DOI:
10.1039/C8EN00156A
(Frontier)
Environ. Sci.: Nano, 2018,
5, 1078-1089
Emerging investigator series: the rise of nano-enabled photothermal materials for water evaporation and clean water production by sunlight
Received
5th February 2018
, Accepted 19th March 2018
First published on 5th April 2018
Abstract
Solar driven water evaporation and distillation is an ancient technology, but has been rejuvenated by nano-enabled photothermal materials in the past 4 years. The nano-enabled state-of-the-art photothermal materials are able to harvest a full solar spectrum and convert it to heat with extremely high efficiency. Moreover, photothermal structures with heat loss management have evolved in parallel. These together have led to the steadily and significantly improved energy efficiency of solar evaporation and distillation in the past 4 years. Some unprecedented clean water production rates have been reported in small-scale and fully solar-driven devices. This frontier presents a timely and systematic review of the impressive developments in photothermal nanomaterial discovery, selection, optimization, and photothermal structural designs along with their applications especially in clean water production. The current challenges and future perspectives are provided. This article helps inspire more research efforts from environmental nano communities to push forward practical solar-driven clean water production.
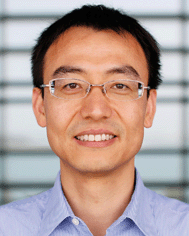 Peng Wang | Professor Peng Wang is currently an associate professor in the Environmental Science and Engineering program at KAUST. He received his Ph.D. degree from the University of California, Santa Barbara (UCSB) in 2008. His research focuses on rational design, synthesis, and application of nanomaterials toward sustainable-energy driven clean water production. |
Environmental significance
Given the vast abundance and inexhaustibility of sunlight, tapping into solar energy to produce clean water is a viable solution to current global challenges of water scarcity and clean energy shortage. Nano-enabled solar driven water evaporation and distillation have experienced very significant growth in the past 4 years. The CO2 free clean water production feature of solar evaporation and distillation makes them promising and attractive for small-scale water plants and point-of-use water supply systems. This article highlights recent developments and emerging applications of these solar-driven processes.
|
Introduction
Photothermal materials are able to effectively capture light and efficiently convert light energy to heat.1,2 The generated heat can be utilized for many kinds of applications, such as photothermal therapy,1 solar electricity generation,3 clean water production,4–6etc. Solar distillation is an ancient technology for solar driven clean water production.7 In a typical solar distillation apparatus, sunlight is captured and converted to heat by photothermal materials and the heat is used to generate water vapor, which is subsequently condensed to produce fresh water.8 Solar-driven water evaporation assisted by photothermal materials is an essentially integral part of solar distillation.9
The research on utilizing photothermal materials for both solar water evaporation and solar distillation has attracted a fast growing interest in the past 4 years.4–6 In particular, the zero CO2 emission feature of solar distillation towards seawater desalination is very contemporarily appealing and relevant in the times of ongoing and ever worsening global warming, clean energy shortage, and water scarcity.10–13
Great progress has been made in the design and fabrication of advanced photothermal materials for solar water evaporation and distillation mainly thanks to the emergence of many nano-enabled new materials, such as plasmonic metal nanoparticles, graphene oxide (GO), and reduced graphene oxide (rGO).4–6 The newly emerged photothermal nanomaterials have made the old concept of solar distillation resurface and become a rejuvenated green technology for clean water production.4–6
This frontier review highlights impressive developments made especially in the past 4 years in photothermal material selection, optimization, and photothermal structural designs. The applications of these new materials and structural designs in solar water evaporation and distillation are reviewed. The current challenges and future perspectives are discussed.
Nano as an enabler for photothermal materials utilizing sunlight
The solar spectrum at sea level ranges from 280 to 2500 nm.14 In order to capture solar energy to a great extent, a good photothermal material for solar water evaporation and distillation is expected to absorb widely within the entire solar spectrum. The development of effective photothermal materials has been a very active playground for nanomaterials in the past 4 years. The role of nano as an enabler for the rise of photothermal materials utilizing solar energy can be reflected in the following broad aspects.4–6,15
Firstly, almost all of, especially inorganic, photothermal materials have a refractive index greater than 2 (https://refractiveindex.info/), leading to their high light reflection (>11%), according to the Fresnel equation.16 To diminish the reflected light loss, the photothermal materials are typically made into highly porous nanostructures, which results in an extremely low effective refractive index and therefore achieves a much lower light reflection.17 From a microscopic point of view, the highly porous structures can create multiple reflections inside the pores (Fig. 1) and thus increase light absorption.16,17
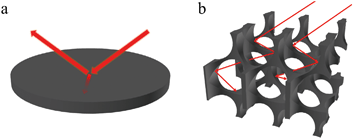 |
| Fig. 1 Comparison of (a) a flat surface and (b) a porous structure in terms of their light reflection and dependence on the incident light angle. | |
Secondly, light absorption of a flat surface has a very strong dependence on the incident light angle, while porous nanostructures of the same material show a reduced dependence degree, which is beneficial for solar photothermal materials (Fig. 1).16 It has been reported that the light absorption of hierarchical graphene foam,18 cauliflower-shaped hierarchical copper nanostructures,19 and metal nanoparticle decorated wood20 exhibited much lower sensitivity to the incident angle of sunlight.
