DOI:
10.1039/C7FO01342F
(Paper)
Food Funct., 2018,
9, 643-654
Comparative study of the effects of phosphatidylcholine rich in DHA and EPA on Alzheimer's disease and the possible mechanisms in CHO-APP/PS1 cells and SAMP8 mice
Received
29th August 2017
, Accepted 2nd December 2017
First published on 6th December 2017
Abstract
Metabolic stress induced by a high-fat (HF) diet leads to cognitive dysfunction and aging. In the present study, Chinese hamster ovary cells stably transfected with amyloid precursor protein (APP) and presenilin 1 (PS1) (CHO-APP/PS1 cells) and SAMP8 mice fed with an HF diet were used to study the effects of docosahexaenoic acid (DHA)-enriched phosphatidylcholine (DHA-PC) and eicosapentaenoic acid (EPA)-enriched phosphatidylcholine (EPA-PC) on Alzheimer's disease (AD) and the possible mechanisms involved in these effects. Behavior test results indicated that DHA-PC exerted better effects than EPA-PC on improving memory and cognitive deficiency. Further analysis showed that DHA-PC and EPA-PC could significantly decrease β-amyloid (Aβ) concentrations in CHO-APP/PS1 cells and SAMP8 mice by inhibiting APP, PS1, and BACE1 expression. Moreover, both DHA-PC and EPA-PC can increase the activities of the antioxidant index, including SOD, T-AOC, GSH, and GSH-PX, and inhibit levels of MDA, NO, and NOS. In addition, the expressions of inflammatory factors (TNF-α, IL-1β) and apoptosis were significantly suppressed via improving the ratio of Bcl-2/Bax and decreasing the expression of pro-apoptosis factors. Interestingly, only DHA-PC could improve the expression of neurotrophic factors, including BDNF, synaptophysin, and growth associated protein 43. DHA-PC and EPA-PC could ameliorate memory and cognitive function of HF diet-fed SAMP8 mice via inhibiting Aβ generation, suppressing oxidative stress and apoptosis, down-regulating inflammatory response, and improving neurotrophic activity. Therefore, DHA-PC and EPA-PC may be applied as food supplements and/or functional ingredients to relieve neurodegenerative disease.
1. Introduction
Alzheimer's disease (AD) is a progressive, age-related, neurodegenerative disorder characterized by memory loss and impaired cognitive function. β-Amyloid (Aβ) peptides play a key role in synaptic damage and memory deficits in the early pathogenesis of AD.1 Aβ is produced by the proteolytic processing of amyloid precursor protein (APP). An important way to process APP is the nonamyloidogenic pathway, in which α-secretase cleaves the Aβ domain in APP, thereby precluding the formation of intact Aβ. However, under normal circumstances, a small amount of APP is processed via the amyloidogenic pathway, in which Aβ is released from APP by β- and γ-secretases.2 Various studies have linked Aβ accumulation either directly or indirectly to synaptic loss and neuronal apoptosis, which subsequently cause oxidative stress, neuro-inflammation, mitochondrial dysfunction, and apoptosis.3,4
Environmental and lifestyle factors such as stress, diet and physical activity have been shown to affect cognition and can also increase the risk of late onset AD (LOAD).5 Previous studies suggested that a high fat/high cholesterol diet (HFD) can modify the risk of LOAD.6 Experimental studies employing mouse models of AD revealed that HFD affected amyloid pathology and cognitive function in adult mice. HFD also caused profoundly disordered lipid metabolism and lipid composition in the brain.1,7 The clinical drugs used for the treatment of AD generally involve side effects. For example, administration of bexarotene is usually accompanied by elevation of plasma triglycerides. Therefore, it is necessary to explore functional foods or supplements to prevent neurodegenerative disease.
Fish oil, an important source of n-3 long-chain polyunsaturated fatty acids (n-3 LCPUFA) containing both eicosapentaenoic acid (EPA) and docosahexaenoic acid (DHA), has been verified to benefit brain health.8 Clinical trials have demonstrated that fish oil can reduce AD-associated pathology through alleviating cognitive deficits and protecting against synaptic degeneration.3 The bioactivities of DHA and EPA depend on their chemical forms. DHA has been proven to be essential to pre- and postnatal brain development, whereas EPA appears to be more influential on behavior and mood. Marine foods, such as fish roe and krill oil, are rich in n-3 LCPUFA-phospholipids (n-3 PUFA-PLs), in which DHA and EPA are usually linked at the sn-2 position of the glycerol backbone; it is likely that the majority of marine n-3 LCPUFA-PLs are found in a mixture. Notably, most n-3 LCPUFAs in fish oil exist in the form of triacylglycerols (TG), while more than 40% of n-3 PUFAs in krill oil consist of phospholipids (PL). Krill oil was found to activate cognitive function more effectively than fish oil in the elderly. Previous studies also reported that n-3 LCPUFA-PLs exhibit a more significant influence on cognitive function than n-3 PUFA-TGs.2,9 Phospholipids enriched with DHA (DHA-PL) were also found to improve Aβ-induced cognitive deficiency in AD rat models; the effects of phospholipids enriched with EPA (EPA-PL) were similar to those of DHA-PL.6,10
It has been verified that n-3 LCPUFA-PLs can improve cognitive deficiency in vitro and in vivo. However, most studies have focused on their therapeutic effects; few studies have concentrated on disease prevention involving Aβ generation. Hence, it is necessary to illuminate the different effects of DHA-PC and EPA-PC on Aβ generation. In the present study, CHO-APP/PS1 cells and high fat (HF) diet-fed SAMP8 mice (a spontaneous Alzheimer's disease mouse) were used to compare the effects of DHA-PC and EPA-PC on AD. Subsequently, the underlying mechanisms involving Aβ generation, oxidative stress, neuro-inflammation, apoptosis and neurotrophic effects were also investigated.
2. Materials and methods
2.1 Preparation of DHA-PC and EPA-PC
EPA-PL was extracted from Cucumaria frondosa (Nanshan Aquatic Market, Qingdao, China). DHA-PL was extracted from squid roe (Boow Foods Co., Ltd, Weihai, China). Briefly, lipids were extracted according to a modified method of Folch et al.11 Squid roe and Cucumaria frondosa were ground into powder after vacuum freeze-drying. Then, the powder was extracted with a 20-fold volume of chloroform–methanol solution (2
:
1, v/v) overnight. The extracted solution was mixed with a one-fourth volume of water after filtration. The mixture was placed into a separating funnel and maintained for 24 h; then, the chloroform layer containing the total lipids was collected and evaporated to dryness under vacuum. Then, EPA-PL and DHA-PL were separated from the total lipids by silica-gel column chromatography using chloroform, acetone, chloroform–methanol (9
:
1, v/v), chloroform–methanol (2
:
1, v/v) and methanol sequentially as eluents. The chloroform–methanol (2
:
1, v/v) eluent and methanol eluent were collected; then, EPA-PL and DHA-PL were obtained after removal of the organic solvents under vacuum. EPA-PC and DHA-PC were purified from EPA-PL and DHA-PL respectively by silica-gel column chromatography.
The fatty acid composition of PL was determined using an Agilent 6890 Gas Chromatograph with a flame-ionization detector. The column was a HPINNOW-AX capillary column (30 m × 0.32 mm × 0.25 m). The temperature of the detector and injector were kept constant at 250 °C and 240 °C, respectively, and the oven temperature was increased from 170 °C to 240 °C at 3 °C min−1 and maintained at 240 °C for 15 min. Nitrogen was used as the carrier gas at a flow rate of 1.2 mL min−1. The DHA-PC contained 32.2% DHA and 9.59% EPA, and the EPA-PC contained 52.99% EPA and 0.67% DHA.
