DOI:
10.1039/C7FO01371J
(Paper)
Food Funct., 2018,
9, 191-199
Quillaja saponin-based hollow salt particles as solid carriers for enhancing sensory aroma with reduced sodium intake†
Received
3rd September 2017
, Accepted 22nd November 2017
First published on 22nd November 2017
Abstract
Dietary salt is a vital ingredient associated with sensory performance in processed foods, while reduced salt intake linked to public health is highly desired by consumers and food manufacturers. In this paper, quillaja saponin (QS) based hollow salt particles (∼10 μm) were fabricated by simple spray drying, and utilized as solid carriers to enhance sensory aromas with reduced sodium intake. QS-coated nanodroplets were firstly prepared as a reservoir for flavor oils (lemon and garlic oil), and then served as frameworks to construct hollow salt particles via general spray drying. Headspace gas chromatography-mass spectrometry (DHS-GC-MS) and panel sensory analysis conclude that the hollow salt particles loaded with flavor oils enhance typical aroma attributes and saltiness perception in comparison with their mixture control. The QS-based hollow salt particles could be developed into novel vehicles for improving flavor performance with reduced sodium intake, and furthermore used for delivery of hydrophobic bioactives in food systems.
Introduction
Dietary salt is a ubiquitous and critical ingredient for flavor, texture and preservation of foods that originated in prehistoric times. In the ancient world salt was amongst the most valuable traded and heavily taxed commodities, from which the English word “salary” is derived. Today, salt can be easily added at the table, during cooking or as a non-discretionary added into processed foods due to the GRAS status of salt. However, a large amount of dietary salt originates from processed foods, approximately 75–80% of adult daily dietary salt intake.1,2 It is highlighted that processed cereals (e.g., white bread and breakfast cereals), meats, dairy and snack products contribute significantly to excess salt intake.2,3 According to the World Health Organization (WHO), the worldwide average daily salt intake is currently from 9 to 12 g salt per day, and is well in excess of the recommended 5 g day−1.4 Excess intake of dietary salt is strongly linked to elevated blood pressure, and increased risk of stroke and cardiovascular outcomes. It has been estimated that up to 7.5 million deaths, accounting for 12.8% of all deaths globally, and contributing approximately 10% of global healthcare expenditure, are linked to excessive salt consumption.5 Government and health agencies consistently stress the need for immediate action to implement interventions to reduce dietary intake of salt.6 Therefore, a reduction in the amount of dietary salt is urgently required, and is especially desirable in the processed food sector. However, the key functional roles of salt in food manufacture arise from its roles not only as a preservative, but also as a flavor enhancer, binding agent, and texture modifier.7 Hence, salt/sodium reduction strategies must be compromised by food safety and maintaining their sensory acceptability to the consumer.8
Salt as a flavor enhancer has been reported to enable a higher intensity perception of the overall aroma of food such as cheeses, sausage, and bread.8,9 Keast et al.10 reported weak or near threshold stimuli results in the enhancement of overall flavor perception based on the effect of taste–taste interaction and taste–odor integration. Therefore, recent studies have been conducted to produce low salt/sodium foods by using salt-associated odors (e.g., cheese, umami and broth) to enhance the perceived intensity of saltiness (described as odor-induced saltiness enhancement (OISE)).11,12 The strategy of such flavor enhancers was open to opportunity to produce low sodium products with a high saltiness intensity. Alternately, salt reduction can be achieved by manipulating the microstructure and distribution of the salt without compromising the taste.3,13 Minter developed hollow salt microspheres based on amphiphilic biopolymers, such as gum acacia, that can reduce salt by 25–50% in certain applications (e.g., breads and salty snacks).14 The hollow microspheres with a high specific surface area, smaller size and good free-flow property might increase the accessibility or efficiency of sodium chloride to the target receptor on the tongue bud, thus resulting in enhancement of saltiness perception.
The objective of this study is to develop a novel salt reduction strategy that integrates a flavor enhancer and hollow superstructure approach, and thus attempts to establish the hollow salt particles based vehicle system for delivery of aroma molecules in a food system. In our previous research, we found that Quillaja saponin (QS), a natural small-molecule surfactant, has the capacity to encapsulate flavor oils into nanoemulsion droplets,15 and the resulting nanodroplets can be utilized as efficient stabilizers to fabricate stable high inner phase emulsions (HIPEs)16 and aqueous foams.17 In this work, QS-coated nanodroplets were first prepared as a reservoir for flavor oils (lemon oil and garlic oil), and then served as a stabilizer to construct hollow salt particles through general spray-drying. The microstructure of the hollow particles was characterized by scanning electron microscopy (SEM), confocal laser scanning microscopy (CLSM) and energy dispersive spectroscopy (EDS). The aroma release profiles in hollow salt particles were monitored by dynamic headspace gas chromatography flame ionization detection (DHS-GC-FID) and dynamic headspace gas chromatography-mass spectrometry (DHS-GC-MS). Panel sensory analysis with real foods (French fries and peanuts) was also conducted to evaluate the flavor and saltiness perception in humans.
Materials and methods
Materials
Quillaja saponin (QS), which is a triterpene-glycoside extracted from the bark of Quillaja saponaria Molina tree, was kindly provided by Ingredion Inc. (Westchester, IL, USA). Table salt was purchased from a local supermarket (Guangzhou, China). Cold-pressed lemon oil (containing approximately 58% limonene, 19% camphene and other minor components) and garlic oil (purity >90%, containing three major constituents, about 58.6% of diallyl disulphide, 15.4% of diallyl trisulphide, 6.7% of diallyl sulfide and other minor components), stored at 4 °C, were kindly provided by Kaihong Flavor Co., Ltd (Guangzhou, Chain). Nile red was purchased from Sigma Chemical Co. (St Louis, MO, USA). Other chemicals were of analytical grade.