Last but not least, the plasmonic effect of noble metal nanomaterials is wavelength specific and highly dependent on their shapes and sizes.21 To harvest a wide solar spectrum, metal nanoparticles with a wide size distribution and/or various shapes are combined in some works.22,23
Development of solar photothermal nanomaterials in the past 4 years
The first and foremost important parameter for photothermal materials in solar water evaporation and distillation is their light absorption capability. In comparison, the light-to-heat conversion performance of photothermal materials receives much less attention, not due to the lack of efforts but because most photothermal materials typically possess very high light-to-heat efficiency. In 2017, Wang et al. compared the light-to-heat conversion efficiency of MXene and carbon nanotubes (CNTs) and found no discernible difference, with both being close to 100%.24 This result agreed with the 100% light-to-heat conversion of Ti2O3 reported by Wu et al.25 and of Au nanoparticles reported by Govorov et al.26
Various nanomaterials with strong light absorbance in the wide solar spectrum have been intensively investigated in the past 4 years, including carbon based materials, metals, metal oxides, and polymers, among others. Broadly speaking, the light, once absorbed by a photothermal material, induces an electric field which drives mobile carriers inside the crystals of the material, and the energy gained by the carriers turns into heat.4
Carbon based materials
Due to the closely spaced energy levels of the loosely-held π electrons in carbon-based materials, they absorb widely in the entire solar spectrum and the excited electrons relax to their ground state, releasing heat. Carbon-based materials, including carbon black, CNTs, graphene, GO, rGO, etc. are among the most well-known photothermal materials because of their broad light absorption, high stability, light weight and low cost. Carbon-based materials contain no toxic metals, possess much better stability under sunlight than polymers, and can be easily made into all kinds of structures with macro-, micro- and nanoscale morphologies desirable for solar thermal applications.5,18,27–37
In 2011, Wang et al. reported a self-assembled Fe3O4/carbon nanocomposite capable of self-floating on top of a water surface for solar water evaporation.38 In 2014 and 2016, amorphous and low-density hollow carbon spheres with a self-floating property were similarly synthesized from organic polymer precursors for solar water evaporation.39,40 In 2015, Jiang et al. used PDMS as a binder to coat carbon black on a cotton gauze and obtained a superhydrophobic gauze structure with self-floating and self-cleaning properties for solar steam generation.41
Making porous structures has been an effective strategy to increase the light absorption of many carbon materials, among which, highly porous graphene and rGO have been popularly explored.30,31,35,37 Moreover, carbon materials possess quite high thermal conductivity, which undesirably leads to a fast heat conduction loss to bulk water. In 2015, Ito et al. found that by doping nitrogen, the thermal conductivity of a graphene membrane could be significantly decreased, which led to a higher solar evaporation energy efficiency (80%) compared to the undoped graphene sample (67%).42 In 2016, Ghasemi et al. fabricated a graphite flake coated porous PDMS foam to reduce heat loss to bulk water.43 In 2017, an rGO/polyurethane composite foam was reported following the same idea.44
In 2014, Chen et al. first reported a bi-layered structure of an exfoliated graphite layer on top of a carbon foam.45 The bottom layers served as an insulating heat barrier to improve the solar evaporation efficiency and at the same time as a support to improve the mechanical properties. Many similar bi-layered rGO and CNT based photothermal structures have since been synthesized with the bottom heat barrier materials being air-laden papers,46 bacterial nanocellulose aerogel,47 polystyrene foam,32,48,49 a mixed cellulose ester membrane,33 PDMS foam,50 a macroporous silica substrate,51 wood block,52,53 or a porous poly(vinylidene fluoride) (PVDF) membrane.36
Surface carbonized wood block is another attractive photothermal material because wood is widely available, biodegradable, has excellent thermal insulation properties and at the same time has abundant water channel networks for fast water transport.54–58 Also reported were other carbon based photothermal materials, such as carbonized mushrooms,59 microporous coke synthesized from zeolite templates,34 a 3D printed CNT/GO/nanofibrous cellulose composite with a box structure.60
Metals
Many metals are known to have strong plasmon resonance. When the frequency of incident light matches the oscillation frequency of delocalized electrons in the metals, it triggers a collective excitation of the electrons, generating hot electrons. The hot electrons oscillate coherently with the incident electromagnetic field, resulting in heat generation by a Joule mechanism.4,6,61 Gold (Au) distinguishes itself among its peers due to its high tunability of light adsorption within the solar spectrum, chemical stability, availability of rich synthesis methods, and nontoxicity.21,62
In 2013 and 2014, Halas's group pioneered Au nanoparticle-enabled solar vapor generation which inspired wide research interest in solar water evaporation and beyond.63–65 Deng et al. fabricated films of Au nanoparticles that could self-float at the air–water interface in 2014 (ref. 66) and further produced free-standing photothermal films by depositing Au nanoparticles onto air-laden paper67 and AAO.68,69 With these self-floating photothermal films, localized heating occurred only at the air–water interface, leading to an improved water evaporation efficiency.
Given the inherently narrow absorption bandwidth of well-sorted Au nanoparticles, in 2015, Kim et al. employed adiabatic plasmonic nanofocusing to attain ultra-broadband light absorption and flexible thin-film black Au membranes, which absorbed 91% at 400–2500 nm and showed effective solar water evaporation.70 In 2016, Singamaneni and coworkers demonstrated the efficacy of a Au nanorod loaded biomaterial derived 3D aerogel for solar water evaporation.71
To further expand the absorption of solar radiation, in 2017, Zhu et al. loaded Au nanoparticles with random sizes and distributions into an AAO template and achieved an absorbance of ∼99% across wavelengths from 400 nm to 10 μm, which resulted in an water evaporation efficiency of 90% under 4-sun intensity (4 kW m−2).22 By coating a 12 nm Al2O3 layer onto the surface of Au nanoparticles, the thermal stability of the Au nanoparticles was increased to 1073 K.72 In 2017, Han and Fratalocchi developed a dark metasurface by dispersing special gold dimers of nanorods connected with nanospheres on a filter paper, which exhibited efficient solar evaporation.73 Hu et al. coated plasmonic Pd, Au and Ag nanoparticles in the pores of natural wood block, which exhibited a high light absorption ability (≈99%) over a broad wavelength range from 200 to 2500 nm and a high solar conversion efficiency of 85% under 10 sun illumination with superior stability longer than 144 h.20
Other plasmonic metal nanomaterials have also been investigated towards solar evaporation and distillation. In 2016, Zhu et al. in situ synthesized self-assembled aluminum (Al) nanoparticles in a AAO membrane and the modified membrane absorbed a broad solar spectrum (>96%), leading to an improved seawater desalination performance.23 In 2017, Ozin et al. reported the size-dependent photothermal effect of germanium (Ge) nanocrystals and pointed out their potential application to solar desalination.74 In 2016, He et al. studied photothermal effects of silver (Ag) and Ag–Au blended plasmonic nanoparticles.75
Furthermore, bimetallic nanocomposites with rationally designed nanostructures have shown some benefits in light harvesting. In 2016, Ag/Au bimetallic hollow mesoporous plasmonic nanoshells were fabricated which yielded a higher solar evaporation rate compared with their solid-core counterparts.76
Metal oxide/mixed metal oxides (MMOs)
In metal oxide type of semiconductors, light is absorbed to generate electron–hole pairs. Solar light, which has a higher energy than the bandgap of the semiconductors, would lead to the creation of above-bandgap electron–hole pairs, which would then relax to the band edges and convert the extra energy into heat. The electron–hole pairs in narrow-bandgap semiconductors would ultimately recombine to give rise to heat generation.77
In 2016, Huang et al. synthesized titania nanocages with a high light trapping capability for solar water evaporation.78 In 2017, TiOx (x < 2) and Ti2O3 nanoparticles with strong light absorption were reported and their application to solar evaporation and desalination was demonstrated.25,79 In 2017, oxygen-deficient molybdenum oxide (MoO3) quantum dots, which possessed a matching-absorption-spectrum to solar light in both visible and near infrared regions, were used for solar water evaporation.80 In 2017, Ye et al. prepared Fe3O4, MnFe2O4, ZnFe2O4, and CoFe2O4 hydrophobic microspheres, which self-assembled into self-floating thin films for solar thermal evaporation.81 In 2018, Wang et al. reported a CuCr2O4 loaded quartz glass fiber membrane as an extremely thermal stable solar photothermal material which provided potential fouling control for practical solar evaporation and distillation applications.82
Other inorganic nanomaterials
Wang et al. investigated the solar driven heating performance of PbS nanoparticles uniformly dispersed in water in 2013.83 In 2016, Nagao et al. reported that the lossy plasmonic resonances of titanium nitride (TiN) nanoparticles were broad enough to cover and effectively absorb the solar spectrum and the TiN nanoparticles, when dispersed in water, showed better performance than Au and carbon black nanoparticles in solar water evaporation.84 In 2017, Wang et al. first reported MXene (Ti3C2) as a full solar spectrum absorber and effective photothermal material for solar water evaporation.24 Synthesis of hierarchical graphdiyne-based architecture was reported in 2017 for solar thermal evaporation.85 In 2016, the assembled Cu7S4 nanocrystal dense film,86 and in 2017, porous floating copper phosphate combined with PDMS87 were separately reported as photothermal materials for solar-driven water evaporation.