2.2 Cell Culture
Chinese hamster ovary cells stably transfected with APP751 and PS1 (M146L) (CHO-APP/PS1 cells) were a gift from the College of Pharmacy (Ocean University of China). The cells were grown in DMEM supplemented with 10% FBS (Gibco, Grand Island, NY, USA), 200 mg mL−1 G418 (Solarbio Life Science, Beijing), and 25 mg mL−1 puromycin (Solarbio Life Science, Beijing) in a humidified atmosphere of 95% air and 5% CO2 at 37 °C. CHO-APP/PS1 cells after 3 to 10 passages were used for further experiments. Before treatment, the cells were seeded at an appropriate density on cell culture plates. Once adhered, appropriate concentrations of DHA-PC and EPA-PC were added to the plates, which were incubated for 48 h.
2.3 Measurement of cell viability
The cell viability was determined using the MTT assay. CHO-APP/PS1 cells were plated at a density of 100
000 cells per mL in 96-well plates. Following phospholipid treatment, the medium was removed, and the cells were washed with PBS; then, MTT (St Louis, MO, USA) dissolved in DMEM was added to each well for an additional 4 h at 37 °C in a CO2 incubator. The formazan was dissolved in 200 μL acidated dimethylcarbinol after removing the medium. The absorbance at 570 nm was measured using a microplate reader (Model 680, Bio-Rad, Tokyo, Japan). Cell viability was expressed as a percentage of the control value.
2.4 Animals
Male SAMP8 mice, 6 months old, were purchased from Nanjing Qingzilan Technology Co., Ltd. All the mice were housed under a 12 h/12 h light/dark cycle at 22 °C with 60 ± 10% humidity. At 10 months of age, the SAMP8 mice were randomly divided into four groups (8 animals per group): low fat (LF) diet group, HF diet group, DHA-PC group and EPA-PC group. The mice were fed with either a LF diet (OpenSource diets #D12450), or a HF diet (OpenSource diets #D12492). The DHA-PC and EPA-PC groups were fed with the HF diet containing 1% DHA-PC and 1% EPA-PC, respectively, for 2 months. The compositions of the experimental diets are shown in Table 1. The animal study protocol was approved by the Animal Ethics Committee of the College of Food Science and Engineering of the Ocean University of China. All the animals were housed at the Laboratory Animal Facility at the Ocean University of China. The research was conducted in accordance with the Guide for the Care and Use of Laboratory Animals (8th edition, Institute of Laboratory Animal Resources on Life Sciences, National Research Council, National Academy of Sciences, Washington DC).
Table 1 Compositions of experimental diets (g kg−1)
Ingredient |
LF group |
HF group |
DHA-PC group |
EPA-PC group |
n.d. = not detected. |
Casein |
140 |
140 |
140 |
140 |
Potato starch |
617.7 |
430.7 |
430.7 |
430.7 |
Sucrose |
100 |
100 |
100 |
100 |
Corn oil |
23.9 |
46 |
46 |
46 |
Lard |
19.1 |
184 |
174 |
174 |
Mineral mix |
35 |
35 |
35 |
35 |
Vitamin mix |
10 |
10 |
10 |
10 |
Cellulose |
50 |
50 |
50 |
50 |
L-Cysteine |
2.5 |
2.5 |
2.5 |
2.5 |
Choline bitartrate |
1.8 |
1.8 |
1.8 |
1.8 |
DHA-PC |
n.d. |
n.d. |
10 |
n.d. |
EPA-PC |
n.d. |
n.d. |
n.d. |
10 |
2.5 Behavior test
Briefly, a circular stainless steel pool (130 cm in diameter and 50 cm in height) was divided into four quadrants which were marked with a triangle, square, diamond, and circle, respectively. The pool was filled with ink-stained water to distinguish the mice from the background. The water temperature was controlled at 21 °C to 23 °C. A circular black escape platform (9 cm in diameter and 29 cm high) was located 1.5 cm beneath the surface of the water in the center of one quadrant. The mice were trained to find the platform with three trials on the first day; then, their ability to find the hidden platform was tested for six consecutive days. The time to find the platform was recorded as latency. If the animal was unable to reach the hidden platform within 60 s, the latency was recorded as 60 s. Regardless of whether the platform was found, the mouse was placed on it for 15 s. The swim paths, distances, and latencies taken to swim to the platform were monitored with a video camera linked to a computer system. Probe tests were performed on the seventh day to evaluate spatial memory retention after the platform was removed. The mice were placed in a position opposite the location of the platform and allowed to swim for 60 s. The number of times the mice crossed over the previous position of the platform and the time spent in the target quadrant were recorded as measures of spatial memory.
2.6 Preparation of hippocampus and brains
All the mice were decapitated after completing the behavioral studies. The brains were separated and divided into cortex, hippocampi and white matter on ice. All the tissues were frozen with liquid nitrogen and stored at −80 °C until use.
2.7 Determination of Aβ concentration by ELISA
CHO-APP/PS1 cells were plated in six-well plates at a density of 200
000 cells per well. Following phospholipid treatment, the medium was recovered for quantitative determination of the Aβ42 concentration. The cells were lysed through ultrasonic treatment for 1 minute and centrifuged at 4500g for 10 min at 4 °C. The supernatant was collected for quantitative determination of the intracellular Aβ42 level. The protein concentrations were determined using a BCA protein assay kit (Nanjing Jiancheng Bioengineering Institute, Jiangsu, China). The intracellular and extracellular Aβ42 levels of CHO-APP/PS1 cells were measured using ELISA kits.
The right hippocampus was homogenized in 10 volumes of TBS (pH 8.0) containing protease inhibitors (20 g ml−1 each pepstatin A, aprotinin, phosphoramidon, and leupeptin, 0.5 mM PMSF, and 1 mM EGTA). Samples were sonicated briefly (two times for 10 s) and centrifuged at 100
000g for 20 min at 4 °C. The soluble fraction (supernatant) was stored for quantitative determination of the soluble Aβ40 and Aβ42 concentrations, whereas the TBS-insoluble pellet was first sonicated in 10 volumes of 2% SDS. The SDS-soluble fraction was used for BACE1 detection. To analyze the insoluble Aβ level, the SDS-insoluble pellet was dissolved and sonicated in 70% formic acid. The extract was neutralized with 0.5 M tris containing 30% acetonitrile and 5 M NaOH before loading on the ELISA plate. The soluble/insoluble Aβ40 and Aβ42 contents in the hippocampus were measured by ELISA kits. The concentration of BACE1 in the hippocampus was measured using ELISA kits (Wuhan USCN Business Co., Ltd, Wuhan, China) according to the manufacturer's instructions.
2.8 Measurement of oxidative stress parameters
The white matter was prepared as a 10% (w/v) tissue homogenate in 0.9% saline solution. Then, the homogenate was centrifuged and the supernatant was collected. The protein concentrations were determined using a BCA protein assay kit. The concentrations of nitric oxide (NO) and malonaldehyde (MDA) and the activities of superoxide dismutase (SOD), inducible nitric oxide synthase (NOS), glutathione peroxidase (GSH-PX), glutathione (GSH) and total antioxidant capacity (T-AOC) were detected with corresponding assay kits (Nanjing Jiancheng Bioengineering Institute, Jiangsu, China).
