A review of sanitation technologies to achieve multiple sustainable development goals that promote resource recovery
Received
17th June 2017
, Accepted 2nd November 2017
First published on 3rd November 2017
Abstract
In 2015 the global community approved the seventeen sustainable development goals (SDGs). The SDGs include a goal regarding access to clean water and sanitation with specific targets such as providing access to adequate and equitable sanitation for all, improving water quality by reducing pollution, halving the proportion of untreated wastewater, and increasing recycling and safe reuse globally. There are opportunities to promote sanitation technologies that would achieve these sanitation targets and minimize health risk from exposure to pathogens while also achieving SDGs related to increased food security and sustainable management of waste and natural resources through the recovery of beneficial resources such as energy and fertilizer. To that end, the objective of this paper is to critically review literature that is supported by material flow diagrams developed for nitrogen, phosphorus, and carbon, to determine the ability of existing sanitation technologies and strategies that are deployed in a centralized or decentralized manner to safely recover resources and thus achieve multiple sustainable development goals. The one strategy and six technologies are 1) dig and cover, 2) bucket latrine, 3) ventilated improved pit (VIP) latrine, 4) double-vault composting latrine, 5) urine-diverting composting latrine, 6) pour-flush toilet connected to a septic tank, and 7) sewered toilet. Results showed the six sanitation technologies and one strategy could be grouped into three categories based on their potential to recover resources without further processes added to provide resource recovery. Group 1 (unimproved, no resource recovery), which included the baseline strategy of dig and cover and the unimproved technology of bucket latrines, demonstrated no ability to achieve resource recovery from human waste without further resource recovery treatment. Container based sanitation (not discussed here) may, however, be an appropriate method to safely dispose of excreta while also providing opportunities for resource recovery. Group 2 (improved, no resource recovery), which included the technologies ventilated improved pit (VIP) latrine, pour-flush toilet with septic tank, and sewered toilet, were also shown to have no ability to recover resources without further resource recovery treatment. Group 3 (improved, resource recovery), which included double-vault composting latrine and urine-diverting compositing latrine), demonstrated the greatest ability to recover resources from human waste. However, policies to ensure safe handling of resources generated at onsite locations is needed and resource recovery processes can be integrated with treatment processes to recover embedded resources from bucket latrines, VIP latrines, pour-flush toilets with septic tanks, and sewered toilets. The results provide guidance to achieve multiple sustainable development goals through the implementation of sanitation technologies that have potential to recover beneficial resources.
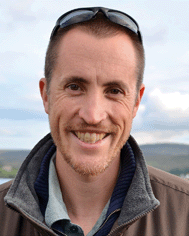 Kevin Orner | Kevin Orner is a Ph.D. Candidate in Environmental Engineering at the University of South Florida, where he studies nutrient and energy recovery from wastewater. After obtaining a B.S. in Civil & Environmental Engineering from the University of Wisconsin-Madison in 2008, he served for two years as a Peace Corps Volunteer in Panama. In 2011, he completed his M.S. in Civil & Environmental Engineering at the University of South Florida. He also is a Fulbright Research Grant recipient. |
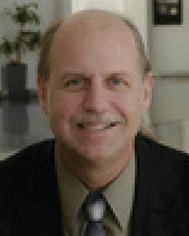 James R. Mihelcic | Dr. James R. Mihelcic is the Samuel L. and Julia M. Flom Professor of Civil and Environmental Engineering at the University of South Florida where he also directs the International Development Engineering Program. He is the author of several engineering books and an international expert in provision of water, sanitation, and hygiene in developed and developing world communities. His teaching and research interests are centered around engineering and sustainability, including understanding how global stressors influence selection and provision of sanitation technologies that can be integrated with nutrient management and resource recovery. |
Water impact
Multiple sustainable development goals and targets related to food security and sustainable management of water, human waste, and natural resources can be integrated with sanitation targets by promoting the safe recovery of resources from urine and feces. To that end, this study reviews literature and compares material flows of nitrogen, phosphorus, and organic carbon for six sanitation technologies and one strategy.
|
1 Introduction
Safe disposal of human excreta has traditionally had the dual goals of protecting human health and the environment. Although 2.1 billion people received improved sanitation from 1990–2015, 2.4 billion still lack access.1 Another 1.5 billion have access to sanitation facilities that do not treat the excreta before discharge into the environment.2 Furthermore, an estimated 280
000 diarrheal deaths still occur annually from inadequate sanitation3 with rates of diarrheal disease estimated to be reduced by approximately 35% through improved sanitation.4,5 Sanitation technologies provide a barrier between humans and harmful pathogens to improve public health, but other factors should be considered. In the past few years a discussion has emerged regarding what makes a sanitation treatment technology more environmentally sustainable; however, this discussion has primarily been applied to larger unit processes and centralized systems that require a sewer connection.6–8 Research has also documented sustainability concerns associated with emissions of greenhouse gases from domestic wastewater treatment.9,10
In 2015 the global community approved the seventeen sustainable development goals (SDGs) and 169 targets that will drive development for the next fifteen years and beyond. The SDGs replace the expired millennium development goals (MDGs). The MDG sanitation targets were “the ones most lagging behind”.11 The SDGs are more ambitious; for example, SDG 6 is to “ensure availability and sustainable management of water and sanitation for all.” Under this goal, target 6.2 aims by 2030 to achieve access to adequate and equitable sanitation for all and target 6.3 seeks to improve water quality by reducing pollution, halving the proportion of untreated wastewater, and increasing recycling and safe reuse globally by 2030.12 These two specific targets thus have the potential to lead to the construction of a large number of new onsite and large centralized wastewater treatment plants around the world. One challenge in meeting these targets is that future planning for sanitation provision will have to not only consider the 2.4 billion people currently without improved sanitation, but also an increasingly affluent global population that will increase to over 9 billion by 2050.13 Because of the large number of the global population still not served by improved sanitation, there are also opportunities to promote new resource recovery goals alongside traditional waste management goals7,14,15 while also evaluating the environmental sustainability of such integrated systems in a developing world setting.16 However, up to this time, research of household sanitation technologies that have resource recovery as an implementation objective has been primarily focused on identifying exposure routes and associated health risks,17,18 demonstrating how ecological sanitation technologies support food security,19 identifying cultural factors that lead to adoption of ecological sanitation technologies,20–22 and identifying mechanisms of pathogen destruction or enhanced treatment of pathogens in such systems.23–25
SDG targets 6.2 and 6.3 should also be integrated with other SDG targets related to food security and sustainable management of natural resources.26 For example, target 2.4 aims to improve food security through increased productivity and production from resilient agriculture practices that help maintain ecosystems, target 12.2 seeks to achieve the sustainable management and efficient use of natural resources, and target 12.5 seeks to reduce waste generation through prevention, reduction, recycling and reuse. Thus, the global community is at a critical point where it should consider how to best achieve SDG targets 6.2 and 6.3 related to sanitation provision and promotion of wastewater treatment/reuse for the 2.4 billion people without access to improved sanitation. Important targets related to increasing food security and more sustainable management of natural resources and wastes can also be achieved alongside SDG targets 6.2 and 6.3. This paper thus differs from existing reviews on sanitation technologies that highlight its connection to reducing diarrhea,27,28 limit helminth infections,29,30 address implementation in urban areas,31,32 manage fecal sludge,33 and improve human rights,34 but do not focus on how resource recovery can achieve multiple sustainable development goals. A recent paper35 highlights the ability of resource recovery to achieve multiple sustainable development goals, but does not review or recommend specific sanitation technologies.
Accordingly, the objective of this paper is to critically review literature to determine the ability of existing sanitation technologies and strategies that are deployed in a centralized or decentralized manner to safely recover resources and thus achieve multiple sustainable development goals. In addition, the influent and effluent material flows of nitrogen, phosphorus, organic carbon (referred to hereafter as carbon), and greenhouse gas emissions were estimated and resource recovery potential of the technologies and strategy were assessed. This information can provide guidance on the implementation of improved sanitation technologies deployed in a centralized or decentralized manner that recover resources and therefore achieve multiple SDGs related to providing access to improved sanitation, increased food security, and sustainable management of water, human waste, and natural resources.
Although newer sanitation technologies are being demonstrated,36–38 this manuscript will evaluate seven proven sanitation strategies and technologies:39,40 one unimproved strategy (dig and cover) and six technologies (1. bucket latrines, 2. ventilated improved pit (VIP) latrine, 3. double-vault composting latrine, 4. urine-diverting compositing latrine, 5. pour-flush toilet with septic tank, and 6. sewered toilet). Our hope is that the 2.4 billion people currently without access and 2 billion who will be added to our planet by 2050 will have access to sanitation technologies that protect human health and the environment. At the same time, broader goals of community well-being and environmental sustainability that benefit future generations through recovery of valuable resources from materials that historically have been considered a waste can also be achieved.
This study's outcomes are important because the number of people who use a household sanitation technology is expected to rise from 2.7 billion to 5 billion by 2030,41 and the sustainable development goals require access to sanitation for all. Implementation of decentralized household-managed technologies will also require different methods to ensure end user compliance, which is not often required for centralized technologies that employ sewers. Several decentralized technologies are highlighted in this study because even in urban contexts not everyone will gain access to a sewer due to geography, presence of informal settlements, and limited resources. Additionally, there may not be sufficient water to operate conventional centralized sanitation technologies that convey waste in sewers from flush toilets, particularly for 46 million people without improved sanitation who live in areas of water scarcity.42 For example, 38% of the 800 million people in Sub-Saharan Africa already live in water scarce areas, yet a 283% increase in water demand is expected between 2005 and 2030.43,44
2 Background of sanitation technologies and strategies
The seven sanitation technologies and strategies examined in this study (Table 1) incorporate some or all of the five functional groups defined by Tilley et al.:39 1) user interface, 2) collection and storage/treatment, 3) conveyance, 4) semi-centralized treatment, and 5) use and/or disposal. Five technologies are considered improved, meaning they separate humans from contacting excreta. Based on how the improved facilities are used, which can be evaluated under the monitoring framework to determine progress towards meeting the SDGs, they can be assigned service levels of safely managed, basic, or limited.45 A safely managed facility, which allows for treatment and disposal to occur onsite, collected and managed off-site, or transported off-site by a sewer, is a good candidate for resource recovery. For example, the safe collection of urine could allow for recovery and reuse of nutrients such as nitrogen, phosphorus, and potassium. Technologies that incorporate stored feces, such as latrines and septic tanks, can incorporate fecal sludge management, defined as the effective collection, transport, treatment and/or disposal of fecal sludge that has not traveled through a sewer.41 Fecal sludge management can be cost-effective sanitation solution that provides potential beneficial reuse of waste solids as a soil amendment or for energy production.46 For example, fecal sludge not only can be composted in a latrine, but may also be collected from latrines, transported, and treated to recover resources.
