DOI:
10.1039/C7FO01323J
(Review Article)
Food Funct., 2018,
9, 42-52
Molecular targets and mechanisms of bioactive peptides against metabolic syndromes
Received
26th August 2017
, Accepted 8th November 2017
First published on 9th November 2017
Abstract
Bioactive peptides are present in all living organisms and have critical roles ranging from protection against infection as the key element of innate immunity, regulating blood pressure and glucose levels, to reducing signs of ageing by killing senescent cells. Bioactive peptides are also encrypted within food protein sequences that can be released during proteolysis or food processing. These specific food protein fragments are reported to have potential for improving human health and preventing metabolic diseases through their impact on inflammation, blood pressure, obesity, and type-2 diabetes. This review mainly focuses on the molecular targets and the underlying mechanisms of bioactive peptides against various metabolic syndromes including inflammation, high blood pressure, obesity, and type-2 diabetes, to provide new insights and perspectives on the potential of bioactive peptides for management of metabolic syndromes.
1. Introduction
The prevalence of various chronic diseases, such as cardiovascular disease, chronic inflammation disease, cancers, diabetes, osteoporosis, etc., is increasing at an alarming rate worldwide.1 In addition to genetic factors, the onset and development of these chronic diseases are lifestyle-related, within which diet plays an important role.2–5 Many bioactive food components such as dietary fiber, omega-3 fatty acids, phytochemicals, and others are thought to have health-promoting properties.6 Food protein-derived bioactive peptides are increasingly recognized for their vast potential to improve human health and prevent chronic diseases via their impacts on various biochemical pathways.7
Numerous bioactive peptides have been identified from various kinds of food sources (as summarized in Table 1); soy, egg, milk, and fish are the most extensively studied proteins for preparing bioactive peptides. In addition to proteolysis, bioactive peptides can also be formed during food processing, in particular fermentation (i.e. His-His-Leu from soy paste,8 Met-Ala-Pro and Met-Lys-Pro from cheese9,10), or present naturally (i.e. lunasin, a 43-amino acid peptide identified from soy).11–13 Their diversified structures14 give bioactive peptides a broad scope of functions ranging from protection against infection as the key element of innate immunity, regulating blood pressure and glucose levels (Fig. 1), to reducing signs of ageing via killing senescent cells.15–17 The objective of this review is to provide an update on recent progress and perspectives regarding bioactive peptides against metabolic diseases and potential underlying mechanisms.
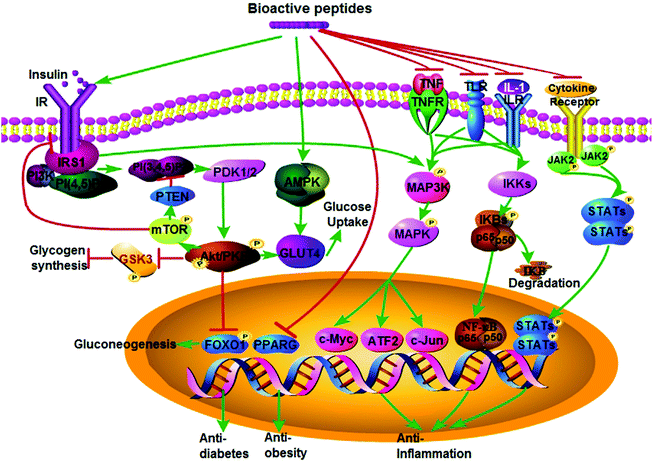 |
| Fig. 1 Proposed mechanisms of bioactive peptides on anti-inflammatory, anti-obesity, and anti-diabetic activities. (A) Bioactive peptides exert their anti-diabetic activity mainly via promoting insulin signaling and AMPK signaling pathways. They may act as an insulin mimic to activate the insulin receptor (IR) and insulin receptor substrate (IRS), followed by recruitment of PI3K to IRS; subsequently, activation of PI3K phosphorylates PKB/AKT via PDK1, which in turn promote glycogen synthesis through GSK3, or promote glucose uptake through GLUT4, or inhibit gluconeogenesis through FOXO1. However, whether the feedback from excessive activation of mTORC1 signaling by over-nutrition in obesity could inhibit PI3K through PTEN or the interaction between IR and IRS or not requires further investigation. Bioactive peptides also may stimulate glucose uptake through activating the AMPK pathway and then promoting GLUT4 translocation to the cell membrane. (B) Bioactive peptides exert their anti-obesity activity via inhibiting expression of nuclear transcript factor PPARγ. (C) The anti-inflammatory activity of bioactive peptides is via inhibiting NF-κB, MAPK, and/or JAK-STAT pathways by inhibiting: (1) the stimulus (TNFα, IL-1, or LPS) and their corresponding receptor (TNFR, IL-1R, TLR)-mediated phosphorylation of MAPKKK and the downstream MAPK leading to inhibition of transcription factors (i.e. c-Myc, ATF-2 and c-Jun). (2) Activation of the IκB kinase (IKK) complex, which in turn limits phosphorylation of IκBα and its subsequent polyubiquitination, proteasomal degradation of IκBα as well as release of homo/heterodimers p50 and RelA (p65), resulting in inhibition of transcription of target genes for inflammatory response. (3) Cytokine-mediated phosphorylation of JAK and subsequent phosphorylation of STAT that in turn form the STAT-STAT dimer and translocation dimer to the nucleus, which need to be further validated. This figure was drawn using the Pathway Builder Tool (Version 2.0, http://www.proteinlounge.com). Abbreviations: AMPK, AMP-activated protein kinase; FOXO1, forkhead box O1; PTEN, phosphatase and tensin homologue; GLUT4, glucose transporter 4; GSK3, glycogen synthase kinase; IL-1, interleukin-1; LPS, lipopolysaccharides; IR, insulin receptor; IRS, IR substrate; JAK-STAT, janus kinase-signal transducer and activator of transcription; MAPK, mitogen activated protein kinase; MAPKKK, MAPK kinase kinase; PDK1, 3-phosphoinositide-dependent kinase; PI3K, phosphoinositide 3-kinase; PKB/AKT, protein kinase B; PPARγ, peroxisome proliferator-activated receptor γ; NF-κB, nuclear factor-kappa B; TNFα, tumor necrosis factor. | |
Table 1 Examples of food proteins derived bioactive peptides and their mechanisms of action
Bioactivities |
Protein source |
Peptide identifies |
Mechanisms of action |
Cells or animals |
Ref. |
Anti-inflammation peptides
|
Animal source |
Bovine casein |
Val-Pro-Pro (VPP); Ile-Pro-Pro (IPP) |
• Inhibited NF-κB pathway |
• 3T3-F442A preadipocytes |
25 and 29
|
• Reduced adipokine levels |
• apolipoprotein E knockout mice |
Bovine casein |
VPP |
• Inhibited either MAPK-JNK pathway or renin–angiotensin system |
• Leukocyte and endothelium |
26 and 30
|
• Adipocyte and macrophage interactions |
Egg |
Ile-Arg-Trp (IRW) |
• Inhibited NF-κB pathway |
• Human endothelial cells |
31 and 32
|
Botanical source |
Marine algae |
Peptide from P. yezoensis |
• Inhibited MAPK pathways |
• RAW 264.7 |
35
|
Plant source |
Soy |
Val-Pro-Tyr |
• Reduced expression of TNF-α, IL-6, IL-1, and IL-17 |
• Caco-2 |
33
|
Soy |
lunasin |
• Reduced expression of Th2 cytokine |
• BALB/c mice |
34
|
|
Anti-hypertensive peptides
|
Plant sources |
Fermented soybean |
His-His-Leu |
• Decreased ACE activity in aorta |
• SHRs |
8
|
Sour milk |
VPP, IPP |
• Increased Mas receptor/NO mediated vasodilation |
• Endothelial cells |
47 and 48
|
• Reduced ACE activity |
• Isolated aortic rings |
Animal sources |
Ovotransferrin |
Ile-Gln-Trp, Leu-Lys-Pro |
• Increased NO-mediated vasodilation |
• Endothelial cells |
49–52
|
• Reduced vascular inflammation |
• SHRs |
Ovotransferrin |
IRW |
• Increased NO-mediated vasodilation |
• SHRs |
56
|
• Reduced vascular inflammation |
• Up-regulated ACE2 expression |
Bonito |
Leu-Lys-Pro-Asn-Met |
• A pro-type ACE inhibitory peptide |
• SHRs |
53
|
Sardine muscle |
Val-Tyr |
• Blocked the angiotensin II type 1 (AT1) receptor |
• Mild hypertensive subjects |
55
|
Lactoferrin |
Leu-Ile-Trp-Lys-Leu, Arg-Pro-Tyr-Leu |
• Ang II type 1 (AT1) receptor antagonist |
• Rabbits |
16
|
• Inhibition of angiotensin II-induced vasoconstriction |
• ACE inhibition |
|
Anti-obesity peptides
|
Animal sources |
Cheese whey |
Casein glycomacropeptides (GMP) |