Fabrication of QS-coated nanodroplets
QS-coated nanodroplets were prepared according to an ultrasonic emulsification protocol we reported previously.15 Briefly, an aqueous phase containing QS was prepared by dispersing 15 mg g−1 saponin in aqueous buffer solution (10.0 mM phosphate buffer, pH 7.0). Then, the aqueous phase with 10% flavor oil (i.e., lemon oil, garlic oil) was sonicated using an ultrasound probe (VCX 500, Sonics and Materials Inc., Newtown USA) for 300 s at 70% maximum tip oscillation amplitude under ice cooling. Previous results revealed that the average droplet size of the QS-coated nanodroplets was approximately 154 nm with fairly monodisperse (PDI = 0.20) when the QS concentration was up to 15 mg g−1.16,17
Preparation and characterization of hollow salt particles
The hollow salt particles were produced via the spray-drying process using a Büchi B-290 mini spray-drier (BUCHI Labortechnik AG, Flawil, Switzerland). The values for the solid contents of the multicomponent mixtures are related to the solid contents of 25 wt% table salt and 10 wt% flavor oil-in-water emulsion (equivalent to 1.0 wt% nanodroplets). The mixtures were fed into the main chamber through a peristaltic pump operating at 150 °C for the inlet air temperature and ∼75 °C for the outlet temperature. For the flavor oil powder, the single flavor oil-in-water emulsion stabilized by saponin was spray-dried and separated with a cyclone. These dry powders were collected and stored in hermetically sealed polyethylene containers in a desiccator at room temperature until analysis.
Particle size analysis.
The particle size distribution of samples was determined using a Horiba particle size distribution analyzer (LA960DRY, Software LA-960, Horiba Ltd, Kyoto, Japan). The calculation is based on the Mie theory allowing particle detection within a range of 10 nm to 3000 μm. A measurement was performed with the following parameters: air, 0.40 MPa; feeder intensity (0–200), 80; relative refractive index, 1.50. The distribution values were the average of 3 determinations of each sample.
Scanning electron microscopy (SEM).
The microstructure of the hollow salt was characterized using SEM. Spray-dried samples were fixed to the sample holder using double-sided tape and underwent gold-sputtered under vacuum to produce an electrically conductive surface and placed in a tabletop SEM (Hitachi TM 3000, Japan).
Confocal laser scanning microscopy (CLSM).
The spray-dried samples were visualized using a Zeiss LSM 510 Meta microscope (LSM700, Carl Zeiss, Oberkochen, Germany) with an inverted 100× oil immersion objective. To prepare visible QS-coated nanodroplets, 5 mg of Nile red was dissolved in 10 g of flavor oil to obtain a saturated organic phase, and then QS nanodroplets based hollow salt particles were prepared and observed with a argon/krypton laser (ArKr, 488 nm).
Energy dispersive spectroscopy (EDS).
EDS was performed using a scanning electronic microscope (EVO 18, Zeiss, Germany) coupled with energy dispersive spectroscopy (Zeiss EVO MA 10 and Zeiss MERLIN FE-SEM, Carl Zeiss AG, Oberkochen, Germany) to determine the elemental distribution of the surface of the hollow salt particles. The distribution values were the average of 3 determinations of each sample.
Aroma release of hollow salt particles
The headspace volatiles of flavored salt powders were determined in combination with DHS-GC-MS and DHS-GC-FID, for the identification and semiquantification of volatile compounds according to our recently reported method.15 Briefly, aliquots of fresh powders (0.1 g, based on the flavor oil effective content) were pipetted into a 20 mL headspace vial. The vial was sealed and equilibrated at 60 °C for 20 min at a rotating speed of 450 rpm in the heating chamber of the autosampler (CTC Analytics AG, Zwingen, Switzerland). 1.5 mL of the headspace vapor was directly transferred into the GC unit (Agilent 7890B, USA) equipped with a HP-5 capillary column (30 m × 0.25 mm i.d.; 0.25 μm film thickness) and connected with a flame ionization detector (FID). The FID temperature was set at 250 °C. The oven temperature profile was programmed as follows: a 10 °C min−1 increment from 35 °C (held for 1 min) to 50 °C in the first stage, and then increased to 100 °C (held for 5 min) at the rate of 10 °C min−1, then a further increase to 200 °C (held for 5 min) at the rate of 15 °C min−1 and finally an increase to 230 °C at the rate of 15 °C min−1 with a hold at 230 °C for 3 min. The flow rate of hydrogen as the flame gas was controlled at 35.0 mL min−1, air flow at 300 mL min−1, and nitrogen as the carrier gas flow at 1.0 mL min−1 under split mode (1
:
1).
GC-mass analysis of volatiles.
Analysis of aroma components was performed using a GC unit (Agilent 7890B, USA) equipped with a mass spectrometric detector (MSD), model HP 5977A. The column used was a HP-5MS, 30 m × 0.25 mm × 0.25 μm (Agilent Technologies, catalog number 122-5532). The temperature programming and carrier gas flow rates were kept the same as for the above-described GC measurements. The mass spectrometer was operated in the electron impact mode with an electron energy of 70 eV; source temperature, 230 °C; quadrupole temperature, 150 °C; mass range m/z 30–500; scan rate, 3.62 s per scan; and EM voltage, 1150. The individual compounds were identified by both MS-library searches (Wiley138 K, John Wiley and Sons, Hewlett Packard, USA) and retention time (RT) against authentic external standards.