Polymers
Polymers provide flexibility and easy moldability that their inorganic counterparts cannot. However, compared with rich inorganic photothermal materials, the choices of stable and water compatible photothermal polymers working within a broad solar spectrum are very limited. In 2015, polypyrrole (PPY), a conjugated conductive polymer, was coated onto a metal mesh using a simple anodization method and the PPY coated mesh showed satisfactory performances in solar water evaporation88 and desalination.89 In 2017, polydopamine was introduced as a low-cost and biodegradable photothermal material and has since been used for solar water evaporation and seawater desalination.90,91 In 2018, Wei and co-workers synthesized a new solar photothermal polymer (poly(1,3,5-hexahydro-1,3,5-triazine), which could be easily solution processed into a self-floating monolithic and single component foam).92 The photothermal foams were mechanically strong, thermally stable to 300 °C and chemically resistant to organic solvents.
Considering their cost, availability, stability, and toxicity,28 carbon based photothermal materials are largely favorable choices and have been more widely explored in solar evaporation and distillation applications than the others. Some comparative studies have hinted that more expensive Au nanocrystals and MXene had no clearly convincing advantages over relatively inexpensive carbon black and/or CNTs in terms of solar photothermal conversion efficiency.83 It has to be pointed out that the purpose of this Frontier is to provide a concise overview of the state-of-the-art of this exciting field; more detailed and comprehensive summaries of photothermal materials, including their types, light-to-heat conversion mechanisms, light absorbing characteristics, etc. can be found in some recent review articles.4–6
Development of photothermal structures utilizing sunlight
In a conventional bulk heating solar distillation apparatus, bulk water is heated to a high temperature (50–90 °C) for water vapor generation, which results in a slow response to sunlight and a bothersome heat management of using an elaborately installed thermal insulation material over the entire bulk water part.8,93,94 In the past 4 years, the interfacial heating idea has been widely accepted and utilized in the design of advanced solar distillation apparatuses.4–6 With an interfacial heating scheme, there is an ultra-fast response to sunlight and the generated heat is mainly confined in the surface water layer, which leaves the bulk water with a much lower temperature and therefore greatly removes the burden of the thermal insulation need of the bulk water body.
Fig. 2 presents the energy balance scheme in a typical interfacial solar water evaporation system, with a photothermal material floating at the air–water interface. In such a system, solar radiation (Psun) is the only energy input which is ultimately split into and is balanced by five energy output pathways: (1) reflective solar radiation (Prf), (2) water evaporation (Peva), including sensible heat and latent heat of the evaporating water, (3) radiative heat loss from the photothermal material/water interface (Prd), (4) convective and conduction heat loss to the overlying air (Pcv), and (5) heat conduction loss to the underlying bulk water (Pcd).
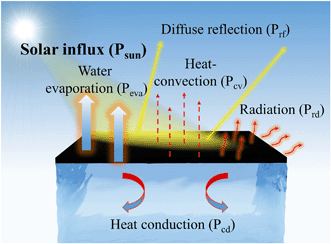 |
| Fig. 2 Energy balance diagram of a solar water evaporation system based on interfacial heating. Note: water evaporation is in open air in this case. | |
The development of photothermal materials and evolution of photothermal structural designs in the past 4 years have been guided by the energy diagram in Fig. 2. Efforts have been focused on minimizing wasteful energy loss (Prf + Pcv + Pcd + Prd) other than water evaporation (Peva) so as to enhance the water evaporation rate, i.e. to increase the solar thermal water evaporation efficiency.
Bulk heating
Halas and co-workers initiated Au nanoparticle-based solar driven water evaporation.63–65 In their work, a configuration of dispersing Au nanoparticles uniformly in bulk water was used. This configuration (Fig. 3a), due to its bulk water heating scheme, delivers unsatisfactory water evaporation efficiency under regular and unconcentrated solar irradiation, although its energy efficiency under concentrated light can be higher. Chen et al. reported a water evaporation efficiency of 69% under 10 sun using graphitized carbon black, carbon black, and graphene dispersed in water.95 Deng et al. enhanced the water evaporation efficiency by dispersing Au nanoparticles along with light scattering polystyrene nanoparticles in bulk water, leading to 35.6% energy efficiency under the illumination of 532 nm laser light with a power density of 35.36
W cm−2.96
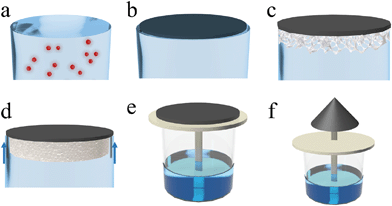 |
| Fig. 3 Evolution of photothermal structures for solar evaporation. (a) Dispersed nanoparticles for bulk water heating; self-floating 2D photothermal materials (b) without a heat barrier, (c) with a macroporous heat barrier, and (d) with a nonporous heat barrier and 2D water channels; (e) 2D and (f) 3D photothermal materials with 1D water channel. | |
Interfacial heating
As opposed to bulk water heating, the interfacial heating concept was started in the early 2010s and has since been a popular approach for solar water evaporation (Fig. 3b–f). In 2011, Wang et al. reported a Fe3O4/carbon composite material self-floating on top of a water surface, which is one of the pioneering works that introduced interfacial heating in solar thermal applications.38 Later, there were examples of Au films,66 carbon black-based superhydrophobic gauze,41 PPY coated mesh,88 self-assembled black titania films,78 self-assembled magnetic MMO microsphere films,81etc. Given the localized and selective heating only at the air–water interface where water evaporation takes place, these interfacial heating systems deliver better energy efficiency than the dispersion configuration under otherwise the same conditions especially under non-concentrated solar irradiation.