2.9 Protein and RNA extraction
CHO-APP/PS1 cells were homogenized in RIPA lysis buffer containing protease inhibitor to extract the protein. The total protein and RNA of the left hippocampus were extracted using a total-DNA-RNA-Protein Kit, following the appropriate operating instructions (Omega Bio-Tek, Inc. San Francisco CA, USA).
2.10 Real-time PCR assay
The concentration of total RNA was assessed using a NanoDrop 2000 spectrophotometer (Thermo Scientific, USA). The total RNA (2 μg) from each sample was reverse transcribed to cDNA using random primers and Moloney murine leukemia virus (MMLV) reverse transcriptase (Madison, WI). Selected genes were amplified using SYBR Green I Master Mix (Roche Applied Science, Mannheim, Germany) in an iQ5 real-time detection system (Bio-Rad Laboratories, Hercules, CA, USA) with 0.3 μM of both the forward and reverse primers. The PCR conditions were as follows: 1 cycle of 95 °C for 10 min, 45 cycles of 95 °C for 15 s, 55 °C to 60 °C for 20 s and 72 °C for 30 s. The purities of the PCR products were assessed by melt curve analysis. The primers were synthesized by Shanghai Sangon Gene Company (Shanghai. China), and the sequences are shown in Table 2. Relative gene expression was quantified using the standard curve method. Results were expressed as the relative values after normalization to β-actin RNA.
Table 2 Sequences of the primers used in quantitative RT-PCR
Gene |
Forward primer |
Reverse primer |
APP |
GCCGTGGCATTCTTTTGGGGC |
GTGGTCAGTCCTCGGTCGGC |
PS1 |
CATCATGATCAGTGTCATTGTTGT |
TGCATTATACTTGGAATTTTTGGA |
BACE1 |
GGCGGGAGTGGTATTATGAAGTGA |
GAAGGATGGTGATGCGGAAGG |
GFAP |
GAAACCAACCTGAGGCTGG |
GGATCTCCTCCTCCAGCGA |
IL-1β |
CTTCAGGCAGGCAGTATCACTCAT |
TCTAATGGGAACGTCACACACCAG |
Bax |
TGCAGAGGATGATTGCTGAC |
GATCAGCTCGGGCACTTTAG |
Bcl-2 |
CGGGAGAACAGGGTATGATA |
CCACCGAACTCAAAGAAGG |
BDNF |
CGGGACGGTCACAGTCCTA |
GGGATTACACTTGGTCTCGTAGAAATAC |
Synaptophysin |
CATTCAGGCTGCACCAAGTG |
TGGTAGTGCCCCCTTTAACG |
β-Actin |
GCAGATGTGGATCAGCAAGC |
GTCAAAGAAAGGGTGTAAAACG |
2.11 Western blot analysis
Protein concentration was determined by the BCA method. Equal amounts of protein were separated on 5% to 12% SDS-PAGE gels and transferred to poly (vinylidene fluoride) membranes. The membranes were incubated with antibodies against APP, PS1, BACE1, Bax, Bcl-2, Caspase 3, Caspase 9, TNF-α, IL-1β, synaptophysin (SYN), growth associated protein-43 (GAP-43) (purchased from Abcam Inc.), and brain derived neurotrophic factor (BDNF) (purchased from Cell Signal Technology Co.) overnight at 4 °C. After this, the membranes were incubated with goat anti-rabbit IgG for 2 h at room temperature, and the blots were detected with chemiluminescent horseradish peroxidase substrate.
2.12 Statistical analysis
The data have normal distributions and are expressed as mean ± standard deviation (SD). One-way analysis of variance (ANOVA) followed by Duncan's test were performed using SPSS version 10.0 software (SPSS Institute, Inc., Chicago, IL, USA). P < 0.01 and P < 0.05 were considered statistically significant. The graphs were generated using Prism 5 software (Graph-Pad Software, Inc., San Diego, CA, USA). Different letters indicate significant differences between each group.
3 Results
3.1 Effects of Phospholipids on cell viability and Aβ generation in CHO-APP/PS1 Cells
The cell viabilities of DHA-PC and EPA-PC on CHO-APP/PS1 cells were determined by MTT assay, and the results are shown as relative cell viability in reference to the control (equal to 100%). As shown in Fig. 1A, DHA-PC and EPA-PC with concentrations of 0 to 60 μg mL−1 had no detectable toxic effects on the growth of CHO-APP/PS1 cells within 48 h; therefore, concentrations of 20 and 40 μg mL−1 were selected for further experiments.
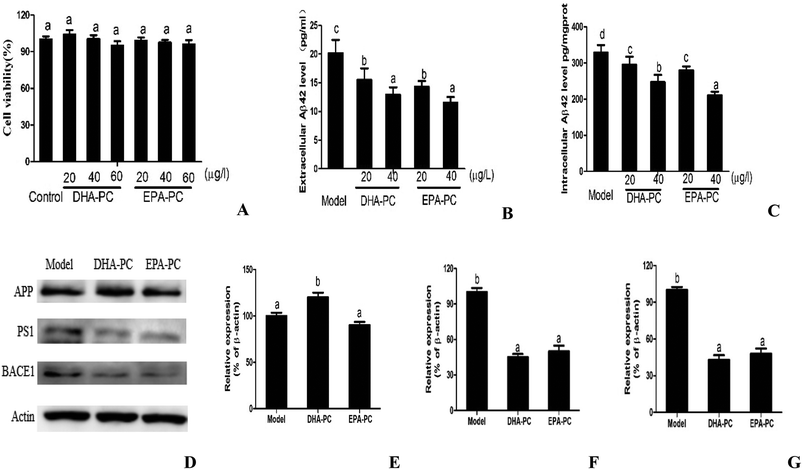 |
| Fig. 1 (A) Effects of DHA-PC and EPA-PC on cell viability in CHO-APP/PS1 cells. Levels of (B) extracellular Aβ42 and (C) intracellular Aβ42 were measured by ELISA. Representative western blots (D) and densitometry of APP (E), PS1 (F) and BACE1 (G). Data are presented as mean ± SD (n = 8); different letters indicate significant differences between the groups. | |
The effects of PL on extracellular and intracellular Aβ generation are shown in Fig. 1B and C. Both DHA-PC and EPA-PC could significantly decrease the extracellular and intracellular Aβ42 levels in a dose-dependent manner. There was no statistical difference between EPA-PC and DHA-PC in decreasing extracellular Aβ42 concentrations with the same concentration. Interestingly, EPA-PC at the concentration of 40 μg mL−1 exhibited a lower intracellular Aβ42 level than DHA-PC at the same concentration, whereas no statistical difference was observed between EPA-PC and DHA-PC at the concentration of 20 μg mL−1.
The western blot technique was used to study the possible mechanisms involved in these effects, and the results are shown in Fig. 1D–F. There was no significant difference in APP protein level between the model group and the EPA-PC group, and the APP protein level of the DHA-PC group significantly increased compared with that of the model group. Notably, pretreatment of DHA-PC and EPA-PC markedly decreased PS1 and BACE1 expression, and no statistical difference was observed between the two groups.