Panel sensory analysis
All sensory analyses were performed in two different sessions in a sensory panel room. The panel consisted of 11 subjects (6 women and 5 men, aged 18–36 years) with no history of known taste or smell disorders who had given informed consent to participate in the sensory tests. Prior to the analysis of the different flavor profiles, the 11 panelists participated in the development of a descriptive vocabulary that best described the sensory attributes associated with the samples and agreed upon a standard sample assessment procedure (ISO 8586).18 In two subsequent sessions, these terms were refined and reduced to the five final descriptors: saltiness, lemon/garlic aroma, French fries odor/fried peanut odor, dispersibility, and oral grainy. At the beginning the panelists were accustomed to evaluating representative samples by learning to distinguish between two different samples of fries containing 2.0 and 4.0% salt (and two different samples of fries containing 0.1 and 0.2% lemon flavor powder) and more to increase their sensitivity and ability to discriminate between the sensory attributes. Moreover, the panelists were trained during 12 h sessions to score their flavor and texture perceptions on a linear scale from 0 to 10 (0 = none, 10 = extremely strong) according to Table S1,† which shows the sensory descriptors with their definitions and references. The training samples were evaluated in duplicate over two consecutive days in the sensory laboratory to assess panel performance.
Sample preparation and sensory analyses.
Fried potato chips and fried peanuts were homemade and individually prescreened by hand to uniform size and shape. 100 g potato fries or peanuts placed in a basket were fried in 1000 mL edible oil for approximately 4 min at 180 °C in a batch fryer. The amount of salt needed to achieve a salt/sample ratio of 1
:
40 was calculated for each of the three salt fractions (2.5 g, in salt effective content). Salt was gradually sprinkled over the fries or peanuts in the bags, while gently shaking to ensure an even distribution throughout the bag. The samples were tested in sensory panel rooms under double blinded and standardized conditions, and a fully randomized presentation design among product, panelist, and replications was chosen. A covered sensory flask encrypted by a three-digit random number was filled with six pieces of fries or peanuts, and the panelists were asked to rank and score the attributes saltiness, lemon/garlic aroma, French fries odor/fried peanut odor, dispersibility, and oral grainy on a scale of 0–10. The comparative sensory test was independently run three times with two different flavors.
Statistical analysis
All of the data were analyzed by SPSS 19.0 and expressed as the mean ± standard deviation (SD). Statistical analysis was performed using one way analysis of variance (ANOVA) followed by Duncan's multiple range test (DMRT). P values <0.05 were considered as significant.
Results and discussion
Preparation and characterization of hollow salt particles
Typically, hollow salt particles were fabricated by using a spray-drying process with a solution created from the table salt solution in the presence of the biosurfactant QS. Fig. 1(a) is a photo of a spray-dried salt powder from a solution containing of 25% salt and 1.0% QS-coated nanodroplets, it shows the resulting product remains free-flowing and has a non-agglomerating property under ambient conditions. Moreover, this product also shows rapid dissolution behavior and can be readily converted into a stable colloid which is well-dispersed in water (see ESI Fig. S1 and Movie S1†). Particle size distribution of the resultant product was obtained by light scattering measurements (Fig. 1(b)), a salt powder that was relatively monodispersed and has a small average size (about 10.58 μm) was observed. In this study, approximately 90% by volume of the particles were below 16 μm, much smaller than that of the salt particles prepared by using 30% salt and 2.0% gum acacia.14 Generally, table salt exhibits a cube crystals morphology with larger size (400–600 μm), while spray-dried salts alone give large aggregates as well due to the re-agglomerating of small salt crystals (Fig. S2(a and b)†). In spite of the small size, it was found that the salt product was essentially non-agglomerating and capable of remaining as a free-flowing solid for a long-time period in excess of 120 days. Salt particles with a smaller size and bigger specific surface area should mean that the same “seasoning level” may be achieved using a lower amount of salt. Fig. 1(c and d) are the SEM images of spray-dried salt particles in the presence of QS and QS-coated nanodroplets respectively, and show the typical hollow structure having a shell formed by individual crystallites of salt. QS, a triterpenoid saponin, can form highly elastic–viscous interfacial layers at the air–water interface, resulting in dense packing amongst the molecules in the adsorption layer.19,20 Based on the unique self-assembly behavior of triterpenoid saponin at the air–water and oil–water interface,21 we have developed QS-coated nanodroplets as efficient stabilizers to fabricate stable HIPEs16 and aqueous foams.17 In this study, we attempt to fabricate generally spherical hollow salt particles based on QS-coated nanodroplets by simple spray drying. QS and its coated nanodroplets might guide the crystallization of salt at the air–liquid interface, and facilitate salt hollowing events. The observations indicate that a higher nanodroplets concentration (about 2.0%) gives smaller salt particles (Fig. 2(a and b)); while excess nanodroplets concentration (about 10%) led to the formation of hollow salt particles with melting salt shells rather than regular salt crystals (Fig. 2(c and d)).