Table 1 The six sanitation technologies and one strategy studied
Name of sanitation technology or strategy |
Strategy/technology |
Centralized/decentralized |
Improved/unimproved |
Can be clustered together to create a hybrid approach that incorporates decentralized and centralized management.
These technologies can meet criteria set by the WHO/UNICEF Joint Monitoring Programme for water supply, sanitation and hygiene to monitor progress towards the SDGs for being a safely managed service provided to a household because they can be implemented so they are not shared by households and the excreta can also be: 1) treated/disposed of in situ, 2) stored temporarily and then emptied, transported, and treated off-site, 3) or transported through a sewer and then treated off-site. These technologies can also meet basic service levels that deploy the technology so it is not shared by multiple households (but ignores excreta management) and limited sanitation service levels that include use of improved technology that is shared by multiple households (but ignores excreta management).
|
1. Dig & Cover |
Strategy |
Decentralized |
Unimproved |
2. Bucket latrine |
Technology |
Decentralized |
Unimproved |
3. Ventilated improved pit (VIP) latrine |
Technology |
Decentralized |
Improvedb |
4. Double-vault composting latrine |
Technology |
Decentralized |
Improvedb |
5. Urine-diverting composting latrine |
Technology |
Decentralized |
Improvedb |
6. Pour-flush toilet with septic tank |
Technology |
Decentralizeda |
Improvedb |
7. Sewered toilet |
Technology |
Centralized |
Improvedb |
The strategy of dig and cover is a slight upgrade over open defecation; the user interface and collection is the ground and feces are covered with a small amount of soil or rolled onto dirt or sand. A bucket latrine, an unimproved technology, collects feces (urine is not commonly collected) and can be daily conveyed to a separate location, such as a composting pile.47 Container-based systems,48 which are similar to bucket latrines because they both utilize manual collection, are not considered in this study due to their recent emergence as a sanitation technology. Container-based systems may be a more hygienic alternative over bucket latrines because the excreta is stored, transported, and disposed of in sealable containers by hired workers and also more amenable to resource recovery at a centralized location.
A ventilated improved pit (VIP) latrine, an improved technology, is similar to the traditional pit latrine in that it has a pit and slab, but also promotes air flow into the pit and up a mesh-covered ventilation pipe to reduce complains of odors and insects.49 The latrines include the user interface of a toilet, collection in a pit, and possible conveyance and treatment through fecal sludge management.39 A double-vault composting latrine requires addition of sufficient dry organic material after each use, and the resulting pile must be mixed to provide sufficient aeration to promote composting.23,50 A urine-diverting composting latrine can assist the composting process and/or pathogen destruction by increasing the carbon-to-nitrogen ratio and assisting desiccation while also allowing for urine collection and subsequent reuse of valuable nutrients that can be used in small-scale farming51–53 while also reducing odors and insects. Composting latrines incorporate a user interface of a dry toilet and collection in a dehydration vault. The feces can be conveyed for further treatment, application or disposal while the urine can be land-applied.39 The pour-flush toilet with septic tank flushes solid and liquid waste using water into a below-ground septic tank or subsurface soak away pit.54 Odors and flies are reduced through a water seal trap. The toilet is the user interface, the septic tank is the collection, and conveyance and treatment is through either buffering of pollutants by the surrounding soil or fecal sludge management when sludge in the tank is emptied.39 However, the liquid effluent from septic tanks is sometimes released without further treatment to groundwater or surface water. Sewered toilets utilize toilets as the user-interface and conveyance through a simplified or gravity sewer.39 Many scenarios exist for the treatment of sewer effluent:55 it could be discharged without treatment, treated for pathogen removal, treated for carbon removal (i.e. measured as biochemical oxygen demand), treated for nutrient removal, treated for resource recovery, and, after possible treatment, disposed to land or water. The scenario provided by Gray & Becker, which models nutrient flows through an urban water system near Perth, Australia, is used in the Sankey diagrams.56
The seven sanitation technologies and strategies vary in number and location. Fig. 1 shows the percentage of the population served by the majority of the above technologies. North America and Australia are dominated by sewered systems with the remaining population mostly served by septic. Europe, South America, and North Africa still have the majority of users on sewer, but also utilize pit latrines in addition to septic. No single technology accounts for the majority in North Asia, South Asia, or sub-Saharan Africa. The leading technologies are sewer and pit latrines in North Asia, septic in South Asia, and pit latrines in sub-Saharan Africa.57
 |
| Fig. 1 Share of population served by different sanitation technologies by region, 2014 (reproduced with permission of the Boston Consulting Group 2014). | |
Treatment of wastewater, defined as pathogen inactivation, organic removal, nutrient removal, and micro-pollutant removal, is uncommon worldwide.32 Even conveyance through a sewer does not result in treatment in most developing world settings, hence the development of SDG target 6.3. In fact, it is estimated that 80–90% of wastewater goes untreated.58,59 Even in high-income countries, 30% of wastewater is untreated, receiving no primary or secondary treatment. The percentage rises to 92% for low-income countries.60 In cities, especially those with large slum populations, fewer than 35% in developing countries have any form of wastewater treatment;2 in sub-Saharan Africa the percentage drops to 2%.61 Most of the wastewater treatment that does exist is primary and is thus limited to solids removal (some of which are organic).
Access to onsite sanitation technologies in cities incorporate additional challenges. For example, an estimated 30 people use each latrine in Kampala;62 only 15% of households used available latrines in Cambodia;63 and 50–60% of onsite sanitation technologies were unusable in Jakarta.64
3 Methods
To determine the ability of specific sanitation technologies to provide recovery of resources embedded in human excrement, the nitrogen, phosphorus, and carbon material flows for the seven sanitation technologies and strategies were visually represented by a Sankey diagram. Flow diagrams are used to communicate the transfer of fluid to and from multiple destinations. One type of flow diagram is the excreta flow diagram (often referred to as a shit flow diagram). This diagram is used to readily understand and visually communicate how excreta physically flows through a city or town.65 Sankey diagrams, another type of flow diagram, also utilize different widths of arrows to visually represent varying quantities of material. Sankey diagrams have been typically used in energy and natural resource applications.66 The Sankey diagrams used in this study differ from excreta flow diagrams because nutrient flows are tracked from urine and feces through a sanitation technology and not through an entire city or town. Though it does not use Sankey diagrams, the mapping tool REVAMP evaluates the potential for recovery of nutrients from urban waste streams.67
The Sankey diagram software used in this study, SankeyMATIC, is available online.68 Three separate Sankey diagrams were produced for each technology: nitrogen, phosphorus, and carbon. Each Sankey diagram (introduced later as Fig. 2) has two columns with an input and an output. The first column has an input of percentage of the nutrient in urine and feces and an output of 100% of the nutrient, which then becomes the input to the second column. The second column has an output of the percentage of the nutrient in each category recovered or unrecovered after entering the sanitation technology. Input quantities were assumed to be the same for all the technologies and strategies examined in this study. The system boundary was assumed to occur immediately around the strategy or technology. For example, we only accounted for biochemical transformations in a latrine pit or septic tank and did not consider any attenuation provided after the contents left the infrastructure and entered the surrounding soil environment.
 |
| Fig. 2 Sankey diagrams for group 1 (as-is, unimproved, no resource recovery) sanitation strategies and technologies (without further resource recovery treatment) that depict nitrogen, phosphorus, and carbon material flows, respectively, as a percentage of input and output. Different color schemes are only used to contrast and differentiate pathways of nitrogen (left), phosphorus (middle), and carbon (right) recovery. | |
3.1 Influent material flow
Influent to a sanitation system can be divided into two primary materials: feces and urine. This study ignored the input of solid or liquid materials used for anal cleansing or menstrual hygiene and greywater discharges because they are regionally dependent and highly variable. The influent values of nitrogen, phosphorus, and carbon from feces and urine were represented in the Sankey diagrams as 100% of the input of these three chemical species for all technologies and strategies.