• Inhibited proliferation and differentiation |
• Primary rat preadipocytes |
72
|
Tuna |
Asp-Ile-Val-Asp-Lys-Ile-Glu-Ile |
• Inhibited C/EBPs and PPARγ expression |
• 3T3-L1 preadipocytes |
73
|
• Inhibited adipocyte differentiation |
Milk |
IPP and VPP |
• Upregulated PPARγ and adiponectin |
• 3T3-F442A preadipocytes |
25
|
• Anti-inflammation |
milk κ-casein |
Caseinomacropeptide |
• Stimulated cholecystokinin release |
• Male wistar rats |
80
|
Plant sources |
Black soy |
Isoflavone-free peptide mixture (BSP) |
• Decreased appetite |
• Diet-induced obese (DIO) mice |
77
|
• Activated AMPK and STAT3 phosphorylation |
• C2C12 myocytes |
Soy protein β-conglycinin |
Val-Arg-Ile-Arg-Leu-Leu-Gln-Arg-Phe-Asn-Lys-Arg-Ser |
• Suppressed appetite |
• Male Sprague-Dawley rats |
78
|
• Stimulated cholecystokinin (CCK) release |
|
Anti-type 2 diabetes peptides
|
Plant sources |
Soybean |
Aglycin |
• Maintained IR and IRS1 expression |
• Type 2 diabetic mice |
106
|
• Elevated phosphorylation of IR, IRS1, and AKT |
• Increased translocation of GLUT4 to cell membrane |
Soybean |
Trp-His |
• Activated AMPK in an insulin-independent way |
• L6 muscle cells |
118 and 119
|
• Increased GLUT4 translocation to plasma membrane |
Animal sources |
Bovine milk casein glycomacropeptide |
Ile-Pro-Pro-Lys-Lys-Asn-Gln-Asp-Lys-Thr-Glu |
• Increased phosphorylation of Akt and AMPK |
• HepG2 cells |
117
|
• Activated both insulin and AMPK signaling pathways |
2 Bioactive peptides on human health
2.1 Bioactive peptides against inflammation
Inflammation is one of the body's defensive responses to stimuli, such as pathogen invasion, injury, tissue damage, or infection.17 Controlled inflammation is essential for normal body function; however, persistent, chronic inflammation at a low intensity is a causative factor for a wide range of chronic diseases such as obesity, type 2 diabetes, asthma, osteoporosis, inflammatory bowel disease, cancer, central nervous system disease, and cardiovascular disease.17,18 Chronic inflammation is mediated mainly by the nuclear factor-kappa B (NF-κB) pathway, the mitogen activated protein kinase-c-jun N-terminal kinase (MAPK-JNK) pathway, and the janus kinase-signal transducer and activator of transcription (JAK-STAT) pathway, usually under the stimuli of tumor necrosis factor α (TNFα), interleukin-1 (IL-1), and lipopolysaccharides (LPS).19–21 In the canonical NF-κB signaling pathway, the stimulus (TNFα, IL-1, or LPS) binds to its respective receptor (tumor necrosis factor receptor, TNFR, interleukin-1 receptor, IL-1R, or Toll-like receptors, TLRs) leading to activation of the IκB kinase (IKK) complex in the cytoplasm, which in turn phosphorylates IκBα followed by its subsequent polyubiquitination, proteasomal degradation of IκBα, and then release of the homo/heterodimers p50 and RelA (also known asp65); after translocation to the nucleus, these dimers activate transcription of target genes for the inflammatory response.22 In the MAPK pathway, phosphorylation and activation of MAPK kinase kinase (MAPKKK) leads to phosphorylation of MAPK kinase (MAPKK), which in turn mediates phosphorylation of MAPKs (including extracellular signal-regulated kinase, ERK, JNK, and p38). Activated MAPKs then phosphorylate a variety of transcription factors (i.e. c-Myc, ATF-2, and c-Jun) leading to diverse cellular activities such as cell proliferation, migration, differentiation, and survival for the ERK1/2 signaling pathway; autophagy, apoptosis stress, and inflammation for the JNK and p38 signaling pathways.23 Similarly, when cytokines bind to their corresponding receptors, JAKs are activated leading to phosphorylation of STAT and formation of STAT-STAT dimer. Subsequently, the dimer is translocated to the nucleus and regulates specific gene expression by directly binding to DNA resulting in the inflammatory response.24
Several food-derived bioactive peptides are reported to have anti-inflammatory activities both in in vitro cellular systems and in in vivo animal models.25–28 Milk-derived tripeptides Val-Pro-Pro (VPP) and Ile-Pro-Pro (IPP) showed anti-inflammation activity through inhibition of the NF-κB pathway, as well as increased release of adiponectin in adipocytes,25 contributing to their protective roles against atherosclerotic development in apolipoprotein E knockout mice.29 Furthermore, VPP's anti-inflammatory effect was reported to be mediated through inhibition of either the MAPK-JNK pathway in leukocyte and endothelium or the renin–angiotensin system (RAS) in adipocyte and macrophage interactions.26,30 Egg-derived tripeptide Ile-Arg-Trp (IRW)'s anti-inflammatory activity also acted through the NF-κB pathway.31,32 Other anti-inflammatory peptides are Val-Pro-Tyr (VPY) and lunasin from soy, and peptide from P. yezoensis (PPY1, a marine algae).33–35 Further information on anti-inflammatory peptides has been recently reviewed.17,25 Given the underlying role of chronic inflammation in many types of chronic diseases, anti-inflammatory peptides identified from food proteins may have a potential role against a wide range of chronic diseases. A number of anti-inflammatory functional foods have been marketed successfully (i.e. lactoferrin from milk whey protein and sesquiterpenoid from Inula plants), providing insight into developing novel peptides against chronic diseases.36–39 However, there is lack of understanding regarding specific peptide receptors and the associated signaling pathways that mediate their anti-inflammation activities; furthermore, crosstalk among RAS components and these signaling pathways requires further elucidation.
2.2 Bioactive peptides against high blood pressure
Most bioactive peptides have been characterized as inhibitors of angiotensin converting enzyme (ACE),8,40–44 a key enzyme for regulation of blood pressure, water, and salt balance in RAS.45 ACE is responsible for conversion of inactive decapeptide angiotensin I (Ang I) into a vasoconstrictive octapeptide, angiotensin II (Ang II), a major component in adverse blood pressure regulation. Ang II has two main receptors, angiotensin type 1 receptor (AT1) and angiotensin type 2 receptor (AT2).46 Binding of angiotensin II to the AT1 receptor causes vasoconstriction and high blood pressure. RAS has been extensively explored as the target for development of antihypertensive drugs.
Well-studied ACE inhibitory peptides include Val-Pro-Pro and Ile-Pro-Pro from sour milk,47–49 Ile-Arg-Trp, Ile-Gln-Trp, and Leu-Lys-Pro from egg protein ovotransferrin,50–52 and Leu-Lys-Pro-Asn-Met and Val-Tyr from fish.53–55 Although most of these peptides have been identified as ACE inhibitors, their mechanisms of action in vivo are more complicated than initially expected. It was revealed that lactoferrin-derived peptides Leu-Ile-Trp-Lys-Leu and Arg-Pro-Tyr-Leu can selectively inhibit angiotensin II-induced vasoconstriction by blocking AT1 receptors.16 Apart from binding to the AT1 receptor, Ang II can also be converted into Ang (1–7) by ACE2, a homologous enzyme to ACE; Ang-(1–7) binds to the G-protein-coupled receptor (GPCR)-MAS, showing a vasodilatory effect.45 Our group demonstrated for the first time that ACE2 is involved with the blood pressure-lowering activity of the ovotransferrin-derived ACE inhibitory peptide Ile-Arg-Trp in SHRs through upregulation of ACE2 gene expression.56 Molecular targets,57 as well as progress on understanding the mechanisms of ACE inhibitory peptides, have been recently reviewed.57,58 Given the complexity of the pathophysiology of hypertension, it seems that many ACE inhibitory peptides function beyond ACE inhibition in vivo.