3.2 Effects of DHA-PC and EPA-PC on cognitive deficiency in behavioral tests
Spatial learning and memory tests were performed on the mice using the Morris water maze, and the results are shown in Fig. 2. In the training session, the SAMP8 mice fed with a LF diet rapidly learned the location of the platform, whereas the HF group had longer escape latency than the LF group. Supplementation of DHA-PC and EPA-PC improved the learning performance of SAMP8 mice in the acquisition phase of training (day 1 to 3 of training) and in the consolidation phase (day 4 to 5 of training) (Fig. 2A). Interestingly, DHA-PC provided a more significant therapeutic benefit than EPA-PC in alleviating spatial deficits of SAMP8 mice.
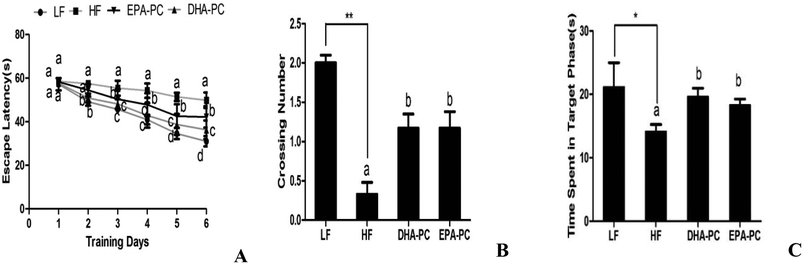 |
| Fig. 2 Effects of DHA-PC and EPA-PC on spatial learning and memory deficiency: (A) time needed to reach the hidden platform in the Morris maze. The number of platform crossings (B) and the time spent in the target quadrant (C) were measured for analysis of spatial memory function. Data are presented as mean ± SD (n = 8); *P < 0.05, **P < 0.01 were considered statistically significant. Different letters indicate significant differences between the groups. | |
A probe trial was carried out to evaluate the memory of SAMP8 mice after completing the 6-day training. The results showed that the HF diet could significantly decrease the number of platform crossings and the time spent in the target quadrant compared to the LH diet (Fig. 2B and C). Interestingly, DHA-PC and EPA-PC could notably improve the time spent in the target quadrant and the number of platform crossings; there was no statistical difference between these two groups.
3.3 Effects of DHA-PC and EPA-PC on Aβ generation
The effects of DHA/EPA-PC on the concentration of soluble/insoluble Aβ40 and Aβ42 in the hippocampus are shown in Fig. 3. The HF diet significantly increased soluble and insoluble Aβ40 and Aβ42 levels compared with the LF diet. Supplementation of DHA-PC and EPA-PC produced a significant reduction of soluble and insoluble Aβ40 and Aβ42 levels; there was no significant difference between DHA-PC and EPA-PC in inhibiting insoluble Aβ40 generation. Notably, DHA-PC was superior to EPA-PC in decreasing soluble Aβ40 and soluble/insoluble Aβ42 levels.
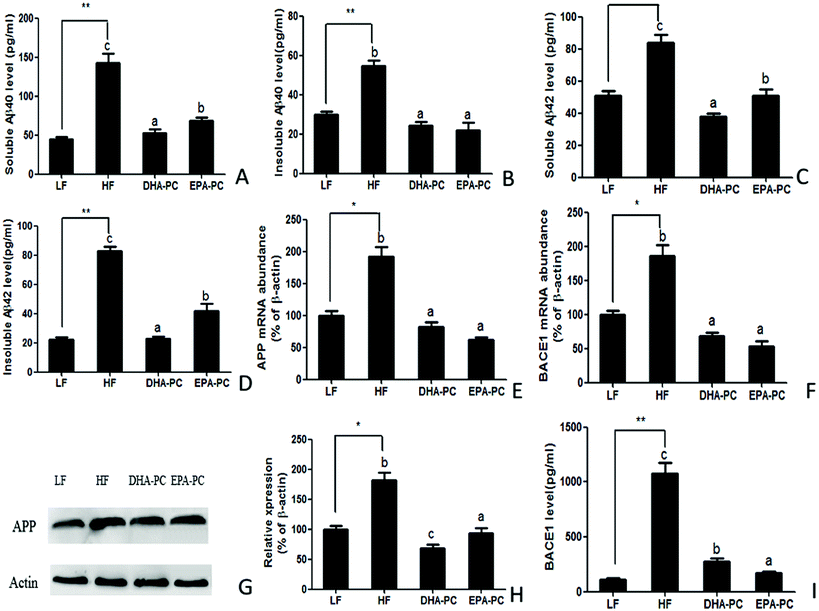 |
| Fig. 3 Effects of DHA-PC and EPA-PC on the regulation of Aβ accumulation. Levels of (A) soluble Aβ40, (B) insoluble Aβ40, (C) soluble Aβ42 and (D) insoluble Aβ42 were measured by ELISA. Relative mRNA levels of APP (E) and BACE1 (F). Representative western blots (G) and densitometry of APP (H). The level of BACE1 was assessed by ELISA (I). Data are presented as mean ± SD (n = 8); *P < 0.05, **P < 0.01 were considered statistically significant. Different letters indicate significant differences between the groups. | |
Aβ is a cleaved fragment of APP via BACE1 and γ-secretase. Both the mRNA level and protein expression of APP and BACE1 were measured, and the results are shown in Fig. 3E–I. Compared with the LF group, the HF group had significantly increased mRNA levels and protein expression of APP and BACE1. Supplementation of DHA-PC and EPA-PC obviously decreased the mRNA and protein levels of APP and BACE1. Moreover, western blot analysis showed that DHA-PC was superior to EPA-PC in decreasing the protein expression of APP. Interestingly, EPA-PC exerted a more significant effect than DHA-PC in decreasing BACE1 level.
3.4 Effects of DHA-PC and EPA-PC on oxidative stress in the brain
Parameters of oxidative stress, such as SOD, MDA, NOS, NO, GSH, GSH-PX and T-AOC, were detected; the results are shown in Fig. 4. Compared with the LF group, the HF group had significantly decreased SOD, GSH, GSH-PX and T-AOC activities, increased levels of MDA and NO, and increased NOS activity. Importantly, DHA-PC and EPA-PC treatment significantly recovered the activities of SOD, GSH, GSH-PX and T-AOC. DHA-PC and EPA-PC treatment also obviously decreased the MDA concentration, NOS activity and NO level. No statistical difference was observed between these two groups in regulating the parameters of oxidative stress.