Humans generate 100–350 g p−1 d−1 of feces,58,69,70 of which the water content is 75% of the mass.69 Factors affecting the mass of fecal matter discharged by a human include their diet, climate, level of urbanization, country's level of income, and age.71–73 Mass generation of fecal nitrogen ranges from 0.8–2.2 g p−1 d−1, or 10–20% of nitrogen in excreta.74,75 The fecal nitrogen consists of approximately 63% organic nitrogen (nucleic acid, proteins, uric acid, and enzymes), 20% ammonia, and 17% organic nitrogen in the cells of bacteria.72 Mass generation of fecal phosphorus ranges from 3.8–5.5 g p−1 d−1, which represents 30% of phosphorus found in excreta,74 and is mostly in the form of calcium phosphate.76 Organic carbon in feces represents approximately 76–79% of carbon in excreta.74,77
Humans produce 0.8–1.5 L p−1 d−1 of urine.71,78 The volume of urine discharge and its chemical characteristics are dependent on physical exertion and environmental conditions as well as water, salt, and protein intake.14,70 80–90% of nitrogen in excreta is found in urine.71,75,79,80 3.8–5.5 g p−1 d−1, or 50–70%, of phosphorus is found in urine.70,74,80 Urine also contains 21–24% of the COD in human excreta.74,79
3.2 Nitrogen pathway and recovery
The effluent nitrogen pathway for the individual Sankey diagrams was determined for each sanitation technology or strategy. For the VIP latrine, the nitrogen in the effluent was determined from a study that measured the amount of residual nitrogen in abandoned latrines, deep leaching of nitrate using a chlorine tracer, and ammonia volatilization in diffusion tubes placed in the pit latrines' ventilation tubes.81 The remaining nitrogen in that study was assumed to be lost due to microbial degradation.39 That study showed that nitrogen loss in the pit occurred as <1% from volatilization of ammonia, 13% from leaching of nitrate, 70% loss from denitrification, and 17% residual nitrogen remaining. The 87% nitrogen removal is greater than one study that only saw 30% nitrogen removal,82 but aligns with the 13% nitrogen lost through leaching aligns with two other previous studies which have seen 1–50% nitrogen loss through leaching,81,83 which can cause nitrate contamination of groundwater.84–88
Dig and cover was assumed to have similar nitrogen effluent values to the pit latrine because it was considered to have similar aerobic and anaerobic zones as expected in a latrine pit. All nitrogen in the feces in the bucket latrine was assumed to remain in the bucket because it is frequently emptied. For the double-vault composting latrine, it was assumed that the urine was not diverted and the nitrogen transformation was similar to what was reported in a study that measured nitrogen in composted poultry manure that was aerated every two weeks and kept in a polyvinyl resin bag. That study determined that nitrogen losses can reach up to 70%,89 and is within the range of 30–94% nitrogen removal found by Fuhrmeister.90 The remaining 30% of the nitrogen was assumed to remain in the compost. The urine diversion system allows for the recovery of 93% of total nitrogen in urine if collected and stored in a sealed container.91 The Sankey diagrams display 88% recovery of urine. In contrast, 70% of the remaining nitrogen present in the fecal matter was assumed to be lost to microbial degradation.69 Septic tanks were assumed to lose 10% of influent nitrogen, which is the average value reported in three studies92–94 and within the range of Fuhrmeister90 due to mineralization and extraction.95 One study measured nitrous oxide emissions from septic tanks to be 0.2 g p−1 d−1 (ref. 96) or 2% of the assumed influent nitrogen. We are not aware of studies measuring nitrous oxide emissions for the other technologies and strategies discussed here. The fecal sludge, present in latrines and septic tanks, typically has 10–100 times the total nitrogen concentration of wastewater.41
For the improved sanitation technology of a flush toilet connected to a sewer, one study of sewer influent and effluent in 8 km of sewer pipe in Hong Kong found no loss of influent nitrogen.97 Another study noted that excreta, which contains 92.2% of household nitrogen effluent, combines with household greywater and rainfall inflow in the sewer. The treatment plant removed 87.7% of the input nitrogen; however, 5% of the input nitrogen was lost due to exfiltration in the sewer and 7.4% remained in the effluent.56
3.3 Phosphorus pathway and recovery
The effluent phosphorus recorded in the Sankey diagrams is also dependent on the particular sanitation technology or strategy. Phosphorus is relatively less mobile and biochemically reactive than nitrogen. Phosphorus in human excreta is commonly found in one of three forms: phosphate, organically bound phosphate, or orthophosphoric acid.41 Phosphate does not travel far into soils98 and therefore is assumed not to cause groundwater contamination. In dig and cover, all phosphorus was assumed to remain in the soil. Recovery of phosphorus was not considered because of the large number of distributed disposal sites associated with this strategy. The feces from bucket latrines can be recovered through fecal sludge management. This study assumed 18% of phosphorus was removed in pit latrines based on the average value of the range reported by Nelson and Murray.82 Double-vault composting latrines retained all the phosphorus in the resulting composted solids99 and urine diversion was assumed to collect 70% of influent phosphorus.77 Phosphorus intake by plants from urine fertilizer can exceed that of mineral fertilizers.100 One study of septic tanks reported 75% phosphorus removal,101 whereas another only reported 35%;90 therefore, this study assumed 55% of the influent phosphorus was removed. Fecal sludge, which contains 2–50 times the concentration of total phosphorus as domestic wastewater, can be recovered from both latrines and septic tanks.41 For the sewered toilet scenario, one study concluded that excreta contains 87.5% of household phosphorus effluent and combines with household greywater and rainfall inflow in the sewer. The treatment plant removes 91.5% of the input phosphorus; however, 5.0% of the input phosphorus was lost due to exfiltration in the sewer and 3.5% remained in the effluent.56
3.4 Carbon pathway and recovery
The amount of carbon that is transformed or remains in a particular technology or strategy is dependent on the systems' redox conditions. For all effluent pathways for dig and cover and a VIP latrine pit, the environment was considered to be 50% anaerobic and 50% aerobic.102 Latrine pits have traditionally been assumed to be totally anaerobic environments; however, some research suggests a portion of the pit contents may first decompose aerobically prior to undergoing anaerobic decomposition deeper in the pit.102 This may be possible because in a VIP latrine airflow is channeled down and across the liquid surface of the pit before exiting out a ventilation pipe. A higher carbon content and resulting anaerobic zone is expected deeper in the pit where solids have settled. Reduction of carbon in the pits through microbial degradation is low, but organic carbon present in the liquids in the pit may enter the surrounding soil.39 In our study, VIP latrines were assumed to retain 35% of influent carbon within the pit;82 the remaining carbon was assumed to be lost either through biochemical reactions of the solids or leaching of liquid from the pit. Because bucket latrines are frequently emptied, all carbon is assumed to remain in the bucket.
3.5 Greenhouse gas emissions
Greenhouse gas emissions were analyzed only for those generated from carbon sources and not nitrogen. Nitrous oxide emissions, common during denitrification, are well studied in municipal wastewater treatment plants.103,104 However, nitrous oxide emissions are not included in this study because, with the exception of septic tanks,95 very little research has been done on nitrous oxide emissions from decentralized technologies such as pit latrines and composting latrines.95,105 Because nitrous oxide has 298 times the greenhouse gas equivalents of carbon dioxide, more research is needed to quantify nitrous oxide emissions from sanitation technologies. Carbon can be converted into greenhouse gases such as carbon dioxide and methane. Approximately 9% of global methane emissions were estimated to originate from wastewater in 2000.106 From 2010–2030, methane emissions from wastewater may increase by almost 20%.32 The emissions of carbon dioxide and methane for dig and cover, a VIP latrine, a double-vault composting latrine, and a urine-diverting composting latrine were determined using the following two equations:107 | CO2 = BOD × EffOD × CFCO2 × [(1 – MCFww × BGCH4)(1 − λ)] | (1) |
| CH4 = BOD × EffOD × CFCH4 × [(MCFww × BGCH4)(1 − λ)] | (2) |
where CO2 and CH4 are the air emissions (g per capita per day), BOD is the daily mass of biochemical oxygen demand (85 g) in the influent,108 EffOD is the oxygen demand removal efficiency (assumed 80%), CFCO2 is the conversion factor for maximum CO2 generation per unit of BOD (1.375 g CO2 g BOD−1), CFCH4 is the conversion factor for maximum CH4 generation per unit of BOD (0.5 g CH4 g BOD−1), and BGCH4 is the fraction of carbon present as CH4 in generated biogas (assumed 65%).106 MCFWW is the methane correction factor for a wastewater treatment unit and λ is the biomass yield (g C converted to biomass per g C consumed).106 An MCFWW value of 0 and λ value of 0.65 were used for the assumed aerobic conditions in composting latrines. VIP latrines and dig and cover were considered to be 50% aerobic and 50% anaerobic; therefore, this study assumed half the mass was devoted to each process. Anaerobic conditions used a MCFWW value of 0.8 and λ value of 0.1.
Our study assumed that sufficient temperature was achieved for thermophilic composting and that users of composting toilets were correctly trained to add sufficient desiccant to obtain an optimal carbon-to-nitrogen ratio and aerate the pile through regular mixing of the solids, though we acknowledge that these assumptions are not always met in the field with the current technology and operating practices.23
In septic tanks, heavy particles settle to the bottom of the tank through sedimentation and anaerobically degrade. CO2 and CH4 emissions from the septic tank were obtained from a study of eight septic tanks that measured and reported emissions to equal 11% and 10% of the influent carbon, respectively.95 The CH4 emissions reported in that study are within the range reported by others.107–109 Approximately 65% of the influent carbon in that study was found to remain in the septic tank effluent,99 which aligns with the 30–40% BOD removal estimated by Tilley et al.39 Therefore, it was assumed that 14% of the influent carbon remained in the septic tank. Because the decomposition rate is slower than the accumulation rate, the growing volume of sludge (0.2 L per capita per day), which typically has BOD5 greater than 750 mg L−1, must be removed41,94 The sludge can be further treated through fecal sludge management and liquid effluent using a leach field.39 For the sewered toilet, dissolved organic carbon was assumed to decrease by 25% (14% of total COD) in an aerobic sewer environment that would produce carbon dioxide.108,110 Because another study noted that of the influent COD into a sewer, 5% is removed through exfiltration, 9% of COD is assumed to be converted into carbon dioxide.56 In the same study, 4% of the carbon entering the treatment plant was in sewage plant effluent, therefore 82% remains in sewage sludge.56 A summary of the resulting influent carbon that was allocated to a particular output stream for the seven sanitation technologies and strategies is provided in Table 2.
Table 2 Percentage of influent carbon assigned to effluent pathways for six sanitation technologies and one strategy
|
Sanitation technologies and strategies |
Dig and cover |
Bucket latrine |
VIP latrine |
Double-vault composting latrine |
Urine-diverting composting latrine |
Pour-flush toilet with septic tank |
Sewered toilet (with treatment) |
% lost to air phase as CO2 |
12 |
0 |
12 |
11 |
8 |
11 |
9 |
% lost to air phase as CH4 |
7 |
0 |
7 |
0 |
0 |
10 |
0 |
% lost to surrounding soil or effluent |
81 |
0 |
46 |
0 |
0 |
65 |
9 |
% recoverable as fecal sludge |
0 |
76 |
35 |
89 |
68 |
14 |
82 |
% recoverable as liquid effluent |
0 |
0 |
0 |
0 |
24 |
0 |
0 |
3.6 Health risks
The health risk associated with each of the onsite technologies and strategies examined in our study was examined previously.17 That study assessed the level of risk for users and the community (low/med/high). Users were defined as the individuals who utilize the sanitation technology or strategy and community was defined as anyone passively affected by living near or downstream of a utilized sanitation technology or strategy.17 The primary route of exposure is by humans directly contacting feces. This study did not include health risks along the service chain.