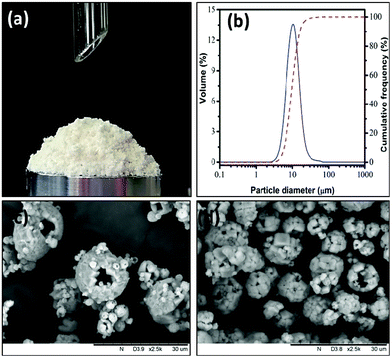 |
| Fig. 1 (a) Free-flowing property, (b) particle size distribution, (c) SEM image of hollow salt particles from 25% salt solution and 1.0% QS alone; and (d) SEM image of hollow salt particles from 25% salt solution and 1.0% QS-coated nanodroplets. | |
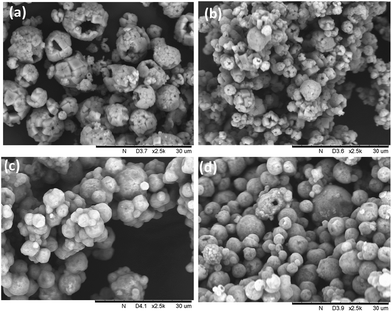 |
| Fig. 2 SEM micrographs of the hollow salt particles from 25% salt solution with 1.0% (a) and 2.0% QS-coated nanodroplets (b); and from 10.0% QS-coated nanodroplets with 10% (c) and 30% salt solution (d). | |
A magnifying hollow salt particle shown in Fig. 3(a) clearly demonstrates the crystal shell architecture formation assembled from the smaller rhomboid salt crystals. Fig. 3(b) is a CLSM image of a single hollow salt particle with similar size, and shows the QS-coated nanodroplets (dyed green by Nile Red) actually located at the outer surface of the salt crystal shell. In order to clarify the possible formation mechanism of the hollow salt particle, we further determined the elemental distribution of the surface of hollow salt particles by using EDS spectra. The EDS spectra allow for good detection of distribution of Cl and Na, as well as C, O and S (Fig. S3†). The former (Cl and Na) are existing elements in salt samples; while the latter (C, O and S) mainly originated from the QS-coated garlic oil nanodroplets. Table 1 compares the EDS atomic percentage attributes among four salt products: normal salt, mixture of salt and oil powder, and hollow salt by QS nanodroplets with two concentrations (1.0 and 2.0%, respectively). It can be seen that for hollow salt products the atomic percentage of C, O and S (from QS-coated garlic oil nanodroplets) increased while the relative distribution of both Na and Cl deceased significantly, in comparison with both normal salt and the salt/oil powder mixture. Specifically, for hollow salt product prepared by 25% salt and 2.0% nanodroplets, the percentage of Na and Cl are 21.39% and 21.79% respectively, much lower than that of normal salt (32.73% and 59.47%) and salt/oil powder mixture (29.61% and 41.13%). This is in agreement with the CLSM result, and suggested that the evaporation drove migration of QS coated nanodroplets into the air–liquid interface during spray drying.
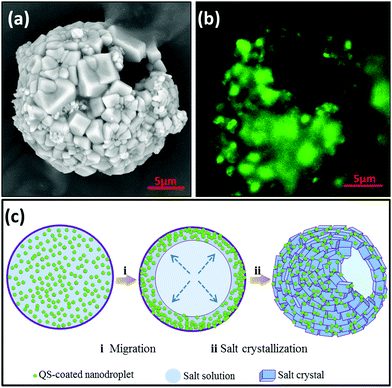 |
| Fig. 3 (a) SEM and (b) CLSM micrographs of hollow salt particles fabricated from 25% salt solution and 1.0% QS-coated nanodroplets; (c) schematic illustration shows the possible formation mechanism of the hollow salt particles. | |
Table 1 EDS atomic percentages (%) of carbon (C), oxygen (O), sodium (Na), chlorine (Cl) and sulfur (S) attributed to the salt productsa
Samples |
Normal salt |
Mixture |
Hollow salt1 |
Hollow salt2 |
Mixture, mixture of normal salt and garlic oil powder; Hollow salt1, hollow salt fabricated from 25% salt and 1.0% QS-coated garlic oil nanodroplets; Hollow salt2, hollow salt fabricated from 25% salt and 2.0% QS-coated garlic oil nanodroplets. Mean values ± standard deviations of triplicate determinations. Different letters (a–d) in the same column indicate significant differences between salts (P < 0.05). C, O and S peaks of normal salt are associated background signal of the sample holder.
|
C |
7.54 ± 0.48% d |
27.09 ± 1.12% c |
39.52 ± 1.01% b |
49.84 ± 0.63% a |
O |
0.29 ± 0.09% d |
2.21 ± 0.27% c |
3.60 ± 0.06% b |
6.81 ± 0.31% a |
Na |
32.73 ± 0.54% a |
29.61 ± 0.48% b |
21.31 ± 0.98% c |
21.39 ± 0.29% c |
Cl |
59.47 ± 0.83% a |
41.13 ± 0.66% b |
35.54 ± 0.23% c |
21.79 ± 0.29% d |
S |
0.02 ± 0.01% b |
0.14 ± 0.07% ab |
0.21 ± 0.12% ab |
0.39 ± 0.06% a |
The spray-drying technique is a low-cost, rapid and scalable method that can be exploited for the production of controlled morphology particles from the nanometer to micrometer size ranges.22,23 In the case of structure formation using the spray-drying process, the evaporation and energy transport driven diffusional flow inside the atomized droplets leads to an increase of the solute/particle concentration on the air–liquid surface. The phenomenon was largely dependent on the surface activity and adsorption property of the solute/particles.24,25Fig. 3(c) shows the schematic illustration, showing the possible formation mechanism of the hollow salt particles. The process starts with atomization of a solution of the multicomponent precursors (containing sodium chloride and QS-coated nanodroplets) into a spray of droplets. As the evaporating droplet shrinks, the evaporation-induced diffusional flow will drive the nanodroplets preferred diffuse and concentrate at the air–liquid surface during drying, attributing to their surface activity.15,19 According to our previous study, QS nanodroplets can be irreversibly adsorbed at the air–liquid interface and exhibited less surface desorption and a high elastic–viscous response to a large mechanical deformation, probably via the formation of self-assembled fibrillar networks of QS.15 Thus, this could supply frameworks for salt localized crystallization on the surfaces of atomized droplets, which means the formed salt crystals have limited mobility resulting in accumulation at the surface and therefore merge into a well-packed shell. In the case of the confining crystallization of salt, the fabrication of a novel carrier with a hollow structure that combines the properties of salt and flavor oil nanodroplets is promising to enhance the aroma intensity.
