008, 41, 589–595 CrossRef CAS PubMed.
- R. Esteghamat-Panah, H. Farrokhpour, H. Hadadzadeh, F. Abyar and H. A. Rudbari, RSC Adv., 2016, 6, 23913–23929 RSC.
- K. Karami, S. Hashemi, J. Lipkowski, F. Mardani, A. A. Momtazi-borojeni and Z. M. Lighvan, Appl. Organomet. Chem., 2017 DOI:10.1002/aoc.3740.
- X.-F. Zhao, Y. Ouyang, Y.-Z. Liu, Q.-J. Su, H. Tian, C.-Z. Xie and J.-Y. Xu, New J. Chem., 2014, 38, 955–965 RSC.
- A. Sułkowska, J. Mol. Struct., 2002, 614, 227–232 CrossRef.
- M. Van De Weert, J. Fluoresc., 2010, 20, 625–629 CrossRef CAS PubMed.
- J. Keizer, J. Am. Chem. Soc., 1983, 105, 1494–1498 CrossRef CAS.
- Y.-J. Hu, Y. Liu, J.-B. Wang, X.-H. Xiao and S.-S. Qu, J. Pharm. Biomed. Anal., 2004, 36, 915–919 CrossRef CAS PubMed.
- J. R. Lakowicz and G. Weber, Biochemistry, 1973, 12, 4161–4170 CrossRef CAS PubMed.
- S. Lehrer, Biochemistry, 1971, 10, 3254–3263 CrossRef CAS PubMed.
-
R. L. Joseph and R. Lakowicz, 1999.
- L. A. Sklar, B. S. Hudson and R. D. Simoni, Biochemistry, 1977, 16, 5100–5108 CrossRef CAS PubMed.
-
S. A. Hussain, arXiv preprint arXiv:0908.1815, 2009.
- T. Főrster, Discuss. Faraday Soc., 1959, 27, 7–17 RSC.
- F. Deng and Y. Liu, J. Lumin., 2012, 132, 443–448 CrossRef CAS.
- Y. Yue, X. Chen, J. Qin and X. Yao, J. Pharm. Biomed. Anal., 2009, 49, 753–759 CrossRef CAS PubMed.
- Y.-J. Hu, Y. Liu, L.-X. Zhang, R.-M. Zhao and S.-S. Qu, J. Mol. Struct., 2005, 750, 174–178 CrossRef CAS.
- W. He, Y. Li, C. Xue, Z. Hu, X. Chen and F. Sheng, Bioorg. Med. Chem., 2005, 13, 1837–1845 CrossRef CAS PubMed.
- Y. Song, Y. Liu, W. Liu, F. A. Villamena and J. L. Zweier, RSC Adv., 2014, 4, 47649–47656 RSC.
- I. Sjöholm, B. Ekman, A. Kober, I. Ljungstedt-Påhlman, B. Seiving and T. Sjödin, Mol. Pharmacol., 1979, 16, 767–777 Search PubMed.
- M. Rakad and A. Jumaily, Iraqi Journal of Cancer and Medical Genetics, 2010, 3, 20–23 Search PubMed.
- M. P. Heng, S. K. Sinniah, W. Y. Teoh, K. S. Sim, S. W. Ng, Y. K. Cheah and K. W. Tan, Spectrochim. Acta, Part A, 2015, 150, 360–372 CrossRef CAS PubMed.
- R. M. Vaden, K. M. Gligorich, R. Jana, M. S. Sigman and B. E. Welm, Breast Cancer Res., 2014, 16, 472 CrossRef PubMed.
- L. R. Kelland, B. A. Murrer, G. Abel, C. M. Giandomenico, P. Mistry and K. R. Harrap, Cancer Res., 1992, 52, 822–828 CAS.
- J. L. Hickey, R. A. Ruhayel, P. J. Barnard, M. V. Baker, S. J. Berners-Price and A. Filipovska, J. Am. Chem. Soc., 2008, 130, 12570–12571 CrossRef CAS PubMed.
- V. Zuco, R. Supino, S. C. Righetti, L. Cleris, E. Marchesi, C. Gambacorti-Passerini and F. Formelli, Cancer Lett., 2002, 175, 17–25 CrossRef CAS PubMed.
Footnote |
† Electronic supplementary information (ESI) available: Fig. S1: 1H NMR spectra of the ligand LH in DMSO-d6. Fig. S2: 1H NMR spectra of complex 1 in DMSO-d6. Fig. S3: 13C {1H} NMR spectra of the ligand LH in DMSO-d6. Fig. S4: 13C {1H} NMR spectra of complex 1 in DMSO-d6. Fig. S5: in vitro cytotoxic activity of sodium saccharin (A), ligand LH (B) and complex 1 (C) against HeLa, A549 and MCF-7 tumor cell lines. Fig. S6: in vitro cytotoxic activity of complex 1 against NIH normal fibroblast cells. CCDC 1522173. For ESI and crystallographic data in CIF or other electronic format, see DOI: 10.1039/c7nj03138f |
|
This journal is © The Royal Society of Chemistry and the Centre National de la Recherche Scientifique 2018 |
Click here to see how this site uses Cookies. View our privacy policy here.