However, due to the presence of a high temperature zone at the air–water interface in the interfacial heating systems, the heat conduction loss (Pcd) from the photothermal materials into the underlying bulk water becomes an issue (Fig. 3b), which prompted the use of thermally insulating heat barriers to separate the photothermal layer and the bulk water. In 2014, Chen et al. first proposed and prepared a bi-layered structure with the bottom layer of carbon foam being a deliberately designed heat barrier.45 In 2015, Deng et al. reported a heat barrier of an air-laden paper that supported a self-assembled Au film right on top of it.67 In addition, the bottom layer acts as a substrate to improve the mechanical properties of the photothermal material. Thereafter, this bi-layered structure has been widely adopted due to the improvement in energy efficiency and mechanical stability.4–6
In some cases, the heat barrier was deliberately designed to be macroporous so as to include in one material both functions of the heat barrier and water transport channels to supply water to the evaporation surfaces (Fig. 3c).51 However, this design was later found imperfect as the water inside the macropores greatly increased the material's thermal conduction and thus compromised its role as a heat barrier.32
In 2017, Zhu et al. and Wang et al. reported 2-dimensional (2D) water channels.32,48 In this design, the 2D water channels were placed outside of the single-function heat barrier of a polystyrene foam with close pores (Fig. 3d) and the new configuration led to an improved energy efficiency of 83–85%. Later, a 1D water channel design was reported in the literature using the capillary effect in porous substrates (Fig. 3e),97 which has since been employed by others.29 In both 2D and 1D water channel configurations, the heat loss due to heat conduction to bulk water can be nearly eliminated.
In the interfacial heating scheme using a floating configuration, the thermal radiation (Prd) is quantified by the Stefan–Boltzmann equation:
where
Φ denotes the radiation heat flux,
ε and
A are the emissivity and surface area of the water evaporation surface, respectively,
σ is the Stefan–Boltzmann constant,
T1 is the temperature of the water evaporation surface, and
T2 is the ambient temperature. Therefore, reducing the water evaporation temperature by making 3D photothermal structures is a rational solution, which increases the water evaporation surface area with the same level of incoming solar intensity. The 3D umbrella structure by Zhu
et al. (
Fig. 3f) is the only example so far,
97 although more 3D designs are expected to come.
One noteworthy work in the structural design for solar evaporation is that of Chen et al. who, in 2016, demonstrated a low-cost and scalable self-floating solar evaporation device capable of generating 100 °C water steam under ambient air conditions without optical concentration.98 The high temperatures were achieved by using thermal concentration and by carefully reducing Prf, Pcv, Pcd, and Prd. The generation of high temperature water vapor under unconcentrated sunlight has the potential to expand the application scope of solar thermal conversion.
Applications of solar distillation and evaporation
Solar distillation
There have been considerable efforts to conduct solar distillation using bench-scale simple solar still devices with interfacial heating design. In these devices, a photothermal material with satisfactory sunlight absorption capability floats on the source water surface and a transparent cover (e.g., glass, plastic) serves as the water condensation surface (Fig. 4a). These solar distillation devices are all able to produce potable water with good quality utilizing a variety of source waters, including synthetic seawater,36,37,48,50,67,92 real seawater,35,55,99 lab-prepared wastewater,46,68,97 river water,49 lake water,100 and sewage water.37 Nevertheless, the water production rates of these devices represented only a small improvement relative to the ancient design of a solar still given the dilemma of both transparency and thermal conductivity of the condensation surface in this old-fashioned design.
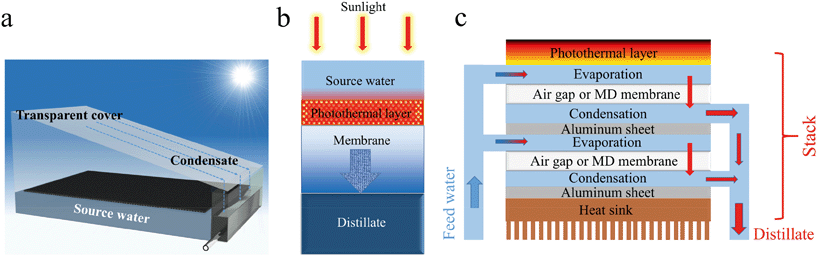 |
| Fig. 4 (a) Simple solar distillation apparatus based on interfacial heating, (b) conceptual design of solar-thermal interfacial heating in MD, and (c) a solar distillation design with a latent heat recovery mechanism. | |
Combination of solar-driven interfacial heating with MD
Membrane distillation (MD) is a thermally-driven separation process, in which only vapor molecules transfer through a microporous hydrophobic membrane. The driving force in the MD process is the vapor pressure difference induced by the temperature difference across the hydrophobic membrane.101 A conventional MD involves heating up the entire bulk water to a uniform temperature and suffers from interfacial temperature polarization due to the removal of latent heat by water evaporation at the MD membrane.102 The interfacial temperature polarization decreases the membrane temperature and thus diminishes the net driving force to mass transport water across the MD membrane.101 The solar-thermal interfacial heating concept has been creatively combined with the MD process to alleviate the aforementioned drawbacks (Fig. 4b). Interfacial heating generates localized heating only on the MD membrane interface and thus eliminates the requirement of heating the entire bulk feed water and at the same time reduces the impact of interfacial temperature polarization.103–107
From 2015 to 2017, Fujiwara together with coworkers demonstrated azobenzene dye loaded AAO and PTFE membranes for light driven seawater desalination.108–110 In 2017, Curcio et al. fabricated PVDF MD membranes filled with spherical silver nanoparticles with controlled sizes.103 Under the illumination of UV light at 366 nm provided by a high pressure UV mercury lamp of 500 W, the membrane surface temperature was 23 K greater than the bulk water, which resulted in an 11 fold increase of the transmembrane water flux, with 0.5 M NaCl being the feed water. In the above designs, the photothermal species/materials were almost uniformly imbedded throughout the pores of the membranes.