2.3 Bioactive peptides against obesity
According to the World Health Organization (WHO), an individual with a body mass index (BMI, weight divided by height square) of 30.0 kg m−2 or greater is obese;59 BMI ranging from 25.0 to 29.9 kg m−2 is classified as overweight. Obese individuals often exhibit abdominal and upper body obesity and have larger waist circumferences (men ≥102 cm, women ≥88 cm); abdominal obesity is closely associated with disorders such as hyperglycemia, type 2 diabetes, hypertension, dyslipidemia, gallbladder disease, coronary heart disease (CHD), and cancer.60–62 Obesity develops when energy intake exceeds energy expenditure resulting from reduced physical exercise and sedentary lifestyle. The energy imbalance contributes to adipose dysfunction and development of obesity and relevant complications. Adipocyte dysfunction is manifest by an excessively increased number and size of adipocytes (hyperplasia and hypertrophy).63 Adipogenesis is regulated by peroxisome proliferator-activated receptor γ (PPARγ) (encoded by PPARG) and CCAAT/enhancer-binding protein α/β/δ (C/EBPα/β/δ);64 activation of PPARγ promotes differentiation of preadipocytes into mature adipocytes.65 Therefore, a potential target for obesity control is reducing adipogenesis by inhibiting the activity of PPARγ or reducing preadipocyte differentiation.66 A synthetic PPARγ antagonist, bisphenol A diglycidyl ether, has been demonstrated to inhibit adipogenesis in preadipocytes 3T3-L1 and 3T3-F442A, which may exert some positive effects in treatment of obesity.67 Other synthetic PPARγ antagonists, such as HX531,68 GW-9962, and LG100641,69 have also been investigated for their potential uses against diet-induced obesity.
There is interest in exploring food components such as peptides or protein hydrolysates as potential alternatives for prevention of obesity. Whey protein isolate has been reported to inhibit expression of PPARγ and lipid accumulation in 3T3-L1 preadipocytes, and also to significantly reduce weight gain in rats fed high-fat diets.70 A soy protein hydrolysate71 and milk casein glycomacropeptides (GMP)72 have also been demonstrated to inhibit fat accumulation in primary and 3T3-L1 adipocytes, respectively. Recently, a peptide Asp-Ile-Val-Asp-Lys-Ile-Glu-Ile derived from boiled tuna was found to decrease glucose uptake and triglyceride (TG) levels, and inhibit C/EBPs and PPARγ levels in 3T3-L1 cells, indicating its potential use against obesity.73
As an endocrine agent, adipocytes secrete a variety of adipocytokines such as leptin, resistin, and adiponectin, which are important regulators of relevant metabolism.65 It has been reported that the plasma concentration of adiponectin in both men and women correlates negatively with BMI74 and visceral adiposity.75 Even though the specific modes of action for PPARγ activator thiazolidinediones (TZDs) such as rosiglitazone to elicit insulin sensitivity and weight gain remain under debate, moderate activation of PPARγ leading to increased adiponectin is considered to be a potential strategy for treating excessive visceral adiposity.61 Accordingly, the aforementioned lactotripeptides Val-Pro-Pro and Ile-Pro-Pro can also induce beneficial adipocyte differentiation, proper upregulation of PPARγ, and secretion of the protective lipid adipokine adiponectin in 3T3-F442A cells,25 indicating their potential role against obesity. A soy protein diet has been reported to increase adiponectin mRNA level in adipose tissue and adiponectin plasma concentration in obese mice,76 which may exert some positive effects to improve obesity. Yamauchi et al.68 indicated that either by reducing adipocyte differentiation and apoptosis or by upregulation of adiponectin, both moderate reduction of PPARγ activity and PPARγ activation could ameliorate insulin resistance, associated with decreased triglyceride content in muscle or liver and prevention of adipocyte hypertrophy. Thus, a crosstalk and coordinated role may exist between PPARγ and the various adipocytokines such as adiponectin to promote insulin sensitivity.
Apart from modulation of adipose biology to lower adiposity, bioactive peptides could also regulate energy balance via increasing satiety, reducing appetite, and increasing energy expenditure to lower food intake and weight gain. A black soybean protein-derived isoflavone-free peptide mixture was reported to reduce body weight and ameliorate hypercholesterolemia in high fat diet-induced obese rats via decreasing appetite and regulating whole-body energy balance.77 A peptide Val-Arg-Ile-Arg-Leu-Leu-Gln-Arg-Phe-Asn-Lys-Arg-Ser derived from soy protein β-conglycinin was demonstrated to decrease body fat and food intake via stimulated release of cholecystokinin (CCK) and thus suppression of appetite.78 Milk casein-derived casomorphins with opioid-like activities can interact with gastric opioid receptors to slow gastrointestinal motility to regulate appetite.79 Other milk proteins such as caseinoglycomacropeptide80 and whey protein81 also have been found to stimulate CCK release and activate the glucagon-like peptide-1(GLP-1) pathway, respectively, to increase satiety while suppressing appetite. Protein is the most satiating macronutrient. A high-protein diet particularly enriched with β-lactoglobulin has been reported to reduce body weight;82 it is not known, however, whether peptides released during gastrointestinal digestion might be responsible for the benefit.
2.4 Bioactive peptides against type-2 diabetes (T2D)
According to Diabetes Canada, “Type 2 diabetes is a disease in which your pancreas does not produce enough insulin, or your body does not properly use the insulin it makes” (http://www.diabetes.ca). Type 2 diabetes (T2D) affects 422 million adults worldwide and this number is expected to reach 592 million by 2035.83,84 In addition to genetic factors, modern sedentary lifestyle and overnutrition are closely associated with the onset and development of T2D.85 As discussed above, obesity is a key contributor to the development of T2D because of prolonged and chronic exposure to excessive nutrition.85 In normal glucose homeostasis, an increase in plasma glucose concentration after a meal stimulates insulin release, thus enhancing uptake of glucose by the liver, gut, and peripheral tissues (i.e. muscle), and also suppressing hepatic glucose production; however, this dynamic balance is disturbed in T2D.86 Pathogenesis of T2D is characterized by impaired insulin secretion and/or insulin resistance.87 Impaired insulin secretion resulting from β-cell loss and dysfunction may occur because of amyloid deposition in pancreatic islets, β-cell differentiation, glucotoxicity, and lipotoxicity,87 while insulin resistance is an impaired response of target organs (adipose tissue, skeletal muscle, and liver) to insulin-mediated actions, such as inadequate suppression of hepatic glucose production, and reduced insulin-mediated glucose uptake and utilization in skeletal muscle and adipose tissue.88,89
The cellular and molecular mechanisms of T2D are associated with insulin signaling, mammalian target of rapamycin (mTOR), and AMP-activated protein kinase (AMPK) signaling pathways.90,91 Under physiological conditions, insulin stimulates phosphorylation of the insulin receptor (IR) and IR substrate (IRS), followed by recruitment of phosphoinositide 3-kinase (PI3K) to IRS. Subsequently, activation of PI3K phosphorylates protein kinase B (PKB/AKT) and atypical protein kinase C isoforms (aPKCs) via 3-phosphoinositide-dependent kinase (PDK1).92 However, in a state of chronic hyperglycemia, a defect of IR or post-receptor (i.e. IRS1, IRS2, and PI3K) may lead to a series of metabolic disorders including (1) decreased glucose transporter 4 (GLUT4)-dependent glucose uptake,90 (2) reduced glycogen synthesis through glycogen synthase kinase (GSK3) or salt-inducible kinase 2 (SIK2),90 (3) increased gluconeogenesis through forkhead box O1 (FOXO1),90 and (4) limited protein synthesis through mTORC1-S6K1 axis in diabetes.90 Furthermore, increased activity of extracellular signal-regulated kinase (ERK) in adipocytes and adipose tissue is associated with T2D pathogenesis.93 Feedback to the insulin pathway also contributes to regulation of glucose homeostasis. Albeit AKT-mTORC1 signaling is limited in diabetes, excessive activation of mTORC1 signaling by over-supplied nutrients in obesity could (1) inhibit IRS and its interaction with IR through growth factor receptor-bound protein 10 (GRB10) phosphorylation;90,94,95 and (2) inhibit phosphatidylinositol 3,4,5-trisphosphate (PIP3) via hypoxia inducible factor 1α (HIF1α) and phosphatase and tensin homologue (PTEN).90,96
It appears that a number of peptides (including proteins) and phytochemicals can enhance insulin sensitivity via multiple signaling pathways.97,98 For instance, Momordica charantia (bitter melon, a traditional medicine used for treatment of T2D) alone and in its powder form can reduce insulin resistance through suppression of NF-κB and JNK/p38 MAPKs pathways in liver, muscle, and adipose tissues in obese and diabetic rats.99,100 A cucurbitane-type triterpenoid enhanced the insulin signaling pathway, GLUT4 expression, and MAPK activation.101 Momordicosides increase glucose uptake through stimulating translocation of GLUT4 to the cell membrane.102Momordica charantia insulin receptor-binding protein (mcIRBP)-derived peptides, mcIRBP-19 and mcIRBP-68, enhance insulin binding to IR, stimulating phosphorylation of AKT and expression of GLUT4, resulting in increased glucose uptake in adipocytes and glucose clearance in diabetic mice.103–105 Soy protein also shows anti-diabetic activities. Aglycin isolated from soybean can improve glucose uptake via elevated phosphorylation of IR, IRS1, and AKT, as well as translocation of GLUT4 to cell membrane.106 Recent progress has been reviewed on the potential of anti-diabetic plants on improving insulin sensitivities.107 However, the link between food-derived bioactive peptides and mTOR signaling, as well as their feedback to insulin signaling, has not been explored.