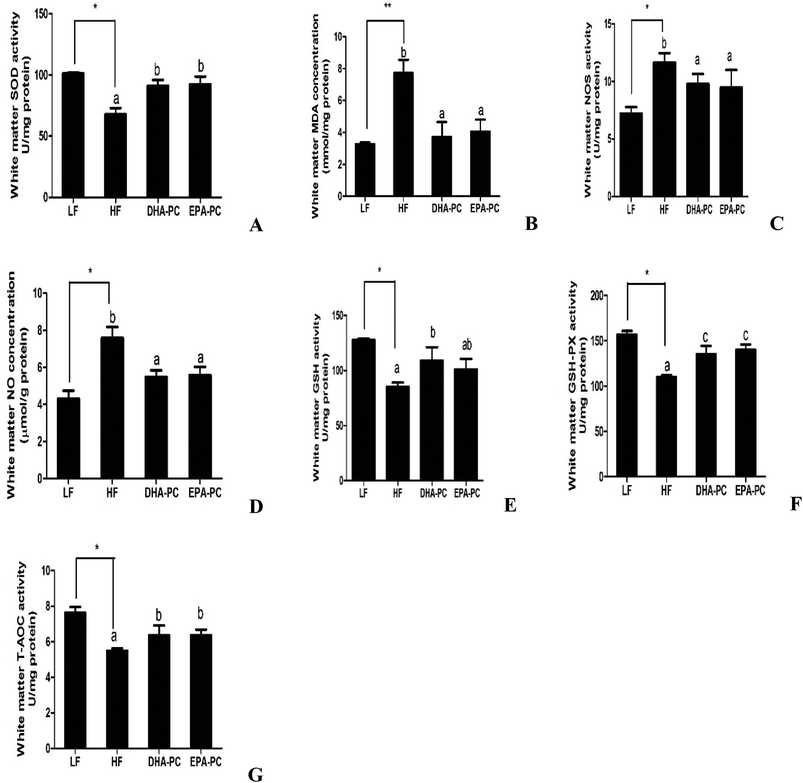 |
| Fig. 4 Effects of DHA-PC and EPA-PC on SOD activity (A), MDA concentration (B), NOS activity (C), NO concentration (D), GHS activity (E), GSH-PX (F), T-AOC activity (G) in mouse white matter. Data are expressed as mean ± SD (n = 8); *P < 0.05, **P < 0.01 were considered statistically significant. Different letters indicate significant differences between the groups. | |
3.5 Effects of DHA-PC and EPA-PC on inflammatory cytokines
RT-PCR analysis demonstrated that the mRNA levels of the inflammatory markers GFAP and IL-1β were significantly elevated in the HF group compared to the LF group. Comparatively, the administration of DHA-PC and EPA-PC resulted in a clear reduction in the mRNA levels of these inflammatory markers (Fig. 5A and B). Further supporting this data, western blot analysis demonstrated that the expression levels of TNF-α and IL-1β in the HF group were obviously increased (Fig. 5C–E) compared with those of the LF group. Supplementation of DHA-PC and EPA-PC obviously decreased the expressions of TNF-α and IL-1β. Interestingly, DHA-PC exerted a more significant effect than EPA-PC in decreasing the IL-1β level; there was no statistical difference between the two groups in inhibiting the protein expression of TNF-α.
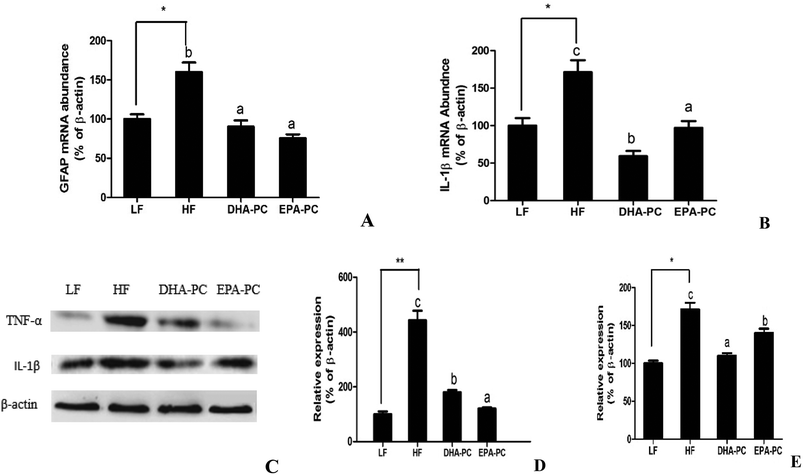 |
| Fig. 5 Effects of DHA-PC and EPA-PC on inflammatory activity. (A) Relative mRNA levels of GFAP and IL-1β (B), western blot analysis of TNF-α and IL-1β (C), protein expression of IL-1β (D) and TNF-α (E). Values are indicated as mean ± SD (n = 8); *P < 0.05, **P < 0.01 were considered statistically significant. Different letters indicate significant differences between the groups. | |
3.6 Effects of DHA-PC and EPA-PC on apoptosis in mouse brain hippocampus
As shown in Fig. 6, the mRNA level of Bax was increased in the HF group and markedly decreased in the DHA-PC and EPA-PC groups, whereas no statistical difference was observed between the DHA-PC and EPA-PC groups. DHA-PC and EPA-PC obviously up-regulated both the mRNA level and protein level of Bcl-2, and DHA-PC exerted a more significant effect than EPA-PC on increasing the Bcl-2 level. Western blot analysis demonstrated the protein expression of crucial apoptosis factors, including Bcl-2, Bax, Caspase 3 and Caspase 9 (Fig. 6). The relative density of Bcl-2/Bax in the HF group significantly decreased compared to that of the LF group (Fig. 6D). Dietary supplementation of DHA-PC and EPA-PC significantly increased the Bcl-2/Bax ratio, and DHA-PC was superior to EPA-PC. The results for Caspase 3 and Caspase 9 showed that the HF diet apparently increased the expression levels of these two pro-apoptosis factors compared to the LF diet. DHA-PC and EPA-PC depressed the protein levels of Caspase 3 and Caspase 9; no statistical difference was observed for the decrease in expression of Caspase 3. Interestingly, EPA-PC exhibited a more significant improvement than DHA-PC in suppressing the expression of Caspase 9.
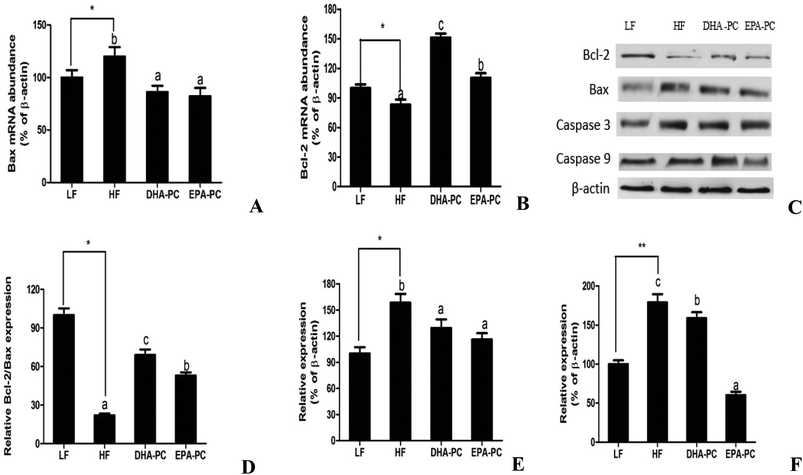 |
| Fig. 6 Effects of DHA-PC and EPA-PC on apoptotic genes and protein. (A) Bax mRNA level, (B) Bcl-2 mRNA level, and expression of Bcl-2, Bax, Caspase 3 and Caspase 9 were measured by western blot (C). Relative protein expression of Bcl-2/Bax (D), Caspase 3 (E), Caspase 9 (F). *P < 0.05, **P < 0.01 were considered statistically significant. Different letters indicate significant differences between the groups. | |
3.7 Effects of DHA-PC and EPA-PC on neurotrophic factors
Neurotrophic factors are related to synaptic plasticity; thus, BDNF, SYN and GAP-43 were investigated to illustrate the benefits of DHA-PC and EPA-PC on synaptic transmission. According to the results shown in Fig. 7, the HF diet significantly decreased the mRNA levels of BDNF and SYN. There were no statistical differences in BDNF and SYN levels between the EPA-PC and HF groups. Interestingly, DHA-PC could significantly improve the BDNF and synapse levels, suggesting that DHA-PC was superior to EPA-PC in improving the BDNF and SYN levels. Moreover, western blot analysis was used to further support the above results. The results showed that dietary supplementation of DHA-PC obviously increased the protein expressions of BDNF, SYN and GAP-43 compared with those of the HF group. However, no statistical differences were observed between the HF group and the LF group in BDNF, SYN and GAP-43 expression. Unfortunately, EPA-PC significantly decreased these three neurotrophic factors.