Enhanced aroma release intensity
Due to the size of the particles and large specific surface, the hollow superstructure has long been recognized as an effective way for delivery or encapsulation of aromas, drugs or other active ingredients, which is important for enhanced application properties of high-quality products in the food, pharmaceutical, and chemical industries.26 In this study, QS-coated nanodroplets were firstly prepared as a reservoir for model flavor oils (lemon and garlic oil), and then utilized to prepare hollow salt particles. The enhancement of typical aromas is evaluated by using DHS-GC-FID combined with DHS-GC-MS. A total of twelve volatiles were identified by chromatographic analysis in the various lemon oil and salt powder formulations (see ESI Fig. S4(a)†), and the intensity of the aromas is summarized in Table 2. The adding of salt had substantial effects on the entire flavor profile because it not only led to enhancement of the lemony and citrusy aroma itself, but also led to an increased perception of flavor attributes such as pine-like, sweet, musty and resinous. These findings correlate well with the literature, which has shown that salt has a significant influence on the temporal release of volatiles that is dependent not only on the salt concentration, but also on the hydrophobicity of the volatile compounds.27 Comparing the single lemon oil powder and the lemon oil powder/salt mixture, a significant enhancement in the intensity for the hollow salt particles was determined for octanal, α-terpinene, linalool, limonene, γ-terpinene, neral and geranial; these compounds were highly associated with the characteristic flavor of lemon oil.28 Taking linalool and limonene as examples, 13.87 and 5.01 times higher aroma density was found for the hollow salt particles, respectively, than that of the lemon oil powder control. For traditional seasoning formulation, the liquid flavor oils were generally preferred to mixing with the solid carrier (e.g. salt, fibers, starch and even silica particles), for easy handling, storage and transportation. However, this always led to agglomeration of particles, low encapsulation efficiency and bad stability. In the case of hollow salt particles, flavor oils were first packed into nanodroplets with a good encapsulation efficiency and oxidative stability,15 and these nanodroplets were spatially distributed to the outer surface of the hollow salt particles (Fig. 3). Accordingly, this might enhance the mass transfer of aroma molecules and subsequent perception of lemony volatiles.
Table 2 Intensity and relative amounts (brackets to control) of aroma active compounds various lemon oil powder formulationsa
No. |
Retention time (min) |
Compounds |
Lemon oil powder |
Mixture |
Hollow salt1 |
Hollow salt2 |
Sensory attributesb |
The percentage in brackets volatiles of the total for the component content. Relative abundances of volatile compounds of lemon oil powder formulations; Mixture, mixture of normal salt and lemon oil powder; Hollow salt1, hollow salt fabricated from 25% salt and 1.0% QS-coated lemon oil nanodroplets; Hollow salt2, hollow salt fabricated from 25% salt and 2.0% QS-coated lemon oil nanodroplets.
Odor descriptions are based on (I) the University of Florida Citrus Flavor database (http://www.crec.ifas.ufl.edu/rouseff/#) and (II) Flavornet (http://www.flavornet.org/flavornet.html).
The total scale of overall lemon aroma ranged from 0 (not perceivable) to 100 (strongly perceivable).
Not detected.
|
1 |
9.122 |
Sabinene |
6.162 (100) |
18.293 (296.75) |
12.877 (208.97) |
11.289 (183.20) |
Warm, oily, peppery, green |
2 |
9.255 |
β-Pinene |
78.001 (100) |
248.279 (318.30) |
116.913 (149.89) |
90.505 (116.04) |
Pine-like, resinous |
3 |
9.552 |
α-3-Carene |
5.336 (100) |
18.869 (353.62) |
8.070 (151.24) |
7.687 (144.06) |
Sweet |
4 |
10.056 |
Myrcene |
27.210 (100) |
81.491 (299.49) |
53.238 (195.66) |
56.665 (208.25) |
Musty, wet soil |
5 |
10.133 |
Camphene |
304.911 (100) |
999.360 (327.75) |
502.619 (164.84) |
465.613 (152.70) |
Warm, oily, camphoraceous |
6 |
10.450 |
Octanal |
— d |
7.681 |
27.331 |
24.632 |
Pungent orange |
7 |
10.958 |
α-Terpinene |
2.015 (100) |
2.349 (116.5) |
2.465 (122.33) |
5.864 (291.02) |
Lemony, citrusy |
8 |
11.189 |
Linalool |
1.947 (100) |
9.468 (486.28) |
22.006 (1130.25) |
27.011 (1387.31) |
Floral, lavender |
9 |
11.287 |
Limonene |
417.289 (100) |
1152.414 (276.17) |
2096.731 (502.46) |
2094.376 (501.90) |
Citrus-like, fresh |
10 |
12.035 |
γ-Terpinene |
24.747 (100) |
67.944 (274.55) |
147.961 (597.89) |
142.409 (575.46) |
Lemony, lime-like |
11 |
12.888 |
Neral |
— |
— |
19.378 |
19.301 |
Lemony, citrusy |
12 |
17.436 |
Geranial |
— |
— |
33.383 |
60.723 |
Lemon oil, lemon cleaner |
|
Sensory scores c |
28.833 (100) |
38.667 (134.10) |
57.833 (200.58) |
76.667 (265.896) |
|
Similarly, Table 3 derived from Fig. S4(b)† shows the relative aroma intensity of the eight volatiles identified in different garlic oil and salt powder formulations by DHS-GC-MS. The results were in good agreement with previously reported key odorants in garlic oil.29 Among them, it is verified that diallyl sulfide (6.7%), diallyl disulphide (58.6%) and diallyl trisulfide (15.4%) constitute the dominant contributes to the overall aroma of garlic. In terms of the relative amount of volatiles compared with garlic oil powder, volatiles intensity for the mixture of normal salt and oil powder was enhanced by about 195% for diallyl disulphide. Furthermore, as compared with the volatile constituents of the mixture, a higher level of volatiles was detected by hollowing (enhanced by 205.17% and 137.53% for diallyl disulphide and diallyl trisulfide, respectively). These enhancements are partially due to the fact that these components are changing the effective area for mass transfer of volatile compounds, which was further confirmed by increasing the relative salt content in the preparation of hollow salt delivery vehicles. The main components, diallyl disulphide and diallyl trisulfide had relatively significant enhancement, specifically, and increased by 336.81% and 310.18% respectively in comparison with the garlic oil powder alone. This observation is in agreement with the results of the hollow salt enhancing lemon intensity, and the results are in line with evidence from interfacial mass transfer mechanisms.30 As expected, sensory evaluation further verified hollow salt particles as significant flavor vehicles in fullness aroma-related attributes of both lemon and garlic perception (P < 0.05) (see Tables 2 and 3). Together, these results indicate that QS-based hollow salt particles as novel carriers have a capability of enhancing aroma sensory.