In addition, AMPK activation was recently proposed as a potential therapeutic target for prevention and amelioration of insulin resistance and T2D.91,108 A Ca2+-dependent pathway mediated by Ca2+/calmodulin-dependent protein kinase kinase β (CaMKKβ) and a low energy level (high AMP/ATP or ADP/ATP ratio) mediated by serine/threonine kinase II (LKB1), phosphorylated and then activated AMPK.109–111 Activation of AMPK phosphorylates AS160 (a 160 kDa Akt substrate), resulting in reduced activity of Rab guanosine triphosphatase (GTPase) but increased GTP load on the storage sites of GLUT4, therefore promoting GLUT4 translocation to cell membrane, which ultimately stimulates glucose uptake in skeletal muscle and adipocytes.112–116 Some bioactive peptides or protein hydrolysates have been demonstrated to improve insulin sensitivity through activating insulin and/or AMPK signaling pathways. For instance, a peptide Ile-Pro-Pro-Lys-Lys-Asn-Gln-Asp-Lys-Thr-Glu derived from casein glycomacropeptide prevented high glucose-induced insulin resistance in hepG2 cells by activating both insulin and AMPK signaling pathways via phosphorylation of Akt and AMPK.117 A dipeptide Trp-His (WH) and a soybean hydrolysate fraction prepared by electrodialysis enhanced glucose uptake in L6 muscle cells through activation of AMPK in an insulin-independent way and enhanced GLUT4 translocation to the plasma membrane, respectively.118,119 Soy β-conglycinin and soybean peptides improved glucose uptake and insulin sensitivity through activation of AMPK in skeletal muscle in Goto-Kakizaki rats.118,120,121
For T2D patients, a normal plasma glucose level either in the fasting or postprandial states is of great importance. Postprandial plasma glucose level is considered to be a better marker of glycemic control than fasting plasma glucose levels.122 Postprandial plasma level is regulated mainly by α-glucosidase and ubiquitous enzyme dipeptidyl peptidase (DPP)-IV. Inhibitors of α-glucosidase and DPP-IV have been explored extensively for potential for management of T2D.122 Research progress in this field has been reviewed.123
Given the complex pathophysiology of T2D, there are multiple targets for bioactive peptides, such as enhancing insulin secretion, potentiating insulin and/or AMPK signaling, and/or inhibiting inflammation, and improved GLUT4 expression and translocation to membrane.87,93,117,124–127 In addition to these targets, the crosstalk among insulin, AMPK, and mTOR signaling pathway is also a key treatment aspect for T2D management in response to anti-diabetes peptides.
3 Conclusions
Bioactive peptides have beneficial effects on blood pressure, inflammation, obesity, and T2D, indicating great promise as functional foods/nutraceuticals against metabolic syndromes. Bioactive peptides such as Leu-Lys-Pro-Asn-Met and Val-Tyr have been commercialized.54 However, use of bioactive peptides has been criticized because their susceptibility to gastrointestinal proteases and peptidase could render them inactive prior to reaching sites of action, despite many bioactive peptides being proved bioavailable and bioactive in vivo. Recent progress on characterization of various target pathways indicates that bioactive peptides might function locally through receptors on cell membranes. This signifies the importance of further research to explore their mechanisms of action in vivo. Integration of advanced omics technologies with bioinformatic analysis is expected to shed light on the novel pathways of action of the peptides in vivo. It would also be worthwhile studying the effects of bioactive peptides on “master regulators” or “central targets” (i.e. MAPK) and “specific targets” (i.e. IR for T2D, PPAR for obesity) to understand the multifunctional nature of many bioactive peptides and the crosstalk that may be involved. There is an urgent need for translational research so that beneficial bioactive peptides can be made commercially available.
Conflicts of interest
No conflicts of interest.
Acknowledgements
This work was supported by National Key R&D Program of China (2017YFD0400600) and Natural Sciences and Engineering Research Council of Canada.
References
- P. Nichols, A. Ussery-Hall, S. Griffin-Blake and A. Easton, The evolution of the steps program, 2003–2010: transforming the federal public health practice of chronic disease prevention, Prev. Chronic Dis., 2012, 9, E50 Search PubMed.
- X. Y. Xu, J. Hall, J. Byles and Z. M. Shi, Dietary Pattern Is Associated with Obesity in Older People in China: Data from China Health and Nutrition Survey (CHNS), Nutrients, 2015, 7, 8170–8188 CrossRef PubMed.
- E. P. Lopez, C. Rice, D. O. Weddle and G. J. Rahill, The relationship among cardiovascular risk factors, diet patterns, alcohol consumption, and ethnicity among women aged 50 years and older, J. Am. Diet. Assoc., 2008, 108, 248–256 CrossRef CAS PubMed.
- X. Y. Zhang, L. Shu, C. J. Si, X. L. Yu, W. Gao, D. Liao, L. Zhang, X. L. Liu and P. F. Zheng, Dietary Patterns and Risk of Stroke in Adults: A Systematic Review and Meta-analysis of Prospective Cohort Studies, J. Stroke Cerebrovasc. Dis., 2015, 24, 2173–2182 CrossRef PubMed.
- D. M. A. McCartney, D. G. Byrne and M. J. Turner, Dietary contributors to hypertension in adults reviewed, Ir. J. Med. Sci., 2015, 184, 81–90 CrossRef CAS PubMed.
- P. Zimmet, K. G. Alberti and J. Shaw, Global and societal implications of the diabetes epidemic, Nature, 2001, 414, 782–787 CrossRef CAS PubMed.
- K. Majumder, Y. Mine and J. P. Wu, The potential of food protein-derived anti-inflammatory peptides against various chronic inflammatory diseases, J. Sci. Food Agric., 2016, 96, 2303–2311 CrossRef CAS PubMed.
- Z. I. Shin, R. Yu, S. A. Park, D. K. Chung, C. W. Ahn, H. S. Nam, K. S. Kim and H. J. Lee, His-His-Leu, an angiotensin I converting enzyme inhibitory peptide derived from Korean soybean paste, exerts antihypertensive activity in vivo, J. Agric. Food Chem., 2001, 49, 3004–3009 CrossRef CAS PubMed.
- H. Tonouchi, M. Suzuki, M. Uchida and M. Oda, Antihypertensive effect of an angiotensin converting enzyme inhibitory peptide from enzyme modified cheese, J. Dairy Res., 2008, 75, 284–290 CrossRef CAS PubMed.
- A. Yamada, T. Sakurai, D. Ochi, E. Mitsuyama, K. Yamauchi and F. Abe, Novel angiotensin I-converting enzyme inhibitory peptide derived from bovine casein, Food Chem., 2013, 141, 3781–3789 CrossRef CAS PubMed.
- V. P. Dia, W. Wang, V. L. Oh, B. O. de Lumen and E. G. de Mejia, Isolation, purification and characterisation of lunasin from defatted soybean flour and in vitro evaluation of its anti-inflammatory activity, Food Chem., 2009, 114, 108–115 CrossRef CAS.