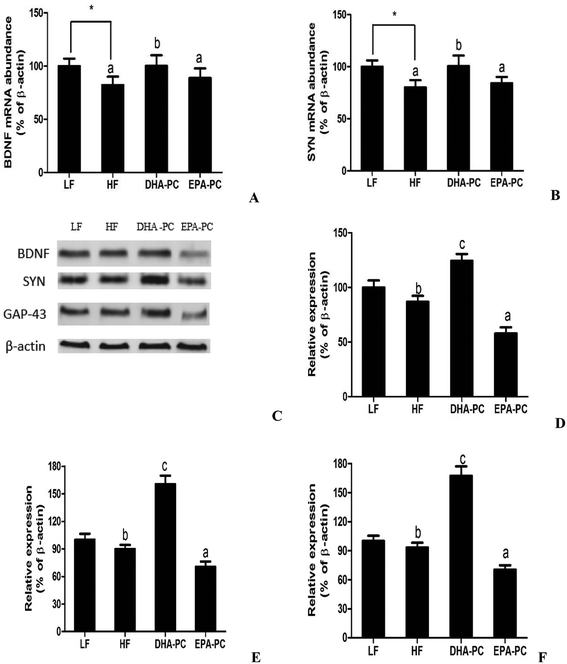 |
| Fig. 7 Effects of DHA-PC and EPA-PC on neurotrophic factors. Representative mRNA levels of BDNF (A) and SYN (B). The expression of BDNF, SYN and GAP-43 were measured by western blot (C). Relative protein expression of BDNF (D), SYN (E), GAP-43 (F). Data are presented as mean ± SD (n = 8); *P < 0.05 was considered statistically significant. Different letters indicate significant differences between the groups. | |
4 Discussion
PLs have been reported to provide beneficial effects against neurodegenerative disorders, including dementia, AD, Parkinson's disease and age-related cognitive decline.12 Marine foods, such as fish roe and krill, are rich in n-3 LCPUFA-PLs, in which DHA and EPA are usually linked at the sn-2 position of the glycerol backbone. However, the beneficial effects of n-3 LCPUFA on neural function have been largely linked to the actions of DHA, an elongated/desaturated product of EPA. This is likely due to brain lipid content, wherein DHA, not EPA, constitutes the major PUFA brain lipid. Accordingly, DHA constitutes 35% to 40% of total polyunsaturated fatty acids, and this fatty acid is mainly located among the phospholipids forming the neuronal membranes and the retina, whereas EPA, a precursor of DHA, constitutes a relatively smaller percentage.13,14 A previous study verified that DHA-PL can improve Aβ-induced cognitive deficiency in a rat model of AD.15 In the present study, we found that both DHA-PC and EPA-PC could inhibit Aβ generation and ameliorate memory and cognitive function.
Aβ deposition is a key factor implicated in neuronal damage and cognitive dysfunction in AD.16 Aβ is formed by the sequential proteolysis of APP. β-Secretase enzyme, widely known as β-site amyloid precursor protein cleaving enzyme 1 (BACE1), firstly cleaves APP to yield a membrane-bound C-terminal fragment called C99. A second enzyme named γ secretase then cuts C99 to liberate Aβ. γ-Secretase is a membrane-embedded, multicomponent proteolytic enzyme consisting of four transmembrane proteins, namely presenilin 1 (PS1), nicastrin, Pen2, and Aph1.2,17 CHO-APP/PS1 cells are widely used as an AD model in vitro.18 The results showed that DHA-PC and EPA-PC could significantly decrease the intracellular and extracellular Aβ42 concentrations through inhibiting the functions of β-secretase and γ-secretase.
To further study the effects of DHA-PC and EPA-PC on AD and investigate the underlying mechanisms, SAMP8 mice were used to investigate the protective effects of DHA-PC and EPA-PC. SAMP8 mice are a model of spontaneously accelerated aging; they exhibit neuropathological abnormalities and cognitive and behavioral alterations observed in AD patients, such as deficits in learning and memory, oxidative stress, and Aβ deposition.19 The Morris water maze results indicated that the HF diet aggravated the cognitive deficiency of SAMP8 mice. DHA-PC and EPA-PC treatments showed a shorter escape latency, and DHA-PC exerted a more significant function, which indicated that DHA-PC was superior to EPA-PC in ameliorating learning deficits. Moreover, DHA-PC and EPA-PC treatment could increase the number of platform crossings and the time spent in the target phase, suggesting that DHA-PC and EPA-PC can improve memory deficits. Our previous studies proved that dietary supplementation of EPA-PLs for 12 weeks prevented the impairment of learning and memory in SAMP8 mice, and DHA-PC improved learning and memory in Aβ-induced cognitive deficiency in an AD rat model, which is consistent with our results.10,17,20
APP is cleaved by BACE1 and γ-secretases to produce Aβ40 and Aβ42. Aβ42 is more hydrophobic than Aβ40 and presents a higher potential for aggregation.21 Aβ is produced as a monomer; however, it readily aggregates to form multimeric complexes. These complexes range from low molecular weight dimers and trimers to high molecular weight protofibrils, which are soluble Aβ. Fibrillary Aβ, known as amyloid plaques, is insoluble Aβ.22 Therefore, we measured the soluble and insoluble Aβ40 and Aβ42 levels in the hippocampus. The results showed that DHA-PC and EPA-PC clearly inhibited the generation of Aβ, and DHA-PC exerted more significant effects than EPA-PC in decreasing soluble Aβ40, soluble Aβ42, and insoluble Aβ42. Next, we explored the possible mechanism associated with Aβ generation. The results showed that DHA-PC and EPA-PC obviously decreased both the mRNA and protein levels of APP and BACE1. Notably, DHA-PC was superior to EPA-PC in decreasing Aβ generation.
Recent studies have demonstrated that dietary DHA-PC and EPA-PL can alleviate brain lipid peroxidation in Aβ-induced cognitive deficiency of aged rats.7,10 Moreover, EPA-PL can protect PC12 cells from oxidative stress.17 The present study suggests that DHA-PC and EPA-PC can reverse the decrease of SOD, T-AOC, GSH, GSH-PX activities and reduce MDA production in white brain matter. These results indicate that DHA-PC and EPA-PC can improve cognitive impairment and inhibit the production of Aβ in the brain of SAMP8 mice by preventing oxidative stress. Oxidative stress has been shown to affect Aβ generation.18,23 Meanwhile, other researchers have suggested that Aβ affects oxidative stress through neuronal lipid peroxidation, protein oxidation, and DNA oxidation. Soluble, aggregated Aβ is postulated to insert into neuronal membranes and induce the formation of ROS and increased neurotoxicity.24 A previous report suggested that n-3 LCPUFAs are an agonist of PPARα, which plays a crucial role in maintaining and restoring redox balance altered by oxidative stress mechanisms. We speculate that DHA-PC and EPA-PC can prevent oxidative stress via activating PPARα.25
Aβ production and deposition can trigger microglia and astrocyte activation, leading to the production of pro-inflammatory cytokines and neurotoxic molecules such as TNF-α, nitric oxide, IL-1β, and ROS, which produce toxic effects on neural tissue.22,26 Moreover, inflammatory cytokines can trigger the production of β-amyloid peptides.27 Previous studies have demonstrated that DHA-PC and EPA-PL can reduce the production of inflammatory factors.6,10 We investigated the mRNA level of GFAP, a predominant marker of glial activation. A detectable increase in GFAP mRNA level was observed, indicating the activation of astrocytes in the brain of SAMP8 mice fed with a high fat diet. We then examined the levels of pro-inflammatory factors, including IL-1β and TNF-α, which can stimulate microglia and astrocyte activation. The results showed that the HF diet significantly elevated the IL-1β and TNF-α contents. DHA-PC and EPA-PC effectively reduced the IL-1β and TNF-α levels, which suppressed neuro-inflammation. These results suggest that DHA-PC and EPA-PC modulate the neuro-inflammation cascade. Importantly, we discovered that DHA-PC has a more significant impact on decreasing the IL-1β level than EPA-PC in the present results. Serhan et al. reported that lipid mediators derived from DHA and EPA displayed potent anti-inflammatory and immunoregulatory actions in vitro and in vivo in murine models of inflammatory diseases.28 We believe that the anti-inflammatory functions of DHA-PC and EPA-PC may partly depend on lipid mediators derived from DHA and EPA.