4 Results & discussion
4.1 Nutrient pathways and recovery (nitrogen, phosphorus, and carbon)
Sanitation technologies have varying capabilities to recover resources to enhance food security, reduce pollution from untreated waste, and reduce waterborne diseases.70 Dig and cover and bucket latrines (as opposed to container-based systems) are not good candidates for safe resource recovery; however, VIP latrines can recover sludge through manual or mechanical excavation. Mechanical removal of sludge into a slurry tanker may not be available or not well advertised; Grimason et al. noted that 74% of people in Blantyre (Malawi) were unaware that mechanical removal was an option.111 Composting latrines produce compost, a soil conditioner.41 Urine-separated composting latrines additionally produce urine, a nutrient-rich fertilizer. Septic tanks produce sludge that can be recovered through fecal sludge management. Sewered toilets have several options for resource recovery. Although sewer effluent without treatment is often reused in agriculture, the pathogens present may make this an unsafe option. With pathogen treatment, nutrients in the wastewater can be reused and more safely applied to crops.112 If centralized treatment does include nutrient management, nutrients such as nitrogen are often removed from the aqueous to atmospheric phase and not recovered. However, several technologies are available to recover nitrogen and phosphorus to align with the new paradigm of resource recovery.7,113
The Sankey diagrams produced for the one strategy and six sanitation technologies without further treatment are presented in Fig. 2a and b, 3a–c, and 4a and b. The seven figures are arranged into three groups based on their potential to recover the resources of nitrogen, phosphorus, and carbon and thus integrate sanitation provision with SDGs related to improving food security and sustainably managing waste and natural resources: group 1 (Fig. 2a and b) (unimproved, no resource recovery), group 2 (Fig. 3a–c) (improved, no resource recovery), and group 3 (Fig. 4a and b) (improved, resource recovery). Group 1 includes dig and cover and bucket latrines; group 2 includes VIP latrines and pour-flush toilets with septic tanks, and sewered toilets without further treatment; and group 3 includes double-vault composting latrine and urine-diverting composting latrine.
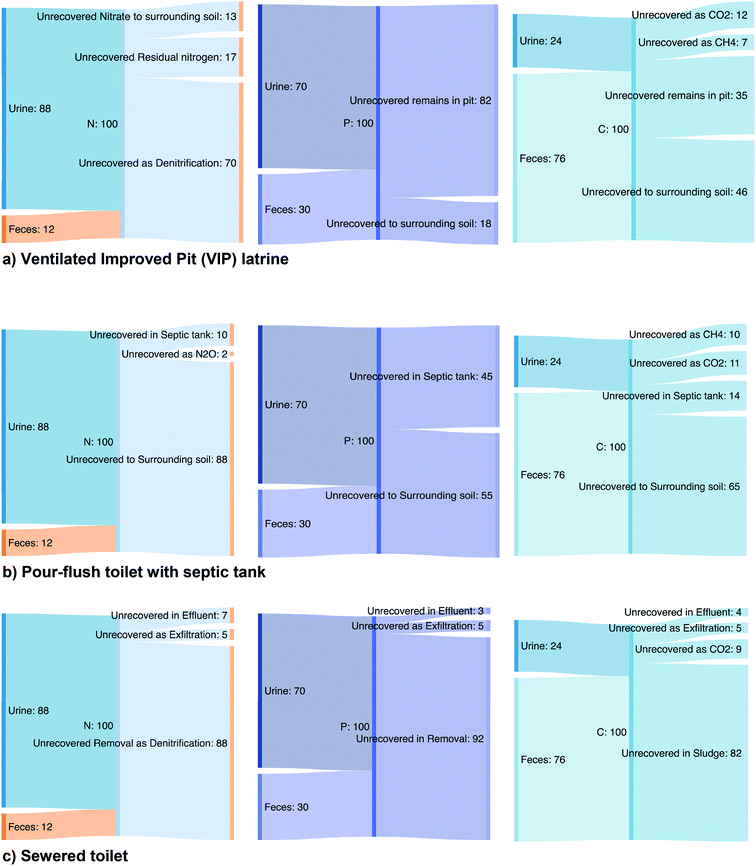 |
| Fig. 3 Sankey diagrams for group 2 (as-is, improved, no resource recovery) sanitation technologies (without further resource recovery treatment) that depict nitrogen, phosphorus, and carbon material flows as a percentage of input and output. Different color schemes are only used to contrast and differentiate pathways of nitrogen (left), phosphorus (middle), and carbon (right) recovery. | |
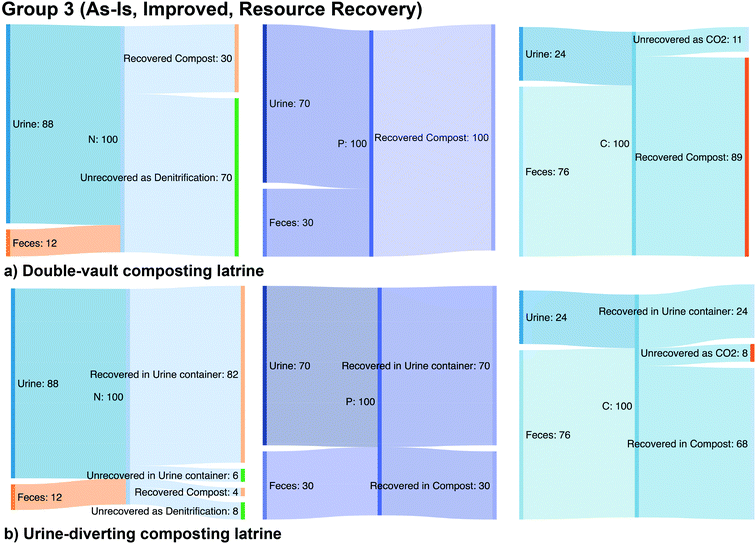 |
| Fig. 4 Sankey diagrams for group 3 (as-is, improved, resource recovery) sanitation technologies (without further resource recovery treatment) that depict nitrogen, phosphorus, and carbon material flows as a percentage of input and output. Different color schemes are only used to contrast and differentiate pathways of nitrogen (left), phosphorus (middle), and carbon (right) recovery. | |
The figures are arranged so the three subfigures associated with each figure are arranged (from left to right) as nitrogen, phosphorus, and carbon. Within each individual Sankey diagram, the number placed in the left of the first column is the percentage of nitrogen, phosphorus, or carbon in the influent that is found in urine and feces. The number to the right of the first column indicates that 100% of nitrogen, phosphorus or carbon is transferred from the urine and feces to the sanitation technology. The number in the middle column is the percentage of nitrogen, phosphorus, or carbon in specific identified effluent pathways. The number in the right column is percentage of a particular element that can be recovered (or not) and subsequently undergo post treatment/management to obtain the particular resource element. The discussion will address the potential for resource recovery and the pathogenic risk for each strategy and technology.
The group 1 strategy and technology of dig and cover and bucket latrines (Fig. 2a and b) have the lowest potential for resource recovery. In this study, dig and cover was presented as a baseline sanitation strategy for comparison to the six sanitation technologies. Even though dig and cover (Fig. 2a) returns the majority of nutrients back to the soil except for nitrogen, which is primarily lost to the atmosphere, this sanitation strategy has no potential to strategically recover nutrients because of the large number of distributed disposal sites associated with this strategy. No literature was identified regarding the recovery of resources from dig and cover as well. Bucket latrines do not recover resources without further resource recovery treatment.
The group 2 (improved, no resource recovery) sanitation technologies of a pour-flush toilet connected to a septic tank, a VIP latrine, and sewered toilet (Fig. 3a–c) are shown to have moderate potential to recover nitrogen, phosphorus, and carbon only if fecal sludge management40 or centralized resource recovery is implemented. Septic tanks can also recover water for use in irrigation.16 Centralized treatment does, however, provide opportunities to recover fit-for-purpose water, energy, and nutrients.114–118 Several centralized resource recovery options exist including anaerobic digestion for energy from biogas and struvite precipitation for nutrients.119–121 However, if the fecal sludge remains in the latrine pit or septic tank, which is the case when the pit or tank is not emptied, or if the fecal sludge is dumped without treatment, no resources can be recovered.
Promoting provision of sewers over fecal sludge management for onsite sanitation technologies is dominant in the majority of sanitation policies and projects.122 Sewered toilets without resource recovery treatment have little difference to the unimproved sanitation strategy of dig and cover in its ability to recover resources. Although the world community has affirmed through SDG target 6.3 that half of untreated wastewater should be treated in the next 15 years, the current situation is that collection of human waste and transport to a sewer does not typically result in treatment.58,59 Thus, we assume that resources (e.g., embedded nutrients) are not currently safely recovered from untreated wastewater. In fact, an estimated 1.5 billion people defined to have improved sanitation have sewage connections without any treatment, spreading disease and depleting water quality in the receiving water body and harming the well-being of fishers and other individuals who rely on the aquatic environment for their economic livelihood.2 Additionally, sewers are the most water-intensive technology examined in this study and water scarcity has been reported to be a barrier for the 46 million people who lack improved sanitation and also live in a water scarce area.42 Also, sewered toilets with treatment are the most expensive form of improved sanitation available on the sanitation ladder and also have higher embedded energy and associated carbon footprint.16
The two group 3 improved sanitation technologies, which include the double-vault composting latrine and the urine-diverting composting latrine, were shown to have the greatest potential for resource recovery (Fig. 4a and b). If operated properly, double-vault composting latrines are uniquely suited for nutrient recovery; Fig. 4a shows that 30% of the nitrogen, 100% of the phosphorus, and 89% of the carbon potentially remains in the compost, which can subsequently be used as a soil amendment. Fig. 4b shows that the integration of urine recovery with a composting latrine increases the potential to recover nitrogen (30% to 86%) because urine separation allows for the collection of a liquid that contains 88% of influent nitrogen and 70% of influent phosphorus. Fig. 4b also shows that the overall percentage of influent nitrogen, phosphorus, and carbon that is potentially recovered in that particular sanitation technology increases to 86%, 100%, and 92%, respectively. Subsequent use of urine and compost allow nutrients to be recycled into agricultural systems through beneficial reuse of waste that support multiple SDGs related to improving food security, sustainably managing natural resources, and reducing waste generation. Barriers to extensive deployment of composting latrines in urban locations have been identified.19
The as-is results (without further resource recovery treatment) show that as one moves up the sanitation ladder to what are considered more developed sanitation technologies (which also requires greater user costs and material and energy inputs) there is not necessarily a corresponding improvement in the potential for integrated resource recovery. Because resource recovery integrated with sanitation provision may require an additional post sanitation technology step, we estimated the percentage of nitrogen, phosphorus, and carbon that could be potentially recovered from our sanitation strategies and technologies if further resource recovery treatment (RRT) occurs. For example, this post sanitation technology RRT step could be fecal sludge management from a latrine or septic tank or centralized resource recovery for a conventional treatment plant.