- E. G. de Mejia and V. P. Dia, Lunasin and lunasin-like peptides inhibit inflammation through suppression of NF-kappa B pathway in the macrophage, Peptides, 2009, 30, 2388–2398 CrossRef PubMed.
- A. Cam and E. G. de Mejia, RGD-peptide lunasin inhibits Akt-mediated NF-κB activation in human macrophages through interaction with the alpha V beta 3 integrin, Mol. Nutr. Food Res., 2012, 56, 1569–1581 CAS.
- C. Udenigwe and R. E. Aluko, Food protein-derived bioactive peptides: production, processing, and potential health benefits, J. Food Sci., 2012, 77, R11–R24 CrossRef CAS PubMed.
- J. Y. Lee, Y. S. Choi, S. J. Lee, C. P. Chung and Y. J. Park, Bioactive peptide-modified biomaterials for bone regeneration, Curr. Pharm. Des., 2011, 17, 2663–2676 CrossRef CAS PubMed.
- R. Fernandez-Musoles, M. Castello-Ruiz, C. Arce, P. Manzanares, M. D. Ivorra and J. B. Salom, Antihypertensive Mechanism of Lactoferrin-Derived Peptides: Angiotensin Receptor Blocking Effect, J. Agric. Food Chem., 2014, 62, 173–181 CrossRef CAS PubMed.
- K. Majumder, Y. Mine and J. P. Wu, The potential of food protein-derived anti-inflammatory peptides against various chronic inflammatory diseases, J. Sci. Food Agric., 2016, 96, 2303–2311 CrossRef CAS PubMed.
- C. Nathan and A. Ding, Nonresolving inflammation, Cell, 2010, 140, 871–882 CrossRef CAS PubMed.
- D. Laveti, M. Kumar, R. Hemalatha, R. Sistla, V. G. Naidu, V. Talla, V. Verma, N. Kaur and R. Nagpal, Anti-inflammatory treatments for chronic diseases: a review, Inflammation Allergy: Drug Targets, 2013, 12, 349–361 CrossRef CAS PubMed.
- J. M. Kyriakis and J. Avruch, Mammalian MAPK signal transduction pathways activated by stress and inflammation: a 10-year update, Physiol. Rev., 2012, 92, 689–737 CrossRef CAS PubMed.
- M. H. Kaplan, STAT signaling in inflammation, Jak-Stat, 2013, 2, e24198 CrossRef PubMed.
- B. Hoesel and J. A. Schmid, The complexity of NF-kappaB signaling in inflammation and cancer, Mol. Cancer, 2013, 12, 86 CrossRef CAS PubMed.
- E. K. Kim and E. J. Choi, Compromised MAPK signaling in human diseases: an update, Arch. Toxicol., 2015, 89, 867–882 CrossRef CAS PubMed.
- J. J. O'Shea, D. M. Schwartz, A. V. Villarino, M. Gadina, I. B. McInnes and A. Laurence, The JAK-STAT pathway: impact on human disease and therapeutic intervention, Annu. Rev. Med., 2015, 66, 311–328 CrossRef PubMed.
- S. Chakrabarti and J. P. Wu, Milk-Derived Tripeptides IPP (Ile-Pro-Pro) and VPP (Val-Pro-Pro) Promote Adipocyte Differentiation and Inhibit Inflammation in 3T3-F442A Cells, PLoS One, 2015, 10, e0117492 Search PubMed.
- Y. Sawada, Y. Sakamoto, M. Toh, N. Ohara, Y. Hatanaka, A. Naka, Y. Kishimoto, K. Kondo and K. Iida, Milk-derived peptide Val-Pro-Pro (VPP) inhibits obesity-induced adipose inflammation via an angiotensin-converting enzyme (ACE) dependent cascade, Mol. Nutr. Food Res., 2015, 59, 2502–2510 CAS.
- D. P. Mohanty, S. Mohapatra, S. Misra and P. S. Sahu, Milk derived bioactive peptides and their impact on human health - A review, Saudi J. Biol. Sci., 2016, 23, 577–583 CrossRef CAS PubMed.
- S. Marcone, O. Belton and D. J. Fitzgerald, Milk-derived bioactive peptides and their health promoting effects: a potential role in atherosclerosis, Br. J. Clin. Pharmacol., 2017, 83, 152–162 CrossRef CAS PubMed.
- T. Nakamura, T. Hirota, K. Mizushima, K. Ohki, Y. Naito, N. Yamamoto and T. Yoshikawa, Milk-Derived Peptides, Val-Pro-Pro and Ile-Pro-Pro, Attenuate Atherosclerosis Development in Apolipoprotein E-Deficient Mice: A Preliminary Study, J. Med. Food, 2013, 16, 396–403 CrossRef CAS PubMed.
- K. Aihara, H. Ishii and M. Yoshida, Casein-Derived Tripeptide, Val-Pro-Pro (VPP), Modulates Monocyte Adhesion to Vascular Endothelium, J. Atheroscler. Thromb., 2009, 16, 594–603 CrossRef CAS PubMed.
- K. Majumder, S. Chakrabarti, S. T. Davidge and J. Wu, Structure and activity study of egg protein ovotransferrin derived peptides (IRW and IQW) on endothelial inflammatory response and oxidative stress, J. Agric. Food Chem., 2013, 61, 2120–2129 CrossRef CAS PubMed.
- W. Y. Huang, S. Chakrabarti, K. Majumder, Y. Y. Jiang, S. T. Davidge and J. P. Wu, Egg-Derived Peptide IRW Inhibits TNF-alpha-Induced Inflammatory Response and Oxidative Stress in Endothelial Cells, J. Agric. Food Chem., 2010, 58, 10840–10846 CrossRef CAS PubMed.
- J. Kovacs-Nolan, H. Zhang, M. Ibuki, T. Nakamori, K. Yoshiura, P. V. Turner, T. Matsui and Y. Mine, The PepT1-transportable soy tripeptide VPY reduces intestinal inflammation, Biochim. Biophys. Acta, Gen. Subj., 2012, 1820, 1753–1763 CrossRef CAS PubMed.
- E. J. McConnell, B. Devapatla, K. Yaddanapudi and K. R. Davis, The soybean-derived peptide lunasin inhibits non-small cell lung cancer cell proliferation by suppressing phosphorylation of the retinoblastoma protein, Oncotarget, 2015, 6, 4649–4662 Search PubMed.
- H. A. Lee, I. H. Kim and T. J. Nam, Bioactive peptide from Pyropia yezoensis and its anti-inflammatory activities, Int. J. Mol. Med., 2015, 36, 1701–1706 CrossRef CAS PubMed.
- B. I. Brown, Nutritional Management of Metabolic Endotoxemia: A Clinical Review, Altern. Ther. Health
Med., 2017, 23, 42–54 Search PubMed.
- N. A. Hering, J. Luettig, S. M. Krug, S. Wiegand, G. Gross, E. A. van Tol, J. D. Schulzke and R. Rosenthal, Lactoferrin protects against intestinal inflammation and bacteria-induced barrier dysfunction in vitro, Ann. N. Y. Acad. Sci, 2017, 1405, 177–188 CrossRef CAS PubMed.
- J. Qin, W. Wang and R. Zhang, Novel natural product therapeutics targeting both inflammation and cancer, Chin. J. Nat. Med., 2017, 15, 401–416 Search PubMed.
- D. J. Newman and G. M. Cragg, Natural Products as Sources of New Drugs from 1981 to 2014, J. Nat. Prod., 2016, 79, 629–661 CrossRef CAS PubMed.
- K. G. Mallikarjun Gouda, L. R. Gowda, A. G. Rao and V. Prakash, Angiotensin I-converting enzyme inhibitory peptide derived from glycinin, the 11S globulin of soybean (Glycine max), J. Agric. Food Chem., 2006, 54, 4568–4573 CrossRef CAS PubMed.
- T. Kodera and N. Nio, Identification of an Angiotensin I-converting Enzyme Inhibitory Peptides from Protein Hydrolysates by a Soybean Protease and the Antihypertensive Effects of Hydrolysates in 4 Spontaneously Hypertensive Model Rats, J. Food Sci., 2006, 71, C164–C173 CrossRef CAS.
- D. D. Kitts and K. Weiler, Bioactive proteins and peptides from food sources. Applications of bioprocesses used in isolation and recovery, Curr. Pharm. Des., 2003, 9, 1309–1323 CrossRef CAS PubMed.