Table 3 Intensity and relative amounts (brackets to control) of aroma active compounds various garlic oil powder formulationsa
No. |
Retention time (min) |
Compounds |
Garlic oil powder |
Mixture |
Hollow salt1 |
Hollow salt2 |
The percentage in brackets volatiles of the total for the component content. Relative abundances of volatile compounds of garlic oil powder formulations. Mixture, mixture of normal salt and garlic oil powder; Hollow salt1, hollow salt fabricated from 25% salt and 1.0% QS-coated garlic oil nanodroplets; Hollow salt2, hollow salt fabricated from 25% salt and 2.0% QS-coated garlic oil nanodroplets.
The total scale of overall garlic aroma ranged from 0 (not perceivable) to 100 (strongly perceivable).
Not detected.
|
1 |
2.899 |
Methyl allyl disulfide |
28.798 (100) |
41.495 (144.08) |
30.041 (104.32) |
38.508 (133.72) |
2 |
3.468 |
Methyl 2-propenyl disulfide |
1.840 (100) |
2.087 (113.42) |
1.817 (98.75) |
— c |
3 |
6.951 |
2,4-Dimethylfuran |
0.398 (100) |
1.000 (251.26) |
0.576 (144.72) |
0.573 (143.97) |
4 |
7.733 |
Diallyl sulfide |
86.553 (100) |
153.453 (177.29) |
45.618 (52.71) |
59.624 (68.89) |
5 |
10.141 |
Dimethyl trisulfide |
3.578 (100) |
1.436 (40.13) |
2.355 (65.82) |
1.056 (29.51) |
6 |
11.335 |
2-Vinyl-[4H]-1,3-dithiin |
7.861 (100) |
3.940 (50.12) |
6.616 (84.16) |
3.043 (38.71) |
7 |
12.629 |
Diallyl disulphide |
84.347 (100) |
165.151 (195.79) |
173.058 (205.17) |
284.093 (336.81) |
8 |
18.363 |
Diallyl trisulfide |
15.549 (100) |
14.012 (90.12) |
21.385 (137.53) |
48.230 (310.18) |
Sensory scores
|
31.666 (100)
|
42.166 (133.16)
|
53.333 (168.42)
|
67.500 (213.16)
|
Panel sensory analysis
We have developed a strategy to enhance aroma or odor intensity via manipulation of QS-coated nanodroplets based hollow salt particles. Subsequently, we attempted to conduct a panel sensory test for evaluating the salt reduction effect of the flavored hollow salt based on the OISE theory.11,12,31 The QS-coated nanodroplets based hollow salt that integrating a flavor enhancer and hollow superstructure approach might provide a fast, strong odor and saltiness perception, and thus enhance the overall salt perception. To test this, we designed a comparative sensory analysis so that the volatiles, saltiness and taste of different salt formulations were assessed simultaneously compared to the reference fries and peanuts at the same overall salt level of 2.5%. Five attributes including “saltiness”, “lemon/garlic aroma”, “French fries odor/fried peanut odor”, “dispersibility”, and “oral grainy” were used as descriptors by a trained panel. A spider plot of the average of each attribute from four salts is presented in Fig. 4(a and b). Firstly, it can be seen that even for a human perception test with real food system, the hollow salt particles always exhibited significant higher (P < 0.05) aroma scores (especially for garlic odors) than the flavor oil powder/salt mixture. The result is consistent with results obtained by DHS-GC analysis. Secondly, the impact of aromas on saltiness perception was demonstrated by higher saltiness scores when increasing the odors concentration in equal salt level. This observation allows us to conclude that the positive salt reduction effect of the QS-based hollow salt particles might contribute to the both the OISE strategy and hollow superstructure. The cross-modal interactions between the aroma and taste are more likely the expression of a cognitive mechanism, which has previously been described in other sensory studies to compensate for salt reduction in foods.12,32,33 For example, sulfur containing compounds in garlic oils were considered as key saltiness-enhancing compounds for saltiness reduction through multisensory-integration mechanisms.32 Additionally, Fig. 4(c) and (d) compare the surface morphology of normal salt and hollow salt on the peanuts, which were sprinkled with normal cube salt crystals (400–500 μm) and QS based hollow salt particles (10–20 μm); obviously the latter provide a better coating efficiency with smaller and separated particles. Therefore, the enhanced delivery rates of hollow salt microspheres can be explained by differential dissolution kinetics and mass transfer across the surface of the matrix to headspace/receptors.3,13 Additionally, reduction in the dissolution time of salt whilst chewing would boost the local concentration of the salt ions triggering a saltier perception.13
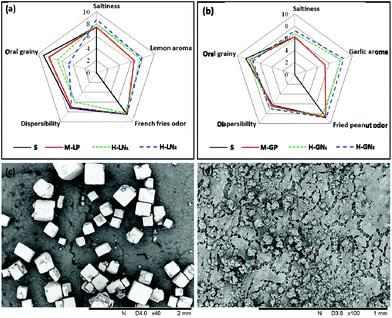 |