Review of Table 3, which presents data modified from Fig. 2–4, shows that the technologies and strategies can be rearranged into three new groups if post sanitation technology resource recovery treatment can be implemented. In this case, group 1RRT (for resource recovery treatment step added) (unimproved, resource recovery) and group 2RRT (improved, resource recovery). Dig and cover remains in group 1 because it cannot be further treated as the feces are scattered and unable to be safely collected and recovered. Bucket latrines can be moved to group 1RRT because, although it is still an unimproved sanitation technology, the buckets can be emptied at a location where fecal sludge management can occur. This allows for some recovery of N, P, and C resources, particularly carbon. In group 2RRT are VIP latrines, pour-flush toilets with septic tank, which can recover resources through post sanitation management strategies such as fecal sludge management, and sewered toilets, which can recover embedded nutrients through resource recovery that takes place at a centralized plant. In fact, the sewered system connected to a treatment/resource recovery facility showed the largest increase in resource recovery potential for nitrogen, phosphorus, and carbon.
Table 3 Comparison of nitrogen, phosphorus, and carbon material flows as a percentage of input and output for improved sanitation technologies and one unimproved strategy before and after further resource recovery treatment (RRT) takes place
Technology |
Group |
Nitrogen recovery (%) |
Phosphorus recovery (%) |
Carbon recovery (%) |
Before RRT |
After RRT |
Before RRT |
After RRT |
Before RRT |
After RRT |
Before RRT |
After RRT |
Dig and cover |
1 |
0 |
0 |
0 |
Bucket latrine |
1 |
1RRT |
0 |
≤12 |
0 |
≤30 |
0 |
≤76 |
VIP latrine |
2 |
2RRT |
0 |
≤17 |
0 |
≤82 |
0 |
≤35 |
Pour-flush w/ septic |
2 |
2RRT |
0 |
≤10 |
0 |
≤45 |
0 |
≤14 |
Sewered without tmt |
2 |
2RRT |
0 |
≤88 |
0 |
≤92 |
0 |
≤82 |
Double-vault composting latrine |
3 |
≤30 |
≤100 |
≤89 |
Urine-diverting composting latrine |
3 |
≤86 |
≤100 |
≤92 |
Three group 2RRT improved technologies and one group 1RRT unimproved technology can recover resources through fecal sludge management; therefore, setting up fecal management programs is essential.50 The number of people who utilize onsite technologies like latrines and septic tanks, which require fecal sludge management, is expected to rise from 2.7 billion to 5.4 billion by 2030.51 A fecal sludge management study of 12 cities found that approximately 67% of households utilized onsite sanitation, but only 22% of those same households safely managed their fecal waste.123 VIP latrines and pour-flush toilets that discharge to a septic tank have potential for resource recovery. In a septic tank, the fecal sludge retains 10%, 20%, and 14% of the influent nitrogen, phosphorus, and carbon, respectively (as shown in Table 3). Likewise, the fecal sludge in the pit of a VIP latrine retains 17%, 82%, and 35% of the influent nitrogen, phosphorus, and carbon, respectively.
Traditionally for a latrine connected to a pit, a new pit is dug once the old pit is filled; however, digging new pits can be inconvenient and may not be an option in dense urban settings.122 Therefore, pit contents are often removed manually with a bucket or mechanically with a slurry tanker. The emptying and collection of fecal sludge from pits and tanks can be a difficult step in the sanitation service train for technical, economic, and health reasons. Technically, collection trucks may not have sufficient space in dense urban settings to access the stored fecal sludge.41 Some collection methods are unable to adequately remove septic tank solids; for example, in Dakar (Senegal) septic tanks were observed to be emptied by slurry tankers with pumps instead of vacuums, which are unable to remove settled solids.124,125 Furthermore, when pumping solids from a latrine pit, an operator typically has to jet water into the pit to break up solids so they are more easily transported to the surface. This, however, results in a more diluted waste stream, which may lower the potential for subsequent resource recovery. The treatment processes may depend on the characteristics of the input fecal sludge, which varies based on its source.41 Economically, household users may not have the money available for emptying services. Additionally, if fecal sludge treatment is nonexistent, inconvenient, or expensive, the collected untreated fecal sludge could be illegally dumped, presenting an adverse risk to humans and the environment.32 This is especially important because it is estimated that 1.2 billion people in the world are currently in need of fecal waste management126 and a study in Sub-Saharan Africa reported only 25% of collected fecal sludge is transported for subsequent treatment.127 This issue is important for health reasons because improper collection, transport, and reuse of the sludge along the sanitation service chain can lead to distribution of pathogens over a large expense of an urban setting.
Many potential beneficial products can be derived from fecal sludge.49 One of the most common outputs is use of the resulting solids as a soil conditioner which can be used, for example, by low-income farmers to increase food production.17 Sources of soil conditioner include untreated fecal sludge, dried fecal sludge, compost, sludge pellets, and digestate from anaerobic digestion.41 Other products that can be recovered from fecal sludge include production of energy via anaerobic digestion and incineration or pyrolysis of fecal sludge.39,41,70,128 The recovery of energy from fecal sludge can thus also support SDG 7, which seeks to ensure access to affordable, reliable, sustainable and modern energy for all. Therefore, the ability to integrate resource recovery with management of fecal sludge presents a new market opportunity, especially in Asian and African cities.129 Resource recovery also provides the ability to pursue carbon neutrality in the sanitation sector by obtaining carbon offsets associated with recovering embedded nutrients and energy.8,16 However, goals of worker safety and resource recovery must be considered in all the sanitation service chain steps that include emptying, transport, treatment, and reuse/disposal of fecal sludge.130,131
4.2 Issues of centralization and decentralization
Centralized and decentralized systems have co-existed for many decades. Thus, it is expected that meeting the sanitation targets of the SDGs will require both strategies. The advantages and disadvantages of both these systems are reviewed elsewhere.132,133 Onsite systems require greater responsibilities of users regarding correct operation and maintenance of the technology. Adding resource recovery to this responsibility may increase user complexity. One advantage of decentralized systems is their ability to return water back to the watershed of origin. Similarly, centralized systems can be readily integrated with fit-for-purpose water reuse in the many urbanizing areas of the world that are already integrated with local agriculture.111,130 This is important because reclaimed water is believed to currently be the highest value resource in wastewater. Hybrid systems that use a combination of onsite and centralized collection and treatment technologies may also be enhance resource recovery. However, the selection of the particular deployment strategy with regards to its resource recovery potential ultimately depends on the location of the waste generator relative to existing or planned collection and treatment systems.134 In either case, there are barriers to both strategies that include a global need to accelerate innovation and adoption of reliable sanitation technologies that enhance integrated resource recovery.135
4.3 Greenhouse gas emissions
Although quantification of greenhouse gas emissions are common for centralized wastewater treatment,136 greenhouse gas emissions from onsite systems are not as well understood.94,106Table 2 suggests there is minimal difference in the carbon dioxide and methane greenhouse gas emissions from the studied strategy and technologies. Carbon dioxide emissions ranged from 0% to 12% and methane emissions ranged from 0–10% of the influent carbon from urine and feces for the seven technologies and strategies investigated. The greatest contribution of greenhouse gas emissions is from methane, which has a 100 year global warming potential that is 25 times the impact of carbon dioxide.137 Therefore, sanitation technologies and strategies with anaerobic zones that produce methane (e.g. dig and cover, VIP latrines, and pour-flush toilets with septic tanks) are worse options from a greenhouse gas emissions perspective than aerobic technologies like properly operated composting latrines because the methane is emitted to the atmosphere and not captured.106 Additionally, more research is needed to quantify the contribution of nitrous oxide to greenhouse gas emissions from sanitation technologies. To reduce emissions, latrine pits should be kept above the water table to limit groundwater contamination and reduce the amount of organics in the submerged anaerobic zones.106,138,139 If further resource recovery treatment is implemented, greenhouse gas emissions from these additional technologies may need to be considered. For example, fecal sludge management technologies such as anaerobic digestion should be properly managed so that additional methane is not emitted into the atmosphere (like has been raised as a concern for implementation of small-scale biodigesters).140
4.4 Health risks
Table 4 shows that the strategy and technologies can be placed in the following order of most risk to least risk to the health of the user and community: 1) dig and cover, 2) bucket latrine, 3) VIP latrine, 4) sewered toilet, 5) pour-flush toilet with septic tank, 6) urine-diverting composting latrine, and 7) double-vault composting latrine. It should be noted that pathogens such as soil-transmitted helminths are a particular health concern in many parts of the world not currently served by improved sanitation, and their persistent in some sanitation and sludge management strategies (e.g., composting latrines, anaerobic digestion) needs to be taken into consideration.23,71,141
Table 4 Pathogen risk summary for household sanitation technologies17
|
Dig and cover |
Bucket latrine |
VIP latrine |
Sewered toilet |
Pour-flush toilet with septic tank |
Urine-diverting composting latrine |
Double-vault composting latrine |
Level of user risk |
High |
Medium |
Medium |
Worker: medium |
Low-medium |
Low |
Low |
Level of community risk |
High |
Medium-high |
Medium |
Low-medium |
Low-medium |
Medium |
Low |
4.5 Significance
The results of this study are significant because the recently adopted sustainable development goal and associated target that seek to provide improved sanitation to the 2.4 billion people lacking sanitation should be more closely aligned with the transition to the green economy that promotes technologies that are socially inclusive, low-carbon, and resource efficient.142 The results from this study are especially important to regions of Sub-Saharan Africa and Asia that have large populations currently unserved by improved sanitation and thus have a great opportunity to integrate innovative resource recovery strategies and technologies with improvements in sanitation coverage. One important consideration in managing collected wastewater or fecal sludge for treatment and resource recovery is that smaller cities (and farmers) often have less technological, institutional, or financial support than larger cities for environmental services.143 In fact, the majority reason for lack of performance or failure of sanitation infrastructure in developing countries is identified as the absence of maintenance.144 This reason is equally important for centralized and onsite systems in regards to proper operation and safe handling of recovered resources across the sanitation service chain. SDG targets related to sanitation provision can be integrated with SDG targets related to improving food security, providing renewable energy, and sustainably managing wastes and natural resources. The global community is thus at a critical juncture where it has the opportunity to rethink how to provide adequate access to improved sanitation so they may better achieve sustainable development for current and future generations.