- R. Kumar, A. Kumar, R. Sharma and A. Baruwa, Pharmacological review on natural ACE inhibitors, Pharm. Lett., 2010, 2, 286 Search PubMed.
- J. R. Chen, T. Okada, K. Muramoto, K. Suetsuna and S. C. Yang, Identification of angiotensin I-converting enzyme inhibitory peptides derived from the peptic digest of soybean protein, J. Food Biochem., 2002, 26, 543–554 CrossRef.
- J. L. Zhuo, F. M. Ferrao, Y. Zheng and X. C. Li, New frontiers in the intrarenal Renin-Angiotensin system: a critical review of classical and new paradigms, Front. Endocrinol., 2013, 4, 166 Search PubMed.
- M. Bader and D. Ganten, Update on tissue renin-angiotensin systems, J. Mol. Med., 2008, 86, 615–621 CrossRef CAS PubMed.
- T. Hirota, A. Nonaka, A. Matsushita, N. Uchida, K. Ohki, M. Asakura and M. Kitakaze, Milk casein-derived tripeptides, VPP and IPP induced NO production in cultured endothelial cells and endothelium-dependent relaxation of isolated aortic rings, Heart Vessels, 2011, 26, 549–556 CrossRef PubMed.
- Y. Nakamura, N. Yamamoto, K. Sakai and T. Takano, Antihypertensive Effect of Sour Milk and Peptides Isolated from It That Are Inhibitors to Angiotensin I-Converting Enzyme, J. Dairy Sci., 1995, 78, 1253–1257 CrossRef CAS PubMed.
- G. S. Rutella, L. Solieri, S. Martini and D. Tagliazucchi, Release of the Antihypertensive Tripeptides Valine-Proline-Proline and Isoleucine-Proline-Proline from Bovine Milk Caseins during in Vitro Gastrointestinal Digestion, J. Agric. Food Chem., 2016, 64, 8509–8515 CrossRef CAS PubMed.
- K. Majumder and J. Wu, Purification and characterisation of angiotensin I converting enzyme (ACE) inhibitory peptides derived from enzymatic hydrolysate of ovotransferrin, Food Chem., 2011, 126, 1614–1619 CrossRef CAS PubMed.
- K. Majumder, S. Chakrabarti, J. S. Morton, S. Panahi, S. Kaufman, S. T. Davidge and J. Wu, Egg-derived tri-peptide IRW exerts antihypertensive effects in spontaneously hypertensive rats, PLoS One, 2013, 8, e82829 Search PubMed.
- K. Majumder, S. Chakrabarti, J. S. Morton, S. Panahi, S. Kaufman, S. T. Davidge and J. Wu, Egg-derived ACE-inhibitory peptides IQW and LKP reduce blood pressure in spontaneously hypertensive rats, J. Funct. Foods, 2015, 13, 50–60 CrossRef CAS.
- H. Fujita and M. Yoshikawa, LKPNM: a prodrug-type ACE-inhibitory peptide derived from fish protein, Immunopharmacol., 1999, 44, 123–127 CrossRef CAS PubMed.
- T. Lafarga and M. Hayes, Bioactive protein hydrolysates in the functional food ingredient industry: Overcoming current challenges, Food Rev. Int., 2017, 33, 217–246 CrossRef CAS.
- T. Kawasaki, E. Seki, K. Osajima, M. Yoshida, K. Asada, T. Matsui and Y. Osajima, Antihypertensive effect of Valyl-Tyrosine, a short chain peptide derived from sardine muscle hydrolyzate, on mild hypertensive subjects, J. Hum. Hypertens., 2000, 14, 519–523 CAS.
- K. Majumder, G. X. Liang, Y. H. Chen, L. L. Guan, S. T. Davidge and J. Wu, Egg ovotransferrin-derived ACE inhibitory peptide IRW increases ACE2 but decreases proinflammatory genes expression in mesenteric artery of spontaneously hypertensive rats, Mol. Nutr. Food Res., 2015, 59, 1735–1744 CAS.
- K. Majumder and J. Wu, Molecular targets of antihypertensive peptides: Understanding the mechanisms of action based on the pathophysiology of hypertension, Int. J. Mol. Sci., 2014, 16, 256–283 CrossRef PubMed.
- J. Wu, W. Liao and C. Udenigwe, Revisiting the mechanisms of ACE inhibitory peptides from food proteins, Trends Food Sci. Technol., 2017, 69, 214–219 CrossRef CAS.
- P. G. Kopelman, Obesity as a medical problem, Nature, 2000, 404, 635 CrossRef CAS PubMed.
- Y. Okamoto, S. Kihara, T. Funahashi, Y. Matsuzawa and P. Libby, Adiponectin: a key adipocytokine in metabolic syndrome, Clin. Sci., 2006, 110, 267–278 CrossRef CAS PubMed.
- M. Ahmadian, J. M. Suh, N. Hah, C. Liddle, A. R. Atkins, M. Downes and R. M. Evans, PPAR [gamma] signaling and metabolism: the good, the bad and the future, Nat. Med., 2013, 99, 557–566 CrossRef PubMed.
- K. L. Spalding, E. Arner, P. O. Westermark, S. Bernard, B. A. Buchholz, O. Bergmann, L. Blomqvist, J. Hoffstedt, E. Näslund and T. Britton, Dynamics of fat cell turnover in humans, Nature, 2008, 453, 783–787 CrossRef CAS PubMed.
- H. S. Camp, D. Ren and T. Leff, Adipogenesis and fat-cell function in obesity and diabetes, Trends Mol. Med., 2002, 8, 442–447 CrossRef CAS PubMed.
- E. D. Rosen and B. M. Spiegelman, Molecular regulation of adipogenesis, Annu. Rev. Cell Dev. Biol., 2000, 16, 145–171 CrossRef CAS PubMed.
- P. Tontonoz, E. Hu and B. M. Spiegelman, Regulation of adipocyte gene expression and differentiation by peroxisome proliferator activated receptor γ, Curr. Opin. Genet. Dev., 1995, 5, 571–576 CrossRef CAS PubMed.
- H. Park, U. Ju, J. Park, J. Song, D. Shin, K. Lee, L. Jeong, J. Yu, H. Lee and J. Cho, PPARγ neddylation essential for adipogenesis is a potential target for treating obesity, Cell Death Differ., 2016, 23, 1296–1311 CrossRef CAS PubMed.
- H. M. Wright, C. B. Clish, T. Mikami, S. Hauser, K. Yanagi, R. Hiramatsu, C. N. Serhan and B. M. Spiegelman, A synthetic antagonist for the peroxisome proliferator-activated receptor γ inhibits adipocyte differentiation, J. Biol. Chem., 2000, 275, 1873–1877 CrossRef CAS PubMed.
- T. Yamauchi, J. Kamon, H. Waki, K. Murakami, K. Motojima, K. Komeda, T. Ide, N. Kubota, Y. Terauchi and K. Tobe, The mechanisms by which both heterozygous peroxisome proliferator-activated receptor γ (PPARγ) deficiency and PPARγ agonist improve insulin resistance, J. Biol. Chem., 2001, 276, 41245–41254 CrossRef CAS PubMed.
- T. Leff and J. E. Reed, The antidiabetic PPARγ ligands: an update on compounds in development, Curr. Med. Chem.: Immunol., Endocr. Metab. Agents, 2002, 2, 33–47 CrossRef CAS.
- A. Rajic, J. Dhulia, C. G. Hosking and D. J. Autelitano, A novel dairy-derived isolate that inhibits adipogenesis and significantly reduces weight gain in a high fat animal model, Int. Dairy J., 2010, 20, 480–486 CrossRef CAS.
- C. Martinez-Villaluenga, V. P. Dia, M. Berhow, N. A. Bringe and E. Gonzalez de Mejia, Protein hydrolysates from β-conglycinin enriched soybean genotypes inhibit lipid accumulation and inflammation in vitro, Mol. Nutr. Food Res., 2009, 53, 1007–1018 CAS.
- S. Xu, X. Mao, F. Ren and H. Che, Attenuating effect of casein glycomacropeptide on proliferation, differentiation, and lipid accumulation of in vitro Sprague-Dawley rat preadipocytes, J. Dairy Sci., 2011, 94, 676–683 CrossRef CAS PubMed.
- Y. M. Kim, I. H. Kim, J. W. Choi, M. K. Lee and T. J. Nam, The anti-obesity effects of a tuna peptide on 3T3-L1 adipocytes are mediated by the inhibition of the expression of lipogenic and adipogenic genes and by the activation of the Wnt/β-catenin signaling pathway, Int. J. Mol. Med., 2015, 36, 327–334 CrossRef CAS PubMed.