| Fig. 4 Sensory profiles of the French fries (a) and fried peanut (b) produced by salting normal salt (S), mixture of salt and lemon oil powder (M-LP), mixture of salt and garlic oil powder (M-GP), hollow salt particles loading 1.0% lemon oil nanodroplets (H-LN1), hollow salt particles loading 1.0% garlic oil nanodroplets (H-GN1), hollow salt particles loading 2.0% lemon oil nanodroplets (H-LN2), hollow salt particles loading 2.0% garlic oil nanodroplets (H-GN2); the physical morphology of salt and attachment to the salted peanut after adding normal salt (c) and hollow salt particles (d). | |
Conclusions
Presently, reducing the amount of salt in processed food without compromising taste by using an innovative ingredient solution is urgently required. To meet this demand, a novel hollow salt particle has been designed and successfully fabricated in a facile spray-drying protocol for enhancing aroma sensing with reduced sodium intake. The hollow salt particles have a structure comprised of individual crystallites of salt attached together for a shell formation stabilized by natural QS coated nanodroplets. This strategy of integrating a flavor enhancer and hollow superstructure enables the enhancement of overall saltiness perception and provides a new route for reducing the salt consumption in foods. Furthermore, a significant use of the hollow salt particles that are utilized as a solid carrier to delivery aroma sensing could be beneficial for formulating salt seasoning products. In this paper, common dietary salt can be developed into an efficient vehicle through controlling the particle size and morphologies with a low-cost, rapid and scalable spray-drying technology. Meanwhile, spray-drying technology could be used not only to create hollow superstructures, but also to incorporate functional molecules within or outside the cavities of these structures, thereby providing a new route to the design of complex ingredients. The QS based hollow salt particles are expected to be developed into novel vehicles, not only for improving flavor performance with reduced sodium intake, but also for delivery of hydrophobic bioactives in food systems.
Conflicts of interest
The authors declare that there are no conflicts to declare.
Acknowledgements
The authors would like to acknowledge the financial support of the National Natural Science Foundation of China (31371744), Science and Technology Planning project of Guangdong province (2016B090920082) and China Postdoctoral Science Foundation (2016M590788).
References
- E. Durack, M. Alonso-Gomez and M. G. Wilkinson, Salt: a review of its role in food science and public health, Curr. Nutr. Food Sci., 2008, 4, 290–297 CrossRef CAS
.
- M. E. Doyle and K. A. Glass, Sodium reduction and its effect on food safety, food quality, and human health, Compr. Rev. Food Sci. Food Saf., 2010, 9, 44–56 CrossRef CAS
.
- K. Konitzer, T. Pflaum, T. P. Oliveira, E. Arendt, P. Koehler and T. Hofmann, Kinetics of sodium release from wheat bread crumb as affected by sodium distribution, J. Agric. Food Chem., 2013, 61, 10659–10669 CrossRef CAS PubMed
.
-
WHO, in Guideline: Sodium intake for adults and children, World Health Organization (WHO), Geneva, Switzerland, 2012 Search PubMed
.
- I. Brown, I. Tzoulaki, V. Candeias and P. Elliott, Salt intakes around the world: Implications for public health, Int. J. Epidemiol. Infect. Dis., 2009, 38, 791–813 CrossRef PubMed
.
-
WHO, Reducing salt intake in populations: Report of a WHO Forum and Technical Meeting, 5-7 October 2006, Paris, France, WHO, Geneva, Switzerland
.
- R. A. Miller and R. C. Hoseney, Role of salt in baking, Cereal Foods World, 2008, 53, 4–6 CAS
.
- M. Dotsch, J. Busch, M. Batenburg, G. Liem, E. Tareilus, R. Mueller and G. Meijer, Strategies to reduce sodium consumption: A food industry perspective, Crit. Rev. Food Sci. Nutr., 2009, 49, 841–851 CrossRef CAS PubMed
.
- D. G. Liem, F. Miremadi and R. S. Keast, Reducing sodium in foods: the effect on flavor, Nutrients, 2011, 3, 694–711 CrossRef CAS PubMed
.
- R. S. Keast and P. A. Breslin, Cross-adaptation and bitterness inhibition of L-tryptophan, L-phenylalanine and urea further support for shared peripheral physiology, Chem. Senses, 2002, 27, 123–131 CrossRef PubMed
.
- G. Lawrence, C. Salles, C. Septier, J. Busch and T. Thomas-Danguin, Odour-taste interactions: A way to enhance saltiness in low-salt content solutions, Food Qual. Prefer., 2009, 20, 241–248 CrossRef
.