In 2017, Li et al. reported a carbon black nanoparticle or SiO2/Au nanoshell coated MD membrane, which delivered an up to 33% increase in distillate flux when irradiated at 1 sun.104 Later, Li along with Halas and coworkers fabricated a bi-layered MD membrane structure, consisting of a thin and carbon black nanoparticle impregnated electrospun polyvinyl alcohol (PVA) porous film stacked on a commercial PVDF MD membrane.12,106 The two designs by Li et al.104,106 had the photothermal materials only on one side of the MD membranes. The nanophotonics-enabled solar membrane distillation (NESMD) system was directly and entirely driven by solar energy. A pilot-scale device with an active area of ∼1 × 1 m, when tested outdoor locally at Houston, USA, was able to produce an average clean water flux of 0.5 kg m−2 h, equivalent to ∼4 L per day under less than 1 sun (700 W m−2) illumination for 8 h of sunlight in summer.
In the same year, Chiavazzo and Asinari along with co-workers reported a solar-driven and fully passive distiller, which could be operated in either MD mode or without a membrane.105 In their design, two major features were noteworthy: multiple cycles of latent-heat recovery and separation of the light-adsorption surface and water evaporation surface (Fig. 4c), both contributing to considerably enhanced energy efficiency. The latent heat from water vapor condensation was collected and recycled back repeatedly to the feed water to drive further water evaporation. The small device, when tested outdoors at Torino, Italy in summer and with real seawater as feed water, produced an unprecedented clean water flux of 1.77 kg kW h−1, equivalent to 1.24 kg m−2 h under 700 W m−2 solar illumination which exceeds the thermodynamic limit of single-stage solar stills.
Applications based on solar water evaporation
Some applications based on water removal by solar evaporation have emerged, including salt extraction towards zero liquid discharge desalination, wastewater volume reduction, and salt or heavy metal extraction. In 2017, Mi et al. used a GO thin film to evaporate 15 wt% NaCl solution, during which salt crystals accumulated on the GO film surface.29 The results pointed to the potential application of solar-thermal RO brine treatment toward a zero liquid discharge desalination process.29 In 2017, Zhu et al. used a 3D photothermal structure and extracted Cu and Au salts out of contaminated water by a solar water evaporation process.97
Other emerging applications
There have also been examples of integrating the solar photothermal process along with the energy generation and transformation process, including photocatalysis and electricity generation. In 2016, a bifunctional membrane consisting of a top layer of TiO2 nanoparticles, a middle layer of Au nanoparticles, and a bottom layer of AAO was designed for simultaneous photocatalytic degradation of water contaminants and solar-driven evaporation.68 In 2017, the salt concentration gradient across the water evaporation surface and the bulk water was utilized by Zhou et al. who provided the first proof-of-concept of simultaneous solar thermal desalination and electricity generation under natural sunlight illumination.111 In 2017, a solar thermal collector based on SiO2/Ag@TiO2 core–shell nanocomposites was reported by Ho et al. for synergistic photothermic driven seawater catalysis to generate hydrogen and to produce desalted water under natural sunlight.112 In 2018, Ho et al. reported simultaneous solar distillation and electricity generation by self-contained monolithic carbon sponges.113 In 2017, Kim et al. used broad light absorbing Au and carbon black and demonstrated their direct solar thermal disinfection effect.62
Challenges and future perspectives
In spite of the amazing achievements made in the past 4 years in photothermal materials, their applications are still at an infant stage and there are significant barriers standing between the status quo and large scale and practical applications of solar evaporation and solar distillation. The following are some of the major challenges, which call for much needed research efforts.
Challenges
(1) There has been no field-accepted guideline on performance evaluation in solar evaporation or distillation research. A review of the literature reveals a large variability in experimental conditions as well as in energy efficiency calculations, which makes it unreliable to directly compare various photothermal materials just by the literature values of their solar energy efficiency and water evaporation rates. (2) The long-term efficacy of many photothermal materials and designs in dealing with realistic waters, such as real seawater, groundwater, river water, and industrial wastewater, has not been confirmed. Dissolved salts, such as NaCl, CaSO4, and MgSO4, in seawater and hypersaline waters, would crystalize during solar evaporation and distillation and their crystallization behaviors are largely unknown in these solar-driven processes. In particular, the influence of salt fouling of the photothermal materials caused by Ca2+, Mg2+ and other insoluble or sparsely soluble matter has not been investigated. Equally importantly, bio-fouling of the photothermal materials resulting from dissolved organic matter and other bio species present in source waters would be expected, but has been paid little attention. (3) Many impaired source waters contain volatile organic compounds (VOCs), which would evaporate along and be collected together with water during solar distillation. The concentration of the VOCs in the collected distillate may even be enriched by distillation, which is the Achilles' heel of solar distillation and needs to be addressed by more advanced and multifunctional photothermal designs. (4) The intermittent nature of solar light has a profound effect on solar evaporation and distillation systems, but has not been looked at thoroughly enough.
Future perspectives
From the solar distillation perspective, it does not need any moving parts, electronic devices and high pressure operations, all of which make it attractive and economical especially for small sized water plants, off-grid and point-of-use (POU) potable clean water production apparatus.114 Moreover, solar distillation produces fresh water from a variety of water sources with impaired water quality and thus is suitable as an emergency response means for natural disaster-impacted, war-torn regions, as well as the sheer volume of impoverished people who lacks access to clean drinking water. Solar evaporation assisted by photothermal materials towards brine or hypersaline water treatment for the purpose of zero-liquid discharge and/or salt extraction is expected to grow as regulations on direct brine discharge are being tightened globally. In both solar evaporation and distillation applications, device design and fabrication seem more important than finding new photothermal materials unless the new materials provide other new prospects in practical applications, such as easy recycle, thermal stability, robustness, flexibility, longevity, etc. In solar distillation devices, more research efforts are highly warranted for water condensation and collection sides and more effective recovery of the latent heat of water condensation will boost their energy efficiency significantly. The future application scope of both solar evaporation and solar distillation is expected to widen in the years to come.
Conflicts of interest
There are no conflicts to declare.
Acknowledgements
The author is grateful to KAUST for the very generous financial support, to Renyuan Li for illustrations and to other group members for discussions.
References
- D. Jaque, L. M. Maestro, B. del Rosal, P. Haro-Gonzalez, A. Benayas, J. L. Plaza, E. M. Rodriguez and J. G. Sole, Nanoparticles for photothermal therapies, Nanoscale, 2014, 6, 9494–9530 RSC.