5 Conclusions
In this study, literature on the ability to integrate resource recovery with existing sanitation technologies and one management strategy was critically reviewed and supported by comparing the associated material flows of nitrogen, phosphorus, and carbon. The work contributes to the discussion on achieving multiple SDG targets related to improved sanitation provision, food security, sustainable management and efficient use of natural resources, waste generation reduction, and sustainable and resilient agriculture practices through recovery of beneficial resources found in human waste. Results showed the six sanitation technologies and one strategy assessed, without further resource recovery treatment, could be grouped into three categories based on their ability to recover resources. Group 1 (dig and cover and bucket latrines) have no resource recovery potential. Group 2 technologies (ventilated improved pit (VIP) latrine, pour-flush toilet with septic tank, and sewered toilet without treatment) provide improved sanitation but had no resource recovery potential unless a resource recovery step was added to treatment. Group 3 technologies (double-vault composting latrine and urine-diverting compositing latrine) had the greatest ability to achieve resource recovery. They however will require careful consideration of safe handling of collected resources which is important for both centralized and onsite systems. If further fecal sludge management or centralized resource recovery treatment occurs, the following technologies and strategies are also shown to have strong potential to recover resources: bucket latrine, VIP latrine, pour-flush toilet with a septic tank, and sewered toilet. Different technologies also are shown with different potential to recover specific resources.
One limitation of the study is the assumptions made in development of the material flow diagrams. The most reliable peer reviewed data were used as inputs whenever possible; however, sometimes data were available only from a single study that was based on a specific cultural or geographical context. Even larger picture data on wastewater management data (volume of water related to production, treatment, reuse) is only available for fifty-five countries.32 Additional studies would provide greater confidence in the total quantity of nutrients that could be potentially recovered along with the greenhouse gases produced during treatment and recovery. We also acknowledge that proper user compliance is assumed for safe recovery of the resources from decentralized technologies such as composting latrines. This complex challenge of how to safely manage a large number of distributed onsite sanitation systems that may not be regulated should be considered against the additional economic and environmental costs of centralized technologies. For example, one study for example concluded that capital and operating costs for a centralized activated sludge treatment system were five times greater than a decentralized septic tank system that also integrated fecal sludge management.145 Greater material and energy intensity is also required to provide collection systems integrated with centralized treatment and resource recovery.13 Centralized treatment does provide the opportunity to recover fit-for-purpose water, which was not a focus of this paper. However, both centralized and decentralized systems will be needed to meet sanitation needs in urban and rural settings and resulting resources that are covered must be safely managed to achieve multiple sustainable development goals.
Conflicts of interest
There are no conflicts to declare.
Acknowledgements
This material is based upon work supported by the National Science Foundation under Grant No. OISE-1243510.
References
- United Nations (UN), http://www.un.org/millenniumgoals/environ.shtml (accessed September 2017).
- R. Baum, J. Luh and J. Bartram, Environ. Sci. Technol., 2013, 47, 1994–2000 CrossRef CAS PubMed.
- A. Prüss-Ustün, J. Bartram, T. Clasen, J. M. Colford, O. Cumming, V. Curtis, S. Bonjour, A. D. Dangour, J. De France, L. Fewtrell, M. C. Freeman, B. Gordon, P. R. Hunter, R. B. Johnston, C. Mathers, D. Mäusezahl, K. Medlicott, M. Neira, M. Stocks, J. Wolf and S. Cairncross, Trop. Med. Int. Health, 2014, 19, 894–905 CrossRef PubMed.
- L. Fewtrell, R. B. Kaufmann, D. Kay, W. Enanoria, L. Haller and J. M. Colford, Lancet Infect. Dis., 2005, 5, 47–52 CrossRef.
- H. Waddington and B. Snilstveit, J. Dev. Effect., 2009, 1, 295–335 CrossRef.
- M. S. M. Jetten, S. J. Horn and M. C. M. van Loosdrecht, Water Sci. Technol., 1997, 35, 171–180 CAS.
- J. S. Guest, S. J. Skerlos, J. L. Barnard, M. B. Beck, G. T. Daigger, H. Hilger, S. J. Jackson, K. Karvazy, L. Kelly, L. Macpherson, J. R. Mihelcic, A. Pramanik, L. Raskin, M. C. M. van Loosdrecht, D. Yeh and N. G. Love, Environ. Sci. Technol., 2009, 43, 6126–6130 CrossRef CAS PubMed.
- W. Mo and Q. Zhang, Environ. Manage., 2012, 112, 360–367 CAS.
- J. H. Ahn, S. Kim, H. Park, B. Rahm, K. Pagilla and K. Chandran, Environ. Sci. Technol., 2010, 44, 4505–4511 CrossRef CAS PubMed.
- H. Yoshida, J. Mønster and C. Scheutz, Water Res., 2014, 61, 108–118 CrossRef CAS PubMed.
-
J. Eliasson, Press Release, United Nations, May 29, 2014. http://www.un.org/press/en/2014/dsgsm778.doc.htm (accessed September 2017) Search PubMed.
- United Nations (UN), https://sustainabledevelopment.un.org/content/documents/21252030%20Agenda%20for%20Sustainable%20Development%20web.pdf (accessed September 2017).
- United Nations Population Fund (UNFPA), http://www.unfpa.org/world-population-trends (accessed September 2015).
- J. R. Mihelcic, L. M. Fry and R. Shaw, Chemosphere, 2011, 84, 832–839 CrossRef CAS PubMed.
- T. A. Larsen, A. C. Alder, R. I. L. Eggen, M. Maurer and J. Lienert, Environ. Sci. Technol., 2009, 43, 6121–6125 CrossRef CAS PubMed.
- P. K. Cornejo, Q. Zhang and J. R. Mihelcic, J. Environ. Manage., 2013, 131, 7–15 CrossRef CAS PubMed.
-
T. A. Stenström, R. Seidu, N. Ekane and C. Zurbrügg, in EcoSanRes Programme, Microbial Exposure and Health Assessments in Sanitation Technologies and Systems, Stockholm Environment Institute, Sweden, 2011, parts 2–3, pp. 10–114 Search PubMed.
-
C. Luthi, A. Panesar, T. Schutze, A. Norstrom, J. McConville, J. Parkinson, D. Saywell and R. Ingle, Sustainable Sanitation in Cities: A framework for action, Papiroz Publishing House, Rijswijk, 2011 Search PubMed.
- C. K. Anand and D. S. Apul, J. Waste Manage., 2014, 34, 329–343 CrossRef PubMed.
- B. Nawab, I. L. P. Nyborg, K. B. Esser and P. D. Jenssen, J Environ Psychol., 2006, 26, 236–246 CrossRef.
- A. Cordova and B. A. Knuth, Urban Water J., 2005, 2, 245–262 CrossRef.
- M. R. Templeton, Environ. Sci.: Water Res. Technol., 2015, 1, 17–21 Search PubMed.
- J. Mehl, J. Kaiser, D. Hurtado, D. A. Gibson, R. Izurieta and J. R. Mihelcic, J. Water Health, 2011, 9, 187–199 CrossRef PubMed.
- M. E. Magri, L. S. Philippi and B. Vinneras, Appl. Environ. Microbiol., 2013, 79, 2156–2163 CrossRef CAS PubMed.
- J. T. Trimmer, N. Nakyanja, R. Ssekubugu, M. Sklar, J. R. Mihelcic and S. J. Ergas, J. Water, Sanit. Hyg. Dev., 2016, 6, 259–268 CrossRef.
- Q. Zhang, C. Prouty, J. B. Zimmerman and J. R. Mihelcic, Engineering, 2016, 2, 481–489 CrossRef.
- L. Fewtrell, R. B. Kaufmann, D. Kay, W. Enanoria, L. Haller and J. M. Colford Jr, Lancet, 2015, 5, 42–52 CrossRef.
- S. Cairncross, C. Hunt, S. Boisson, K. Bostoen, V. Curtis, I. C. H. Fung and W. Schmidt, Int. J. Epidemiol., 2010, 39, i193–i205 CrossRef PubMed.
- K. Ziegelbauer, B. Speich, D. Mausezahl, R. Bos, J. Keiser and J. Utzinger, PLoS Med., 2012, 9, 1–17 Search PubMed.
- E. C. Strunz, D. G. Addiss, M. E. Stocks, S. Ogden, J. Utzinger and M. C. Freeman, PLoS Med., 2014, 11, 1–38 Search PubMed.
- A. Y. Katukiza, M. Ronteltap, C. B. Niwagaba, J. W. A. Foppen, F. Kansiime and P. N. L. Lens, Biotechnol. Adv., 2012, 30, 964–978 CrossRef CAS PubMed.
- K. Andersson, S. Dickin and A. Rosemarin, Sust, 2016, 8, 1289–1303 CrossRef.
- S. Semiyaga, M. A. E. Okure, C. B. Niwagaba, A. Y. Katukiza and F. Kansiime, Resour., Conserv. Recycl., 2015, 104, 109–119 CrossRef.
- R. Gine-Garriga, O. Flores-Baquero, A. Jimenez-Fedez de Palencia and A. Perez-Foguet, Sci. Total Environ., 2017, 580, 1108–1119 CrossRef CAS PubMed.
- J. T. Trimmer, R. D. Cusick and J. S. Guest, Environ. Sci. Technol., 2017, 51, 10765–10776 CrossRef CAS PubMed.
- A. A. Forbis-Stokes, P. F. O'Meara, W. Mugo, G. M. Simiyu and M. A. Deshusses, Environ. Eng. Sci., 2016, 33, 898–906 CrossRef CAS PubMed.
- R. A. Bair, O. Y. Ozcan, J. L. Calabria, G. H. Dick and D. H. Yeh, Water Sci. Technol., 2015, 72, 1543–1551 CrossRef CAS PubMed.