Mitochondria-dependent apoptosis is known to be involved in many pathological conditions, including Aβ-induced neurotoxicity. Bax, a member of the Bcl-2 family, can initiate apoptosis. Bcl-2 is identified as a negative regulator of apoptosis. The ratio of Bcl-2/Bax is the key indicator of inhibition of mitochondria-dependent apoptosis.29 Caspase-9 and Caspase-3 are the initiator and executioner, respectively. Our findings indicated that DHA-PC and EPA-PC can significantly increase the ratio of Bcl-2/Bax and inhibit the expression of Caspase 3 and Caspase 9. DHA-PC has a more significant impact than EPA-PC on improving the ratio of Bcl-2/Bax. Interestingly, EPA-PC was superior to DHA-PC in decreasing the expression of Caspase 9. We speculate that other pathways may be involved in regulating Caspase 9. A previous study introduced acidic noncaspase protease-like cathepsins as novel mediators of apoptosis.30 Cathepsins may be involved in regulating the expression of Caspase 9.
Many reports have verified that neurotrophins are involved in neuronal survival, neurogenesis, differentiation and synaptic plasticity.31 Brain-derived neurotrophic factor (BDNF) is a prototypic neurotrophin that regulates diverse developmental events from the selection of neural progenitors to terminal dendritic differentiation and connectivity of neurons.32 SYN, the protein located at the presynaptic vesicle, is known to have a vital effect on cognitive behavior.33 GAP-43 regulates the growth state of axon terminals, and deficient expression of GAP-43 results in weakened neuron regeneration ability after damage.34 BDNF treatment has been proved to stimulate protein expression of SYN and GAP-43. Increased BDNF expression can alleviate damaged synaptogenesis of neurons in neuronal diseases.35 DHA is the most abundant n-3 LCPUFA in the central and peripheral nervous system; it plays an important role in neurogenesis and synaptogenesis. The beneficial effects of n-3 LCPUFAs on neural function have been largely linked to the action of DHA, an elongated/desaturated product of EPA.8 Our results showed that DHA-PC significantly increased the protein levels of BDNF, SYN and GAP-43, which demonstrates the neuroprotective effects of DHA-PC in synaptic plasticity. Also, DHA-PC may increase the expressions of SYN and GAP-43 by stimulating BDNF expression.
In summary, our study demonstrated that both DHA-PC and EPA-PC may have beneficial effects on AD. The behavior test results indicated that DHA-PC exerts better effects than EPA-PC on escape latency. The mechanism study showed that DHA-PC exhibited more significant effects than EPA-PC on inhibiting the generation of soluble Aβ40/Aβ42 and the IL-1β level, while promoting the expressions of BDNF, SYN, GAP-43 and the protein ratio of Bcl-2/Bax. Meanwhile, EPA-PC was superior to DHA-PC in decreasing pro-apoptotic factor Caspase 9 and the inflammatory cytokine TNF-α. Therefore, DHA-PC and EPA-PC may be applied as food supplements and/or functional ingredients to relieve neurodegenerative disease.
Conflicts of interest
The authors declare that there are no conflicts of interest.
Acknowledgements
This work was supported by grants from the National Natural Science Foundation of China (No. 31371757), the State Key Program of National Natural Science of China (No. 31330060) and the National Natural Science Foundation of China-Shandong Joint Fund for Marine Science Research Centers (U1606403).
References
- A. A. Farooqui, T. Farooqui and L. A. Horrocks, Metabolism and Functions of Bioactive Ether Lipids in the Brain, Indian J. Hortic., 2008, 4698, 729–740 Search PubMed.
- N. J. Munn, E. Arnio, D. Liu, R. A. Zoeller and L. Liscum, Deficiency in ethanolamine plasmalogen leads to altered cholesterol transport, J. Lipid Res., 2003, 44, 182–192 CrossRef CAS.
- E. Kraffe, P. Soudant and Y. Marty, Fatty acids of serine, ethanolamine, and choline plasmalogens in some marine bivalves, Lipids, 2004, 39, 59–66 CrossRef CAS PubMed.
- A. Naud, R. Cabr, M. Jov, V. Ayala, H. Gonzalo, M. Portero-Otn, I. Ferrer and R. Pamplona, Lipidomics of human brain aging and Alzheimer's disease pathology, Int. Rev. Neurobiol., 2015, 122, 133–189 CrossRef PubMed.
- A. A. Farooqui, Lipid mediators in the neural cell nucleus: their metabolism, signaling, and association with neurological disorders, Neuroscientist, 2009, 15, 392–407 CrossRef CAS PubMed.
- A. M. Luoma, F. Kuo, O. Cakici, M. N. Crowther, A. R. Denninger, R. L. Avila, P. Brites and D. A. Kirschner, Plasmalogen phospholipids protect internodal myelin from oxidative damage, Free Radicals Biol. Med., 2015, 84, 296–310 CrossRef CAS PubMed.
- M. Ifuku, T. Katafuchi, S. Mawatari, M. Noda, K. Miake, M. Sugiyama and T. Fujino, Anti-inflammatory/anti-amyloidogenic effects of plasmalogens in lipopolysaccharide-induced neuroinflammation in adult mice, J. Neuroinflammation, 2012, 9, 1–13 Search PubMed.
- F. Echeverra, R. Valenzuela, H. R. Catalina and A. Valenzuela, Docosahexaenoic acid (DHA), a fundamental fatty acid for the brain: New dietary sources, Prostag. Leukotr. Ess., 2017, 124, 1–10 CrossRef PubMed.
- S. Hiratsuka, K. Koizumi, T. Ooba and H. Yokogoshi, Effects of dietary docosahexaenoic acid connecting phospholipids on the learning ability and fatty acid composition of the brain, J. Nutr. Sci. Vitaminol., 2009, 55, 374–380 CrossRef CAS PubMed.
-
P. L. Wood, A. Khan, R. Mankidy, T. Smith and D. B. Goodenowe, Plasmalogen deficit: a new and testable hypothesis for the etiology of Alzheimer's disease, 2011 Search PubMed.
- J. Folch, M. Lees and G. H. Stanley, A Sample Method for the Isolation and Purification of Total Lipids from Animal Tissue, J. Biol. Chem., 1957, 226, 497–509 CAS.