- P. K. Jain, X. Huang, I. H. El-Sayed and M. A. El-Sayed, Noble Metals on the Nanoscale: Optical and Photothermal Properties and Some Applications in Imaging, Sensing, Biology, and Medicine, Acc. Chem. Res., 2008, 41, 1578–1586 CrossRef CAS PubMed.
- F. Cao, K. McEnaney, G. Chen and Z. F. Ren, A review of cermet-based spectrally selective solar absorbers, Energy Environ. Sci., 2014, 7, 1615–1627 CAS.
- G. H. Liu, J. L. Xu and K. Y. Wang, Solar water evaporation by black photothermal sheets, Nano Energy, 2017, 41, 269–284 CrossRef CAS.
- V. D. Dao and H. S. Choi, Carbon-Based Sunlight Absorbers in Solar-Driven Steam Generation Devices, Global Chall., 2018, 2(2), 1700094 CrossRef.
- Z. Y. Deng, J. H. Zhou, L. Miao, C. Y. Liu, Y. Peng, L. X. Sun and S. Tanemura, The emergence of solar thermal utilization: solar-driven steam generation, J. Mater. Chem. A, 2017, 5, 7691–7709 CAS.
- E. Delyannis, Historic background of desalination and renewable energies, Sol. Energy, 2003, 75, 357–366 CrossRef CAS.
- A. E. Kabeel and S. A. El-Agouz, Review of researches and developments on solar stills, Desalination, 2011, 276, 1–12 CrossRef CAS.
- A. F. Muftah, M. A. Alghoul, A. Fudholi, M. M. Abdul-Majeed and K. Sopian, Factors affecting basin type solar still productivity: A detailed review, Renewable Sustainable Energy Rev., 2014, 32, 430–447 CrossRef.
- H. Sharon and K. S. Reddy, A review of solar energy driven desalination technologies, Renewable Sustainable Energy Rev., 2015, 41, 1080–1118 CrossRef CAS.
- S. M. Rodrigues, P. Demokritou, N. Dokoozlian, C. O. Hendren, B. Karn, M. S. Mauter, O. A. Sadik, M. Safarpour, J. M. Unrine, J. Viers, P. Welle, J. C. White, M. R. Wiesner and G. V. Lowry, Nanotechnology for sustainable food production: promising opportunities and scientific challenges, Environ. Sci.: Nano, 2017, 4, 767–781 RSC.
- P. Westerhoff, P. Alvarez, Q. Li, J. Gardea-Torresdey and J. Zimmerman, Overcoming implementation barriers for nanotechnology in drinking water treatment, Environ. Sci.: Nano, 2016, 3, 1241–1253 RSC.
- W. Wang, G. Li, D. Xia, T. An, H. Zhao and P. K. Wong, Photocatalytic nanomaterials for solar-driven bacterial inactivation: recent progress and challenges, Environ. Sci.: Nano, 2017, 4, 782–799 RSC.
- C. A. Gueymard, The sun's total and spectral irradiance for solar energy applications and solar radiation models, Sol. Energy, 2004, 76, 423–453 CrossRef.
- J. Chang, L. Zhang and P. Wang, Intelligent Environmental Nanomaterials, Environ. Sci.: Nano, 2018 10.1039/C7EN00760D.
- M. K. Hedayati and M. Elbahri, Antireflective Coatings: Conventional Stacking Layers and Ultrathin Plasmonic Metasurfaces, A Mini-Review, Materials, 2016, 9, 22 CrossRef PubMed.
- J. G. Cai and L. M. Qi, Recent advances in antireflective surfaces based on nanostructure arrays, Mater. Horiz., 2015, 2, 37–53 RSC.
- H. Ren, M. Tang, B. Guan, K. Wang, J. Yang, F. Wang, M. Wang, J. Shan, Z. Chen, D. Wei, H. Peng and Z. Liu, Hierarchical Graphene Foam for Efficient Omnidirectional Solar–Thermal Energy Conversion, Adv. Mater., 2017, 29, 1702590 CrossRef PubMed.
- P. X. Fan, H. Wu, M. L. Zhong, H. J. Zhang, B. F. Bai and G. F. Jin, Large-scale cauliflower-shaped hierarchical copper nanostructures for efficient photothermal conversion, Nanoscale, 2016, 8, 14617–14624 RSC.
- M. Zhu, Y. Li, F. Chen, X. Zhu, J. Dai, Y. Li, Z. Yang, X. Yan, J. Song, Y. Wang, E. Hitz, W. Luo, M. Lu, B. Yang and L. Hu, Plasmonic Wood for High-Efficiency Solar Steam Generation, Adv. Energy Mater., 2018, 8(4), 1701028 CrossRef.
- H. J. Chen, L. Shao, Q. Li and J. F. Wang, Gold nanorods and their plasmonic properties, Chem. Soc. Rev., 2013, 42, 2679–2724 RSC.
- L. Zhou, Y. L. Tan, D. X. Ji, B. Zhu, P. Zhang, J. Xu, Q. Q. Gan, Z. F. Yu and J. Zhu, Self-assembly of highly efficient, broadband plasmonic absorbers for solar steam generation, Sci. Adv., 2016, 2(4), e1501227 Search PubMed.
- L. Zhou, Y. Tan, J. Wang, W. Xu, Y. Yuan, W. Cai, S. Zhu and J. Zhu, 3D self-assembly of aluminium nanoparticles for plasmon-enhanced solar desalination, Nat. Photonics, 2016, 10, 393 CrossRef CAS.
- R. Y. Li, L. B. Zhang, L. Shi and P. Wang, MXene Ti3C2: An Effective 2D Light-to-Heat Conversion Material, ACS Nano, 2017, 11, 3752–3759 CrossRef CAS PubMed.
- J. Wang, Y. Y. Li, L. Deng, N. N. Wei, Y. K. Weng, S. Dong, D. P. Qi, J. Qiu, X. D. Chen and T. Wu, High-Performance Photothermal Conversion of Narrow-Bandgap Ti2O3 Nanoparticles, Adv. Mater., 2017, 29(3), 1603730 CrossRef PubMed.
- H. H. Richardson, M. T. Carlson, P. J. Tandler, P. Hernandez and A. O. Govorov, Experimental and Theoretical Studies of Light-to-Heat Conversion and Collective Heating Effects in Metal Nanoparticle Solutions, Nano Lett., 2009, 9, 1139–1146 CrossRef CAS PubMed.