- C. Furlong, M. R. Templeton and W. T. Gibson, J. Water, Sanit. Hyg. Dev., 2014, 4, 231–239 CrossRef.
-
E. Tilley, L. Ulrich, C. Luthi, P. Reymond and C. Zurbrugg. Compendium of Sanitation Systems and Technologies, Swiss Federal Insitute of Aquatic Science and Technology, Switzerland, 2nd edn, 2014 Search PubMed.
- Gates Foundation, http://www.gatesfoundation.org/What-We-Do/Global-Development/Reinvent-the-Toilet-Challenge (accessed September 2017).
-
L. Strande, M. Ronteltap and D. Brdjanovic, Faecal Sludge Management: Systems Approach for Implementation and Operation, IWA Publishing, London, 2014, ch. 1–2, pp. 1–44 Search PubMed.
- L. M. Fry, J. R. Mihelcic and D. W. Watkins, Environ. Sci. Technol., 2008, 42, 4298–4304 CrossRef CAS PubMed.
-
New Partnership for Africa's Development (NEPAD), Water in Africa: Management Options to Enhance Survival and Growth, Economic Commission for Africa, 2006 Search PubMed.
-
Charting our water future: Economic frameworks to inform decision-making, ed. C. Douglas, 2030 Water Resources Group, 2009 Search PubMed.
- World Health Organization (WHO), http://www.who.int/water_sanitation_health/monitoring/coverage/indicator-6-2-1-safely-managed-sanitation-services-and-hygiene.pdf?ua=1 (accessed June 2017).
- R. Kennedy-Walker, T. Holderness, D. Alderson, J. M. Amezaga and C. A. Paterson, Environ. Sci.: Water Res. Technol., 2016, 2, 97–106 Search PubMed.
-
W. Berger, Technology review of composting toilets – Basic overview of composting toilets (with or without urine diversion), Deutsche Gesellschaft für Internationale Zusammenarbeit (GIZ) GmbH, Eschborn, Germany, 2011 Search PubMed.
- S. Tilmans, K. Russel, R. Sklar, L. Page, S. Kramer and J. Davis, Environ. Urban., 2015, 27, 89–104 CrossRef PubMed.
- Practical Action. https://answers.practicalaction.org/our–resources/item/ventilated–improved–pit–latrine (accessed September 2017).
-
EAWAG and D. Spuhler, http://www.sswm.info/category/implementation-tools/water-use/hardware/toilet-systems/composting-toilets (accessed September 2017).
- M. Maurer, P. Schwegler and T. A. Larsen, Water Sci. Technol., 2003, 48, 37–46 CAS.
- W. Rauch, D. Brockmanna, I. Petersa, T. A. Larsen and W. Gujer, Water Res., 2003, 37, 681–689 CrossRef CAS PubMed.
- J. A. Wilsenach, C. A. H. Schuurbiers and M. C. M. van Loosdrecht, Water Res., 2007, 41, 458–466 CrossRef CAS PubMed.
-
J. R. Mihelcic, E. A. Myre, L. M. Fry, L. D. Phillips and B. B. Barkdoll, in Field Guide to Environmental Engineering for Development Workers: Water, Sanitation, Indoor Air, American Society of Civil Engineers (ASCE) Press, Reston, VA, 2009, ch. 20, pp. 381–408 Search PubMed.
- Global Water Pathogens Project (GWPP), www.waterpathogens.org/.
- S. Gray and N. Becker, Urban Water, 2002, 4, 331–346 CrossRef CAS.
- Boston Consulting Group https://www.bcg.com/documents/file178928.pdf (accessed June 2017).
-
Sick Water? The Central Role of Wastewater Management in Sustainable Development, ed. E. Corcoran, C. Nellemann, E. Baker, R. Bos, D. Osborn and H. Savelli, UN Environmental Programme, Nairobi, 2010 Search PubMed.
- PRWEB. www.prweb.com/releases/2016/09/prweb13717685.htm (accessed September 2017).
- T. Sato, M. Qadir, S. Yamamoto, T. Endo and A. Zahoor, Agric. Water Manage., 2013, 130, 1–13 CrossRef.
-
UNEP, International Source Book on Environmentally Sound Technologies for Wastewater and Stormwater Management, UNEP Division of Technology, Industry and Economics, Osaka, 2004 Search PubMed.
-
I. Gunther, A. Horst, C. Luthi, M. H. Joachim, B. C. Niwagaba and K. I. Tumwebaze, Where do Kampala's poor “go”? Urban sanitation conditions in Kampala's low-income areas. Research evidence for policy No. 1, ETH, Zurich, 2011 Search PubMed.
-
A. Robinson, Sanitation Finance in Rural Cambodia, Water and Sanitation Program, 2012 Search PubMed.
-
E. Kvarnstrom, K. Emilsson, A. R. Stintzing, M. Johansson, H. Jonsson, E. af Petersens, C. Schonning, J. Christensen, D. Hellstrom, L. Qvarnstrom, P. Ridderstolpe and J. Drangert, Urine Diversion: One Step Towards Sustainable Sanitation, Stockholm Environmental Institute, http://www.ecosanres.org/pdf_files/Urine_Diversion_2006-1.pdf (accessed November 2017) Search PubMed.
- Sustainable Sanitation Alliance (SuSanA). http://www.Sfd.susana.org.
- United States Energy Information Administration (US EIA), http://www.eia.gov/totalenergy/data/annual/ (accessed September 2017).
-
D. I. W. Ddiba, K. Andersson and A. Rosemarin, Resource Value Mapping (REVAMP): A tool for evaluating the resource recovery potential of urban waste streams, Stockholm Environment Institute, Stockholm, 2016 Search PubMed.
- Sankeymatic, www.sankeymatic.com (accessed September 2015).
- C. Rose, A. Parker, B. Jefferson and E. Cartmell, Environ. Sci. Technol., 2015, 45, 1827–1879 CrossRef CAS PubMed.
- H. Heinonen-Tanski and C. Wijk-Sijbesma, Bioresour. Technol., 2004, 96, 403–411 CrossRef PubMed.
-
R. G. Feachem, D. J. Bradley, H. Garelick and D. D. Mara, Sanitation and disease: health aspects of excreta and wastewater management, World Bank Studies in Water Supply and Sanitation 3, Wiley, Chichester, 1983 Search PubMed.
-
C. Lentner, Geigy scientific tables: −1: Units of measurement, body fluids, composition of the body, CIBA-Geigy, Basle, 1981 Search PubMed.
- N. L. Schouw, S. Danteravanich, H. Mosbaek and J. C. Tjell, Sci. Total Environ., 2002, 286, 155–166 CrossRef CAS PubMed.
-
L. Ulrich and C. Luthi, 2nd edition of the Compendium of Sanitation Systems and Technologies, Sandec News, Dubendorf, 2014 Search PubMed.
-
B. Vinneras, PhD Thesis, Swedish University of Agricultural Sciences, 2002 Search PubMed.
-
J. J. R. Frausto da Silva and R. J. P. Williams, The Biological Chemistry of the Elements – The Inorganic Chemistry of Life, Oxford University Press, Oxford, 1997 Search PubMed.
-
D. Del Porto and C. Steinfeld in The Composting System Book: A Practical Guide to Choosing, Planning, and Maintaining Composting Toilet Systems, an Alternative to Sewer and Septic Systems, ed. P. Nesbitt and D. Dwyer, The Center for Ecological Pollution Prevention, Concord, MA, 1999, ch. 3, pp. 15–30 Search PubMed.
-
R. Gensch, H. Jönsson, T. A. Stenström and L. Dagerskog, Stockholm Environmental Institute, EcoSanRes Services, http://www.ecosanres.org/pdf_files/ESR2010-1-PracticalGuidanceOnTheUseOfUrineInCropProduction.pdf. (accessed September 2017).
-
S. A. Esrey, I. Andersson, A. Hillers and R. Sawyer, Closing the Loop: Ecological sanitation for food security, UNDP, Morelos, 2000 Search PubMed.
-
E. Von Munch and M. Winker, Technology review of urine diversion components, Deutche Gesellschaft fur Internationale Zusammenarbeit, Eschborn, 2011 Search PubMed.
- G. Jacks, F. Sefe, M. Carling, M. Hammar and P. Letsamao, Environ. Geol., 1999, 38, 199–203 CrossRef CAS.
- K. L. Nelson and A. Murray, Annu. Rev. Environ. Resour., 2008, 33, 119–151 CrossRef.
-
U. Winblad and M. Simpson-Hebert, Ecological Sanitation, Stockholm Environmental Institute, Stockholm, 2004 Search PubMed.
-
G. Tredoux, A. S. Talma and J. F. P. Engel, presented in part at WISA 2000 Biennial Conference, Sun City, South Africa, May, 2000 Search PubMed.
- E. Zingoni, D. Love, C. Magadza, W. Moyce and K. Musiwa, Phys. Chem. Earth, 2005, 30, 680–688 CrossRef.
- B. Dzwairo, Z. Hoko, D. Love and E. Guzha, Phys. Chem. Earth, 2006, 31, 779–788 CrossRef.
- E. C. Okafor and K. Opuene, Int. J. Environ. Sci. Technol., 2007, 4, 233–240 CrossRef CAS.
- I. Chenini and S. Khemiri, Int. J. Environ. Sci. Technol., 2009, 6, 509–519 CrossRef CAS.
- S. Uenosono, S. Takahashi, M. Nagatomo and S. Yamamuro, Soil Sci. Plant Nutr., 2002, 48, 9–13 CrossRef CAS.
- E. R. Fuhrmeister, K. J. Schwab and T. R. Julian, Environ. Sci. Technol., 2015, 49, 11604–11611 CrossRef CAS PubMed.
- S. Wohlsager, J. Clemens, P. T. Nguyet, A. Rechenburg and U. Arnold, Water Environ. Res., 2010, 82, 840–847 CrossRef CAS PubMed.
-
O. B. Kaplan, in Septic Systems Handbook, Lewis Publishers, Boca Raton, FL, 2nd edn, 1991, ch. 12, pp. 145–154 Search PubMed.
- M. Pell and F. Nyberg, J. Environ. Qual., 1989, 18, 451–467 CrossRef CAS.