- N. Nasri, N. Beno, C. Septier, C. Salles and T. Thomas-Danguin, Cross-modal interactions between taste and smell: Odour-induced saltiness enhancement depends on salt level, Food Qual. Prefer., 2011, 22, 678–682 CrossRef
.
- M. Rutkevičius, G. H. Mehl, J. T. Petkov, S. D. Stoyanov and V. N. Paunov, Fabrication of salt-hydrogel marbles and hollow-shell microcapsules by an aerosol gelation technique, J. Mater. Chem. B, 2015, 3, 82–89 RSC
.
-
S. Minter, Salt product, WO2009/133409Al, 2009, W. I. P. Organization, World Intellectual Property Organization, US Search PubMed
.
- X. W. Chen, Y. J. Chen, J. M. Wang, J. Guo, S. W. Yin and X. Q. Yang, Phytosterol structured algae oil nanoemulsions and powders: improving antioxidant and flavor properties, Food Funct., 2016, 7, 3694–3702 CAS
.
- X. W. Chen, J. M. Wang, J. Guo, Z. L. Wan, S. W. Yin and X. Q. Yang, Hierarchical high internal phase emulsions and transparent oleogels stabilized by quillaja saponin-coated nanodroplets for color performance, Food Funct., 2017, 8, 823–831 CAS
.
- X. W. Chen, D. X. Yang, Y. Zou and X. Q. Yang, Stabilization and functionalization of aqueous foams by Quillaja saponin-coated nanodroplets, Food Res. Int., 2017, 99, 679–687 CrossRef CAS PubMed
.
-
No, I. S. 8586
Sensory analysis-general guidelines for the selection, training and monitoring of selected assessors and expert sensory assessors, International Organization for Standardization, Geneva, 2012 Search PubMed
.
- K. Wojciechowski, Surface activity of saponin from Quillaja bark at the air/water and oil/water interfaces, Colloids Surf., B, 2013, 108, 95–102 CrossRef CAS PubMed
.
- R. Stanimirova, K. Marinova, S. Tcholakova, N. D. Denkov, S. Stoyanov and E. Pelan, Surface rheology of saponin adsorption layers, Langmuir, 2011, 27, 12486–12498 CrossRef CAS PubMed
.
- S. Böttcher and S. Drusch, Saponins self-assembly and behavior at aqueous interfaces, Adv. Colloid Interface Sci., 2017, 243, 105–113 CrossRef PubMed
.
- A. B. D. Nandiyanto and K. Okuyama, Progress in developing spray-drying methods for the production of controlled morphology particles: From the nanometer to submicrometer size ranges, Adv. Powder Technol., 2011, 22, 1–19 CrossRef CAS
.
- A. Carné-Sánchez, I. Imaz, M. Cano-Sarabia and D. Maspoch, A spray-drying strategy for synthesis of nanoscale metal-organic frameworks and their assembly into hollow superstructures, Nat. Chem., 2013, 5, 203–211 CrossRef PubMed
.
- D. Sen, S. Mazumder, J. S. Melo, A. Khan, S. Bhattyacharya and S. F. D'souza, Evaporation driven self-assembly of a colloidal dispersion during spray drying: volume fraction dependent morphological transition, Langmuir, 2009, 25, 6690–6695 CrossRef CAS PubMed
.
- M. Fatnassi, C. Tourné-Péteilh, T. Cacciaguerra, P. Dieudonné, J. M. Devoisselle and B. Alonso, Tuning nanophase separation and drug delivery kinetics through spray drying and self-assembly, New J. Chem., 2010, 34, 607–610 RSC
.
- F. Caruso, R. A. Caruso and H. Möhwald, Production of hollow microspheres from nanostructured composite Particles, Chem. Mater., 1999, 11, 3309–3314 CrossRef CAS
.
- M. Emorine, C. Septier, I. Andriot, C. Martin, C. Salles and T. Thomas-Danguin, Combined heterogeneous distribution of salt and aroma in food enhances salt perception, Food Funct., 2015, 6, 1449–1459 CAS
.
- Y. Yang, C. Zhao, G. Tian, C. Lu, S. Zhao, Y. Bao and J. Zheng, The effects of preheating and storage temperature on aroma profile and physical properties of citrus-oil emulsions, J. Agric. Food Chem., 2017, 65, 7781–7789 CrossRef CAS PubMed
.
- F. L. Yang, X. G. Li, F. Zhu and C. L. Lei, Structural characterization of nanoparticles loaded with garlic essential oil and their insecticidal activity against Tribolium castaneum, J. Agric. Food Chem., 2009, 57, 10156–10162 CrossRef CAS PubMed
.
- M. Harrison and B. P. Hills, Mathematical model of flavor release from liquids containing aroma-binding macromolecules, J. Agric. Food Chem., 1997, 45, 1883–1890 CrossRef CAS
.
- U. Yucel and D. G. Peterson, Effect of protein-lipid-salt interactions on sodium availability in the mouth and consequent perception of saltiness: In solutions, J. Agric. Food Chem., 2015, 63, 7487–7493 CrossRef CAS PubMed
.
- N. Nasri, C. Septier, N. Beno, C. Salles and T. Thomas-Danguin, Enhancing salty taste through odour-taste-taste interactions: Influence of odour intensity and salty tastants’ nature, Food Qual. Prefer., 2013, 28, 134–140 CrossRef
.
- M. Batenburg and R. van der Velden, Saltiness enhancement by savory aroma compounds, J. Food Sci., 2011, 76, 1750–3841 CrossRef PubMed
.
Footnote |
† Electronic supplementary information (ESI) available. See DOI: 10.1039/c7fo01371j |
|
This journal is © The Royal Society of Chemistry 2018 |