- X. Zhu, K. Yang and B. Chen, Membranes prepared from graphene-based nanomaterials for sustainable applications: a review, Environ. Sci.: Nano, 2017, 4, 2267–2285 RSC.
- R. Bjorkland, D. A. Tobias and E. J. Petersen, Increasing evidence indicates low bioaccumulation of carbon nanotubes, Environ. Sci.: Nano, 2017, 4, 747–766 RSC.
- C. Finnerty, L. Zhang, D. L. Sedlak, K. L. Nelson and B. Mi, Synthetic Graphene Oxide Leaf for Solar Desalination with Zero Liquid Discharge, Environ. Sci. Technol., 2017, 51, 11701–11709 CrossRef CAS PubMed.
- Y. Fu, G. Wang, T. Mei, J. Li, J. Wang and X. Wang, Accessible Graphene Aerogel for Efficiently Harvesting Solar Energy, ACS Sustainable Chem. Eng., 2017, 5, 4665–4671 CrossRef CAS.
- X. Hu, W. Xu, L. Zhou, Y. Tan, Y. Wang, S. Zhu and J. Zhu, Tailoring Graphene Oxide-Based Aerogels for Efficient Solar Steam Generation under One Sun, Adv. Mater., 2017, 29, 1604031 CrossRef PubMed.
- L. Shi, Y. C. Wang, L. B. Zhang and P. Wang, Rational design of a bi-layered reduced graphene oxide film on polystyrene foam for solar-driven interfacial water evaporation, J. Mater. Chem. A, 2017, 5, 16212–16219 CAS.
- G. Wang, Y. Fu, X. F. Ma, W. B. Pi, D. W. Liu and X. B. Wang, Reusable reduced graphene oxide based double-layer system modified by polyethylenimine for solar steam generation, Carbon, 2017, 114, 117–124 CrossRef CAS.
- J. J. Wang, Z. H. Liu, X. L. Dong, C. E. Hsiung, Y. H. Zhu, L. M. Liu and Y. Han, Microporous cokes formed in zeolite catalysts enable efficient solar evaporation, J. Mater. Chem. A, 2017, 5, 6860–6865 CAS.
- P. Zhang, J. Li, L. Lv, Y. Zhao and L. Qu, Vertically Aligned Graphene Sheets Membrane for Highly Efficient Solar Thermal Generation of Clean Water, ACS Nano, 2017, 11, 5087–5093 CrossRef CAS PubMed.
- Y. Wang, C. Wang, X. Song, S. K. Megarajan and H. Jiang, A facile nanocomposite strategy to fabricate a rGO–MWCNT photothermal layer for efficient water evaporation, J. Mater. Chem. A, 2018, 6, 963–971 CAS.
- Y. Yang, R. Zhao, T. Zhang, K. Zhao, P. Xiao, Y. Ma, P. M. Ajayan, G. Shi and Y. Chen, Graphene-Based Standalone Solar Energy Converter for Water Desalination and Purification, ACS Nano, 2018, 12, 829–835 CrossRef CAS PubMed.
- Y. Zeng, J. F. Yao, B. A. Horri, K. Wang, Y. Z. Wu, D. Li and H. T. Wang, Solar evaporation enhancement using floating light-absorbing magnetic particles, Energy Environ. Sci., 2011, 4, 4074–4078 CAS.
- Y. Zeng, K. Wang, J. F. Yao and H. T. Wang, Hollow carbon beads for significant water evaporation enhancement, Chem. Eng. Sci., 2014, 116, 704–709 CrossRef CAS.
- J. G. Zhou, Z. L. Sun, M. Q. Chen, J. T. Wang, W. M. Qiao, D. H. Long and L. C. Ling, Macroscopic and Mechanically Robust Hollow Carbon Spheres with Superior Oil Adsorption and Light-to-Heat Evaporation Properties, Adv. Funct. Mater., 2016, 26, 5368–5375 CrossRef CAS.
- Y. Liu, J. Chen, D. Guo, M. Cao and L. Jiang, Floatable, Self-Cleaning, and Carbon-Black-Based Superhydrophobic Gauze for the Solar Evaporation Enhancement at the Air–Water Interface, ACS Appl. Mater. Interfaces, 2015, 7, 13645–13652 CAS.
- Y. Ito, Y. Tanabe, J. Han, T. Fujita, K. Tanigaki and M. Chen, Multifunctional Porous Graphene for High-Efficiency Steam Generation by Heat Localization, Adv. Mater., 2015, 27, 4302–4307 CrossRef CAS PubMed.
- S. M. Sajadi, N. Farokhnia, P. Irajizad, M. Hasnain and H. Ghasemi, Flexible artificially-networked structure for ambient/high pressure solar steam generation, J. Mater. Chem. A, 2016, 4, 4700–4705 CAS.
- G. Wang, Y. Fu, A. K. Guo, T. Mei, J. Y. Wang, J. H. Li and X. B. Wang, Reduced Graphene Oxide-Polyurethane Nanocomposite Foam as a Reusable Photoreceiver for Efficient Solar Steam Generation, Chem. Mater., 2017, 29, 5629–5635 CrossRef CAS.
- H. Ghasemi, G. Ni, A. M. Marconnet, J. Loomis, S. Yerci, N. Miljkovic and G. Chen, Solar steam generation by heat localization, Nat. Commun., 2014, 5, 4449 CAS.
- J. Lou, Y. Liu, Z. Wang, D. Zhao, C. Song, J. Wu, N. Dasgupta, W. Zhang, D. Zhang, P. Tao, W. Shang and T. Deng, Bioinspired Multifunctional Paper-Based rGO Composites for Solar-Driven Clean Water Generation, ACS Appl. Mater. Interfaces, 2016, 8, 14628–14636 CAS.
- Q. Jiang, L. Tian, K. K. Liu, S. Tadepalli, R. Raliya, P. Biswas, R. R. Naik and S. Singamaneni, Bilayered Biofoam for Highly Efficient Solar Steam Generation, Adv. Mater., 2016, 28, 9400–9407 CrossRef CAS PubMed.
- X. Li, W. Xu, M. Tang, L. Zhou, B. Zhu, S. Zhu and J. Zhu, Graphene oxide-based efficient and scalable solar desalination under one sun with a confined 2D water path, Proc. Natl. Acad. Sci. U. S. A., 2016, 113, 13953–13958 CrossRef CAS PubMed.
- Z. Liu, H. Song, D. Ji, C. Li, A. Cheney, Y. Liu, N. Zhang, X. Zeng, B. Chen, J. Gao, Y. Li, X. Liu, D. Aga, S. Jiang, Z. Yu and Q. Gan, Extremely Cost-Effective and Efficient Solar Vapor Generation under Nonconcentrated Illumination Using Thermally Isolated Black Paper, Global Chall, 2017, 1(2), 1600003 CrossRef PubMed.