- Y. Arita, S. Kihara, N. Ouchi, M. Takahashi, K. Maeda, J. Miyagawa, K. Hotta, I. Shimomura, T. Nakamura, K. Miyaoka, H. Kuriyama, M. Nishida, S. Yamashita, K. Okubo, K. Matsubara, M. Muraguchi, Y. Ohmoto, T. Funahashi and Y. Matsuzawa, Paradoxical decrease of an adipose-specific protein, adiponectin, in obesity, Biochem. Biophys. Res. Commun., 1999, 257, 79–83 CrossRef CAS PubMed.
- M. Ryo, T. Nakamura, S. Kihara, M. Kumada, S. Shibazaki, M. Takahashi, M. Nagai, Y. Matsuzawa and T. Funahashi, Adiponectin as a biomarker of the metabolic syndrome, Circ. J., 2004, 68, 975–981 CrossRef CAS PubMed.
- A. Nagasawa, K. Fukui, M. Kojima, K. Kishida, N. Maeda, H. Nagaretani, T. Hibuse, H. Nishizawa, S. Kihara and M. Waki, Divergent effects of soy protein diet on the expression of adipocytokines, Biochem. Biophys. Res. Commun., 2003, 311, 909–914 CrossRef CAS PubMed.
- E. Jang, J. Moon, J. Ko, C. Ahn, H. Lee, J. Shin, C. Park and J. Kang, Novel black soy peptides with antiobesity effects: activation of leptin-like signaling and AMP-activated protein kinase, Int. J. Obes., 2008, 32, 1161 CrossRef CAS PubMed.
- T. Nishi, H. Hara, K. Asano and F. Tomita, The soybean β-conglycinin β 51–63 fragment suppresses appetite by stimulating cholecystokinin release in rats, J. Nutr., 2003, 133, 2537–2542 CAS.
- K. Erdmann, B. W. Cheung and H. Schröder, The possible roles of food-derived bioactive peptides in reducing the risk of cardiovascular disease, J. Nutr. Biochem., 2008, 19, 643–654 CrossRef CAS PubMed.
- N. L.-R. Pedersen, C. Nagain-Domaine, S. Mahé, J. Chariot, C. Rozé and D. Tomé, Caseinomacropeptide specifically stimulates exocrine pancreatic secretion in the anesthetized rat, Peptides, 2000, 21, 1527–1535 CrossRef CAS.
- W. Hall, D. Millward, S. Long and L. Morgan, Casein and whey exert different effects on plasma amino acid profiles, gastrointestinal hormone secretion and appetite, Br. J. Nutr., 2003, 89, 239–248 CrossRef CAS PubMed.
- L. Pichon, M. Potier, D. Tome, T. Mikogami, B. Laplaize, C. Martin-Rouas and G. Fromentin, High-protein diets containing different milk protein fractions differently influence energy intake and adiposity in the rat, Br. J. Nutr., 2008, 99, 739–748 CrossRef CAS PubMed.
- L. Guariguata, D. R. Whiting, I. Hambleton, J. Beagley, U. Linnenkamp and J. E. Shaw, Global estimates of diabetes prevalence for 2013 and projections for 2035, Diabetes Res. Clin. Pract., 2014, 103, 137–149 CrossRef CAS PubMed.
-
World Health Organization, Global report on diabetes, 2016, http://www.who.int/mediacentre/factsheets/fs312/en/ (accessed on July 25, 2017).
- K. A. Coughlan, R. J. Valentine, N. B. Ruderman and A. K. Saha, AMPK activation: a therapeutic target for type 2 diabetes?, Diabetes, Metab. Syndr. Obes.: Targets Ther., 2014, 7, 241–253 Search PubMed.
- R. A. DeFronzo, Pathogenesis of type 2 diabetes mellitus, Med. Clin. North Am., 2004, 88, 787–835 CrossRef CAS PubMed.
-
M. F. Kalin, M. Goncalves, J. John-Kalarickal and V. Fonseca, Pathogenesis of type 2 diabetes mellitus, in Principles of diabetes mellitus, ed. L. Poretsky, Springer international publishing, 2017, pp. 267–277 Search PubMed.
- J. L. Leahy, Pathogenesis of type 2 diabetes mellitus, Arch. Med. Res., 2005, 36, 197–209 CrossRef CAS PubMed.
- J. Lu, G. Xie, W. Jia and W. Jia, Insulin resistance and the metabolism of branched-chain amino acids, Front. Med., 2013, 7, 53–59 CrossRef PubMed.
- K. D. Copps and M. F. White, Regulation of insulin sensitivity by serine/threonine phosphorylation of insulin receptor substrate proteins IRS1 and IRS2, Diabetologia, 2012, 55, 2565–2582 CrossRef CAS PubMed.
- R. W. Mackenzie and B. T. Elliott, Akt/PKB activation and insulin signaling: a novel insulin signaling pathway in the treatment of type 2 diabetes, Diabetes, Metab. Syndr. Obes.: Targets Ther., 2014, 7, 55–64 CrossRef CAS PubMed.
- T. F. Franke, D. R. Kaplan, L. C. Cantley and A. Toker, Direct regulation of the Akt proto-oncogene product by phosphatidylinositol-3,4-bisphosphate, Science, 1997, 275, 665–668 CrossRef CAS PubMed.
- K. Ozaki, M. Awazu, M. Tamiya, Y. Iwasaki, A. Harada, S. Kugisaki, S. Tanimura and M. Kohno, Targeting the ERK signaling pathway as a potential treatment for insulin resistance and type 2 diabetes, Am. J. Physiol.: Endocrinol. Metab., 2016, 310, E643–E651 Search PubMed.
- K. R. Wick, E. D. Werner, P. Langlais, F. J. Ramos, L. Q. Dong, S. E. Shoelson and F. Liu, Grb10 inhibits insulin-stimulated insulin receptor substrate (IRS)-phosphatidylinositol 3-kinase/Akt signaling pathway by disrupting the association of IRS-1/IRS-2 with the insulin receptor, J. Biol. Chem., 2003, 278, 8460–8467 CrossRef CAS PubMed.
- P. P. Hsu, S. A. Kang, J. Rameseder, Y. Zhang, K. A. Ottina, D. Lim, T. R. Peterson, Y. M. Choi, N. S. Gray, M. B. Yaffe, J. A. Marto and D. M. Sabatini, The mTOR-Regulated Phosphoproteome Reveals a Mechanism of mTORC1-Mediated Inhibition of Growth Factor Signaling, Science, 2011, 332, 1317–1322 CrossRef CAS PubMed.
- F. Das, N. Ghosh-Choudhury, N. Dey, C. C. Mandal, L. Mahimainathan, B. S. Kasinath, H. E. Abboud and G. G. Choudhury, Unrestrained Mammalian Target of Rapamycin Complexes 1 and 2 Increase Expression of Phosphatase and Tensin Homolog Deleted on Chromosome 10 to Regulate Phosphorylation of Akt Kinase, J. Biol. Chem., 2012, 287, 3808–3822 CrossRef CAS PubMed.
- M. F. Bachok, B. N. M. Yusof, A. Ismail and A. A. Hamid, Effectiveness of traditional Malaysian vegetables (ulam) in modulating blood glucose levels, Asia Pac. J. Clin. Nutr., 2014, 23, 369–376 Search PubMed.
- J. Singh, E. Cumming, G. Manoharan, H. Kalasz and E. Adeghate, Medicinal chemistry of the anti-diabetic effects of momordica charantia: active constituents and modes of actions, Open Med. Chem. J., 2011, 5, 70–77 CrossRef CAS PubMed.
- S. J. Yang, J. M. Choi, S. E. Park, E. J. Rhee, W. Y. Lee, K. W. Oh, S. W. Park and C. Y. Park, Preventive effects of bitter melon (Momordica charantia) against insulin resistance and diabetes are associated with the inhibition of NF-kappaB and JNK pathways in high-fat-fed OLETF rats, J. Nutr. Biochem., 2015, 26, 234–240 CrossRef CAS PubMed.
- J. Bai, Y. Zhu and Y. Dong, Response of gut microbiota and inflammatory status to bitter melon (Momordica charantia L.) in high fat diet induced obese rats, J. Ethnopharmacol., 2016, 194, 717–726 CrossRef PubMed.