- D. Wang, L. Zhang, M. Wen, L. Du, X. Gao, C. Xue, J. Xu and Y. Wang, Enhanced neuroprotective effect of DHA and EPA-enriched phospholipids against 1-methyl-4-phenyl-1,2,3,6-tetrahydropyridine (MPTP) induced oxidative stress in mice brain, J. Funct. Foods, 2016, 25, 385–396 CrossRef CAS.
- A. F. Domenichiello, A. P. Kitson, C. T. Chen, M. O. Trpanier, P. M. Stavro and R. P. Bazinet, The effect of linoleic acid on the whole body synthesis rates of polyunsaturated fatty acids from α-linolenic acid and linoleic acid in free-living rats, J. Nutr. Biochem., 2016, 30, 167–176 CrossRef CAS PubMed.
- A. P. Kitson, A. H. Metherel, C. T. Chen, A. F. Domenichiello, M. O. Trpanier, A. Berger and R. P. Bazinet, Effect of dietary docosahexaenoic acid (DHA) in phospholipids or triglycerides on brain DHA uptake and accretion, J. Nutr. Biochem., 2016, 33, 91–102 CrossRef CAS PubMed.
- S. Yamashita, M. Hashimoto, A. M. Haque, K. Nakagawa, M. Kinoshita, O. Shido and T. Miyazawa, Oral Administration of Ethanolamine Glycerophospholipid Containing a High Level of Plasmalogen Improves Memory Impairment in Amyloid β-Infused Rats, Lipids, 2017, 1–11 Search PubMed.
- G. Bitan, M. D. Kirkitadze, A. Lomakin, S. S. Vollers, G. B. Benedek and D. B. Teplow, Amyloid β-Protein (Aβ) Assembly: Aβ40 and Aβ42 Oligomerize through Distinct Pathways, Proc. Natl. Acad. Sci. U. S. A., 2003, 100, 330–335 CrossRef CAS PubMed.
- L. Shi, J. Chen, J. Yang, T. Pan, S. Zhang and Z. Wang, MiR-21 protected human glioblastoma U87MG cells from chemotherapeutic drug temozolomide induced apoptosis by decreasing Bax/Bcl-2 ratio and caspase-3 activity, Brain Res., 2010, 1352, 255–264 CrossRef CAS PubMed.
- F. G. De Felice, P. T. Velasco, M. P. Lambert, K. Viola, S. J. Fernandez, S. T. Ferreira and W. L. Klein, Abeta oligomers induce neuronal oxidative stress through an N-methyl-D-aspartate receptor-dependent mechanism that is blocked by the Alzheimer drug memantine, J. Biol. Chem., 2007, 282, 11590–11601 CrossRef CAS PubMed.
- H. Park and M. M. Poo, Neurotrophin regulation of neural circuit development and function, Nat. Rev. Neurosci., 2013, 14, 7–23 CrossRef CAS PubMed.
- L. O. Hanuš, D. O. Levitsky, I. S. Valery and M. Dembitsky, Plasmalogens, fatty acids and alkyl glyceryl ethers of marine and freshwater clams and mussels, Food Chem., 2009, 116, 491–498 CrossRef.
- R. Yan and R. Vassar, Targeting the β secretase BACE1 for Alzheimer's disease therapy, Lancet Neurol., 2014, 13, 319–329 CrossRef CAS PubMed.
- L. Tapiaarancibia, E. Aliaga, M. Silhol and S. Arancibia, New insights into brain BDNF function in normal aging and Alzheimer disease, Brain Res. Rev., 2008, 59, 201–220 CrossRef CAS PubMed.
- W. Y. Wang, M. S. Tan, J. T. Yu and L. Tan, Role of pro-inflammatory cytokines released from microglia in Alzheimer's disease, Ann. Transl. Med., 2015, 3, 136 Search PubMed.
- H. Misonou, M. Morishima-Kawashima and Y. Ihara, Oxidative stress induces intracellular accumulation of amyloid beta-protein (Abeta) in human neuroblastoma cells, Biochemistry, 2000, 39, 6951–6959 CrossRef CAS PubMed.
- F. Echeverra, M. Ortiz, R. Valenzuela and L. A. Videla, Long-chain polyunsaturated fatty acids regulation of PPARs, signaling: Relationship to tissue development and aging, Prostag. Leukotr. Ess., 2016, 114, 28–34 CrossRef PubMed.
- S. Yamashita, A. Honjo, M. Aruga, K. Nakagawa and T. Miyazawa, Preparation of marine plasmalogen and selective identification of molecular species by LC-MS/MS, J. Oleo Sci., 2014, 63, 423–430 CrossRef CAS PubMed.
- T. T. Zhang, W. Li, G. Meng, P. Wang and W. Liao, Strategies for transporting nanoparticles across the blood-brain barrier, Biomater. Sci., 2016, 4, 219 RSC.
- C. N. Serhan, J. Dalli, R. A. Colas, J. W. Winkler and C. Nan, Protectins and Maresins: New Pro-Resolving Families of Mediators in Acute Inflammation and Resolution Bioactive Metabolome, Biochim. Biophys. Acta, 2015, 1851, 397 CrossRef CAS PubMed.
- L. Shi, J. Chen, J. Yang, T. Pan, S. Zhang and Z. Wang, MiR-21 protected human glioblastoma U87MG cells from chemotherapeutic drug temozolomide induced apoptosis by decreasing Bax/Bcl-2 ratio and caspase-3 activity, Brain Res., 2010, 1352, 255–264 CrossRef CAS PubMed.
- H. M, N. J, J. M, H. C, T. V, W.-M. S, W. M, S.-B. W, T. A, H. A and S. t. S, Cathepsin D links TNF-induced acid sphingomyelinase to Bid-mediated caspase-9 and -3 activation, Cell Death Differ., 2004, 11, 550 CrossRef PubMed.
- A. Hedengran, P. B. Szecsi, J. Dyerberg, W. S. Harris and S. Stender, n-3 PUFA Esterified to Glycerol or as Ethyl Esters Reduce Non-Fasting Plasma Triacylglycerol in Subjects with Hypertriglyceridemia: A Randomized Trial, Lipids, 2015, 50, 165 CrossRef CAS PubMed.
- P. Saravanan, N. C. Davidson, E. B. Schmidt and P. C. Calder, Cardiovascular effects of marine omega-3 fatty acids, Lancet, 2010, 376, 540 CrossRef CAS.
- K. Nagao, K. Nakamitsu, H. Ishida, K. Yoshinaga, T. Nagai, H. Mizobe, K. Kojima, T. Yanagita, F. Beppu and N. Gotoh, A comparison of the lipid-lowering effects of four different n-3 highly unsaturated fatty acids in HepG2 cells, J. Oleo Sci., 2014, 63, 979–985 CrossRef CAS PubMed.
- J. Kim, S. Lee, B. R. Choi, H. Yang, Y. Hwang, J. H. Park, F. M. Laferla, J. S. Han, K. W. Lee and J. Kim, Sulforaphane epigenetically enhances neuronal BDNF expression and TrkB signaling pathwaysffig, Mol. Nutr. Food Res., 2016, 61, 1–13 Search PubMed.
- J. G. Robinson and N. J. Stone, Antiatherosclerotic and antithrombotic effects of omega-3 fatty acids, Am. J. Cardiol., 2006, 98, 39i CrossRef CAS PubMed.
|
This journal is © The Royal Society of Chemistry 2018 |