- R. Laak, R. Costello and M. A. Parese, J. Environ. Eng., 1981, 107, 581–590 CAS.
- H. Philip, S. Maunoir, A. Rambaud and L. S. Philippi, Water Sci. Technol., 1993, 28, 57–64 CAS.
- L. R. Diaz-Valbuena, H. L. Leverenz, C. D. Cappa, G. Tchobanoglous, W. R. Horwath and J. L. Darby, Environ. Sci. Technol., 2011, 45, 2741–2747 CrossRef CAS PubMed.
- F. Jiang, Y. Chen, H. R. Mackey and M. C. van Loosdrecht, Water Sci. Technol., 2011, 64, 618–626 CrossRef CAS PubMed.
-
J. P. Padmasiri, G. M. Jayatilake and J. P. K. Kotuwegedara, presented in part at 18th WEDC Conference, Kathmandu, Nepal, September 1992 Search PubMed.
-
National Environmental Services Center (NESC), Phosphorus and Onsite Wastewater Systems, West Virginia University, 2013, vol. 24, no. 1 Search PubMed.
- H. Kirchmann and S. Petterson, Fert. Res., 1995, 40, 149–154 CrossRef.
- Lombardo Associates, Inc., http://lombardoassociates.com/pdfs/060410-P-Geochemistry-FINAL-LAI-Version.pdf (accessed September 2017).
- J. N. Bhagwan, D. Still, C. Buckley and K. Foxon, Water Sci. Technol., 2008, 58, 21–27 CrossRef CAS PubMed.
- P. Czepiel, P. Crill and R. Harriss, Environ. Sci. Technol., 1995, 29, 2352–2356 CrossRef CAS PubMed.
- Y. Law, L. Ye, Y. Pan and Z. Yuan, Philos. Trans. R. Soc., B, 2012, 367, 1265–1277 CrossRef CAS PubMed.
- M. C. Reid, K. Guan, F. Wagner and D. L. Mauzerall, Environ. Sci. Technol., 2014, 48, 8727–8734 CrossRef CAS PubMed.
-
A. Zouboulis and A. Tolkou, in Managing Water Resources under Climate Uncertainty, Springer International Publishing, 2015, pp. 197–220 Search PubMed.
-
Environmental Protection Agency (EPA), Greenhouse Gas Emissions Estimation Methodologies for Biogenic Emissions from Selected Source Categories, RTI International Work Assignment 4–18, Washington, D.C., 2010 Search PubMed.
-
H. S. Eggleston, L. Buendia, K. Miwa, T. Ngara and K. Tanabe, 2006 IPCC guidelines for national greenhouse gas inventories, National Greenhouse Gas Inventories Programme, IGES, Japan, 2006 Search PubMed.
-
L. P. Kinnicutt, C. E. A. Winslow and R. W. Pratt, in Sewage Disposal, John Wiley and Sons, New York, 1910, ch. 13, pp. 406–441 Search PubMed.
- K. Raunkjaer, T. Hvitved-Jacobsen and P. H. Nielsen, Water Environ. Res., 1995, 67, 181–188 CrossRef CAS.
- A. M. Grimason, K. C. Tembo, G. C. Jabu and M. H. Jackson, J. R. Soc. Promot. Health, 2000, 120, 175–182 CrossRef CAS PubMed.
- M. E. Verbyla, S. M. Oakley and J. R. Mihelcic, Environ. Sci. Technol., 2013, 47, 3598–3605 CrossRef CAS PubMed.
- K. D. Orner, O. Y. Ozcan, D. Saetta, T. H. Boyer, D. H. Yeh, D. Anderson and J. A. Cunningham, Environ. Eng. Sci., 2017 DOI:10.1089/ees.2017.0016.
- T. Asano and A. D. Levin, Water Sci. Technol., 1996, 33, 1–14 CAS.
- Water Environment Research Foundation (WE&RF) Nutrient Recovery State of the Knowledge. www.werf.org/c/2011Challenges/Nutrient_Recovery.aspx.
- J. R. Mihelcic, L. M. Fry and R. Shaw, Chemosphere, 2011, 84, 832–839 CrossRef CAS PubMed.
- J. Nouri, K. Naddafi, R. Nabizadeh and M. Jafarinia, Environ. Pollut., 2007, 4, 145–149 CAS.
- P. L. McCarty, J. Bae and J. Kim, Environ. Sci. Technol., 2011, 45, 7100–7106 CrossRef CAS PubMed.
- American Biogas Council, https://www.americanbiogascouncil.org/pdf/ABC%20Biogas%20101%20Handout%20NEW.pdf (accessed September 2017).
-
W. O. Khunjar and J. Fisher, Water Environment Reuse Foundation, http://www.weat.org/Presentations/2014Eckenfelder_v1_HSandCH.pdf (accessed September 2017).
-
A. Milbrandt, A Geographic Perspective on the Current Biomass Resource Availability in the United States, Technical Report, National Renewable Energy Laboratory, NREL/TO-560-39181, 2005 Search PubMed.
- International Bank for Reconstruction and Development/The World Bank, Targeting the Urban Poor and Improving Services in Small Towns, The Missing Link in Sanitation Service Delivery, A Review of Fecal Sludge Management in 12 Cities, 2014 Search PubMed.
-
I. Blackett, P. Hawkins and C. Heymans, The Missing Link in Sanitation Service Delivery, A Review of Fecal Sludge Management in 12 Cities, Water and Sanitation Program (WSP), International Bank for Reconstruction and Development/The World Bank, 2014 Search PubMed.
-
S. T. Diongue, Master's Thesis, Institut International du Génie de l'Eau et de l'Environnement (Burkina Faso)/Ecole Ploytechnique Fédérale de Lausanne (Suisse), 2006 Search PubMed.
-
E. M. Sonko, Traitement des boues de vidange de systèmes autonomes d'assainissement à Dakar (Sénégal): évaluation de l'efficacité de la séparation solide/liquide de lits de séchage non plantés soumis à différentes charges de boues de vidange et à divers apports, DEA: Institut des Sciences de l'Environnement, UCAD, 2008 Search PubMed.
- Y. P. Thye, M. R. Templeton and M. Ali, Crit. Rev. Environ. Sci. Technol., 2011, 41, 1793–1819 CrossRef.
-
Boston Consulting Group (BCG), Omni Ingestor global market sizing project: Final deliverable part B: Complete compendium, Boston, 2012 Search PubMed.
- S. Diener, S. Semiyaga, C. B. Niwagaba, A. M. Muspratt, J. B. Gning, M. Mbeguere, J. E. Ennin, C. Zurbrugg and L. Strande, Resour., Conserv. Recycl., 2014, 88, 32–38 CrossRef.
-
S. Chowdry and D. Kone, Business Analysis of Fecal Sludge Management: Emptying and Transportation Services in Africa and Asia, The Bill & Melinda Gates Foundation, Seattle, 2012 Search PubMed.
- United Nations (UN). http://www.unwater.org/publications/wastewater-management-un-water-analytical-brief/ (accessed September 27, 2017).
- A. A. Forbis-Stokes, P. F. O'Meara, W. Mugo, G. M. Simiyu and M. A. Deshusses, Environ. Eng. Sci., 2016, 33, 898–906 CrossRef CAS PubMed; M. Daelman, E. van Voorthuizen, U. van Dongen, E. Volcke and M. van Loosdrecht, Water Res., 2012, 46, 3657–3670 CrossRef PubMed.
- M. A. Massoud, A. Tarhini and J. A. Nasr, J. Environ. Manage., 2009, 90, 652–659 CrossRef PubMed.
- G. Libralato, A. Volpi Ghirardin and F. Avezzù, J. Environ. Manage., 2012, 94, 61S–68S CrossRef PubMed.
- E. J. Lee, C. S. Criddle, P. Bobel and D. L. Freyberg, Environ. Sci. Technol., 2013, 47, 10762–10770 CrossRef CAS PubMed.
- J. R. Mihelcic, Z. J. Ren, P. K. Cornejo, A. Fisher, A. J. Simon, S. W. Snyder, Q. Zhang, D. Rosso, T. M. Huggins, W. Cooper, J. Moeller, B. Rose, B. L. Schottel and J. Turgeon, Environ. Sci. Technol., 2017, 51, 7749–7758 CrossRef CAS PubMed.
- G. Mannina, G. Ekama, D. Caniani, A. Cosenza, G. Esposito, R. Gori, M. Garrido-Baserba, D. Rosso and G. Olsson, Sci. Total Environ., 2016, 551–552, 254–270 CrossRef CAS PubMed.
- Environmental Protection Agency
(EPA), http://www3.epa.gov/climatechange/ghgemissions/gases/ch4.html (accessed September 2017).
- J. P. Graham and M. L. Polizzotto, Environ. Health Perspect., 2013, 121, 521–530 CrossRef PubMed.
-
M. Doorn, S. Towprayoon, S. Vierira, W. Irving, C. Palmer, R. Pipatti and C. Wang, 2006 IPCC Guidelines for National Greenhouse Gas Inventories, Chapter 6: Wastewater Treatment and Discharge, IPCC, 2006 Search PubMed.
- S. Bruun, L. S. Jensen, T. K. V. Vu and S. G. Sommer, Renewable Sustainable Energy Rev., 2014, 33, 736–741 CrossRef.
- N. D. Manser, I. Wald, S. J. Ergas, R. Izurieta and J. R. Mihelcic, Environ. Sci. Technol., 2015, 49, 3128–3135 CrossRef CAS PubMed.
-
United Nations Environmental Programme (UNEP), Towards a Green Economy: Pathways to Sustainable Development and Poverty Eradication, St-Martin-Bellevue, 2011 Search PubMed.
-
UN-Habitat, Meeting Development Goals in Small Urban Centres: Water and Sanitation in the World's Cities, London, 2006 Search PubMed.
-
M. Libhaber and Á. Orozco-Jaramillo, Sustainable Treatment and Reuse of Municipal Wastewater: For Decision Makers and Practicing Engineers, IWA Publishing, London, UK, 2012 Search PubMed.
- P. H. Dodane, M. Mbeguere, S. Ousmane and L. Strande, Environ. Sci. Technol., 2012, 46, 3705–3711 CrossRef CAS PubMed.
|
This journal is © The Royal Society of Chemistry 2018 |