- B. Jiang, M. Ji, W. Liu, L. Chen, Z. Cai, Y. Zhao and X. Bi, Antidiabetic activities of a cucurbitanetype triterpenoid compound from Momordica charantia in alloxaninduced diabetic mice, Mol. Med. Rep., 2016, 14, 4865–4872 CrossRef CAS PubMed.
- M. J. Tan, J. M. Ye, N. Turner, C. Hohnen-Behrens, C. Q. Ke, C. P. Tang, T. Chen, H. C. Weiss, E. R. Gesing, A. Rowland, D. E. James and Y. Ye, Antidiabetic activities of triterpenoids isolated from bitter melon associated with activation of the AMPK pathway, Chem. Biol., 2008, 15, 263–273 CrossRef CAS PubMed.
- H. Y. Lo, C. C. Li, T. Y. Ho and C. Y. Hsiang, Identification of the bioactive and consensus peptide motif from Momordica charantia insulin receptor-binding protein, Food Chem., 2016, 204, 298–305 CrossRef CAS PubMed.
- H. Y. Lo, T. Y. Ho, C. C. Li, J. C. Chen, J. J. Liu and C. Y. Hsiang, A novel insulin receptor-binding protein from Momordica charantia enhances glucose uptake and glucose clearance in vitro and in vivo through triggering insulin receptor signaling pathway, J. Agric. Food Chem., 2014, 62, 8952–8961 CrossRef CAS PubMed.
- H. Y. Lo, T. Y. Ho, C. Lin, C. C. Li and C. Y. Hsiang, Momordica charantia and its novel polypeptide regulate glucose homeostasis in mice via binding to insulin receptor, J. Agric. Food Chem., 2013, 61, 2461–2468 CrossRef CAS PubMed.
- J. Lu, Y. Zeng, W. Hou, S. Zhang, L. Li, X. Luo, W. Xi, Z. Chen and M. Xiang, The soybean peptide aglycin regulates glucose homeostasis in type 2 diabetic mice via IR/IRS1 pathway, J. Nutr. Biochem., 2012, 23, 1449–1457 CrossRef CAS PubMed.
- M. Eddouks, A. Bidi, B. El Bouhali, L. Hajji and N. A. Zeggwagh, Antidiabetic plants improving insulin sensitivity, J. Pharm. Pharmacol., 2014, 66, 1197–1214 CrossRef CAS PubMed.
- N. B. Ruderman, D. Carling, M. Prentki and J. M. Cacicedo, AMPK, insulin resistance, and the metabolic syndrome, J. Clin. Invest., 2013, 123, 2764–2772 CrossRef CAS PubMed.
- M. J. Sanders, P. O. Grondin, B. D. Hegarty, M. A. Snowden and D. Carling, Investigating the mechanism for AMP activation of the AMP-activated protein kinase cascade, Biochem. J., 2007, 403, 139–148 CrossRef CAS PubMed.
- M. C. Towler and D. G. Hardie, AMP-activated protein kinase in metabolic control and insulin signaling, Circ. Res., 2007, 100, 328–341 CrossRef CAS PubMed.
- K. A. Coughlan, R. J. Valentine, N. B. Ruderman and A. K. Saha, Nutrient Excess in AMPK Downregulation and Insulin Resistance, J. Endocrinol. Diabetes Obes., 2013, 1, 1008 Search PubMed.
- H. Sano, S. Kane, E. Sano, C. P. Miinea, J. M. Asara, W. S. Lane, C. W. Garner and G. E. Lienhard, Insulin-stimulated phosphorylation of a Rab GTPase-activating protein regulates GLUT4 translocation, J. Boil. Chem., 2003, 278, 14599–14602 CrossRef CAS PubMed.
- G. Ramm, M. Larance, M. Guilhaus and D. E. James, A role for 14–3–3 in insulin-stimulated GLUT4 translocation through its interaction with the RabGAP AS160, J. Boil. Chem., 2006, 281, 29174–29180 CrossRef CAS PubMed.
- K. Sakamoto and G. D. Holman, Emerging role for AS160/TBC1D4 and TBC1D1 in the regulation of GLUT4 traffic, Am. J. Physiol.: Endocrinol. Metab., 2008, 295, E29–E37 CrossRef CAS PubMed.
- D. Carling, AMPK signalling in health and disease, Curr. Opin. Cell Biol., 2017, 45, 31–37 CrossRef CAS PubMed.
- S. Chen, D. H. Wasserman, C. MacKintosh and K. Sakamoto, Mice with AS160/TBC1D4-Thr649Ala knockin mutation are glucose intolerant with reduced insulin sensitivity and altered GLUT4 trafficking, Cell Metab., 2011, 13, 68–79 CrossRef CAS PubMed.
- J. J. Song, Q. Wang, M. Du, T. G. Li, B. Chen and X. Y. Mao, Casein glycomacropeptide-derived peptide IPPKKNQDKTE ameliorates high glucose-induced
insulin resistance in HepG2 cells via activation of AMPK signaling, Mol. Nutr. Food Res., 2017, 61, 1600301 Search PubMed.
- C. Roblet, A. Doyen, J. Amiot, G. Pilon, A. Marette and L. Bazinet, Enhancement of glucose uptake in muscular cell by soybean charged peptides isolated by electrodialysis with ultrafiltration membranes (EDUF): activation of the AMPK pathway, Food Chem., 2014, 147, 124–130 CrossRef CAS PubMed.
- M. Soga, A. Ohashi, M. Taniguchi, T. Matsui and T. Tsuda, The di-peptide Trp-His activates AMP-activated protein kinase and enhances glucose uptake independently of insulin in L6 myotubes, FEBS Open Bio, 2014, 4, 898–904 CrossRef CAS PubMed.
- W. J. Yeh, H. Y. Yang and J. R. Chen, Soy beta-conglycinin retards progression of diabetic nephropathy via modulating the insulin sensitivity and angiotensin-converting enzyme activity in rats fed with high salt diet, Food Funct., 2014, 5, 2898–2904 CAS.
- N. Tachibana, Y. Yamashita, M. Nagata, S. Wanezaki, H. Ashida, F. Horio and M. Kohno, Soy beta-conglycinin improves glucose uptake in skeletal muscle and ameliorates hepatic insulin resistance in Goto-Kakizaki rats, Nutr. Res., 2014, 34, 160–167 CrossRef CAS PubMed.
- A. Avignon, A. Radauceanu and L. Monnier, Nonfasting plasma glucose is a better marker of diabetic control than fasting plasma glucose in type 2 diabetes, Diabetes Care, 1997, 20, 1822–1826 CrossRef CAS PubMed.
- P. Patil, S. Mandal, S. K. Tomar and S. Anand, Food protein-derived bioactive peptides in management of type 2 diabetes, Eur. J. Nutr., 2015, 54, 863–880 CrossRef CAS PubMed.
- J. Caron, B. Cudennec, D. Domenger, Y. Belguesmia, C. Flahaut, M. Kouach, J. Lesage, J. F. Goossens, P. Dhulster and R. Ravallec, Simulated GI digestion of dietary protein: Release of new bioactive peptides involved in gut hormone secretion, Food Res. Int., 2016, 89, 382–390 CrossRef CAS PubMed.
- A. Zambrowicz, M. Pokora, B. Setner, A. Dabrowska, M. Szoltysik, K. Babij, Z. Szewczuk, T. Trziszka, G. Lubec and J. Chrzanowska, Multifunctional peptides derived from an egg yolk protein hydrolysate: isolation and characterization, Amino Acids, 2015, 47, 369–380 CrossRef CAS PubMed.
- B. Konrad, D. Anna, S. Marek, P. Marta, Z. Aleksandra and C. Jozefa, The Evaluation of Dipeptidyl Peptidase (DPP)-IV, alpha-Glucosidase and Angiotensin Converting Enzyme (ACE) Inhibitory Activities of Whey Proteins Hydrolyzed with Serine Protease Isolated from Asian Pumpkin (Cucurbita ficifolia), Int. J. Pept. Res. Ther., 2014, 20, 483–491 CrossRef CAS PubMed.
- S. L. Huang, C. L. Jao, K. P. Ho and K. C. Hsu, Dipeptidyl-peptidase IV inhibitory activity of peptides derived from tuna cooking juice hydrolysates, Peptides, 2012, 35, 114–121 CrossRef CAS PubMed.
|
This journal is © The Royal Society of Chemistry 2018 |