- Z. Z. Wang, Q. X. Ye, X. B. Liang, J. L. Xu, C. Chang, C. Y. Song, W. Shang, J. B. Wu, P. Tao and T. Deng, Paper-based membranes on silicone floaters for efficient and fast solar-driven interfacial evaporation under one sun, J. Mater. Chem. A, 2017, 5, 16359–16368 CAS.
- Y. C. Wang, L. B. Zhang and P. Wang, Self-Floating Carbon Nanotube Membrane on Macroporous Silica Substrate for Highly Efficient Solar-Driven Interfacial Water Evaporation, ACS Sustainable Chem. Eng., 2016, 4, 1223–1230 CrossRef CAS.
- C. Chen, Y. Li, J. Song, Z. Yang, Y. Kuang, E. Hitz, C. Jia, A. Gong, F. Jiang, J. Y. Zhu, B. Yang, J. Xie and L. Hu, Highly Flexible and Efficient Solar Steam Generation Device, Adv. Mater., 2017, 29(3), 1701756 CrossRef PubMed.
- K. K. Liu, Q. Jiang, S. Tadepallifit, R. Raliya, P. Biswas, R. R. Naik and S. Singamaneni, Wood Graphene Oxide Composite for Highly Efficient Solar Steam Generation and Desalination, ACS Appl. Mater. Interfaces, 2017, 9, 7675–7681 CAS.
- Q. Jiang and S. Singamaneni, Water from Wood: Pouring through Pores, Joule, 2017, 1, 429–430 CrossRef.
- M. W. Zhu, Y. J. Li, G. Chen, F. Jiang, Z. Yang, X. G. Luo, Y. B. Wang, S. D. Lacey, J. Q. Dai, C. W. Wang, C. Jia, J. Y. Wan, Y. G. Yao, A. Gong, B. Yang, Z. F. Yu, S. Das and L. B. Hu, Tree-Inspired Design for High-Efficiency Water Extraction, Adv. Mater., 2017, 29(44), 1704107 CrossRef PubMed.
- G. B. Xue, K. Liu, Q. Chen, P. H. Yang, J. Li, T. P. Ding, J. J. Duan, B. Qi and J. Zhou, Robust and Low-Cost Flame-Treated Wood for High-Performance Solar Steam Generation, ACS Appl. Mater. Interfaces, 2017, 9, 15052–15057 CAS.
- H. Liu, C. Chen, G. Chen, Y. Kuang, X. Zhao, J. Song, C. Jia, X. Xu, E. Hitz, H. Xie, S. Wang, F. Jiang, T. Li, Y. Li, A. Gong, R. Yang, S. Das and L. Hu, High-Performance Solar Steam Device with Layered Channels: Artificial Tree with a Reversed Design, Adv. Energy Mater., 2018, 8(8), 1701616 CrossRef.
- C. Jia, Y. Li, Z. Yang, G. Chen, Y. Yao, F. Jiang, Y. Kuang, G. Pastel, H. Xie, B. Yang, S. Das and L. Hu, Rich Mesostructures Derived from Natural Woods for Solar Steam Generation, Joule, 2017, 1, 588–599 CrossRef.
- N. Xu, X. Z. Hu, W. C. Xu, X. Q. Li, L. Zhou, S. N. Zhu and J. Zhu, Mushrooms as Efficient Solar Steam-Generation Devices, Adv. Mater., 2017, 29(28), 1606762 CrossRef PubMed.
- Y. Li, T. Gao, Z. Yang, C. Chen, W. Luo, J. Song, E. Hitz, C. Jia, Y. Zhou, B. Liu, B. Yang and L. Hu, 3D-Printed, All-in-One Evaporator for High-Efficiency Solar Steam Generation under 1 Sun Illumination, Adv. Mater., 2017, 29, 1700981 CrossRef PubMed.
- P. K. Jain, X. H. Huang, I. H. El-Sayed and M. A. El-Sayed, Noble Metals on the Nanoscale: Optical and Photothermal Properties and Some Applications in Imaging, Sensing, Biology, and Medicine, Acc. Chem. Res., 2008, 41, 1578–1586 CrossRef CAS PubMed.
- S. Loeb, C. Li and J.-H. Kim, Solar Photothermal Disinfection using Broadband-Light Absorbing Gold Nanoparticles and Carbon Black, Environ. Sci. Technol., 2018, 52, 205–213 CrossRef CAS PubMed.
- O. Neumann, A. S. Urban, J. Day, S. Lal, P. Nordlander and N. J. Halas, Solar Vapor Generation Enabled by Nanoparticles, ACS Nano, 2013, 7, 42–49 CrossRef CAS PubMed.
- N. J. Hogan, A. S. Urban, C. Ayala-Orozco, A. Pimpinelli, P. Nordlander and N. J. Halas, Nanoparticles Heat through Light Localization, Nano Lett., 2014, 14, 4640–4645 CrossRef CAS PubMed.
- Z. Fang, Y.-R. Zhen, O. Neumann, A. Polman, F. J. García de Abajo, P. Nordlander and N. J. Halas, Evolution of Light-Induced Vapor Generation at a Liquid-Immersed Metallic Nanoparticle, Nano Lett., 2013, 13, 1736–1742 CrossRef CAS PubMed.
- Z. Wang, Y. Liu, P. Tao, Q. Shen, N. Yi, F. Zhang, Q. Liu, C. Song, D. Zhang, W. Shang and T. Deng, Bio-Inspired Evaporation Through Plasmonic Film of Nanoparticles at the Air–Water Interface, Small, 2014, 10, 3234–3239 CrossRef CAS PubMed.
- Y. Liu, S. Yu, R. Feng, A. Bernard, Y. Liu, Y. Zhang, H. Duan, W. Shang, P. Tao, C. Song and T. Deng, A Bioinspired, Reusable, Paper-Based System for High-Performance Large-Scale Evaporation, Adv. Mater., 2015, 27, 2768–2774 CrossRef CAS PubMed.
- Y. Liu, J. Lou, M. Ni, C. Song, J. Wu, N. P. Dasgupta, P. Tao, W. Shang and T. Deng, Bioinspired Bifunctional Membrane for Efficient Clean Water Generation, ACS Appl. Mater. Interfaces, 2016, 8, 772–779 CAS.
- S. Yu, Y. Zhang, H. Duan, Y. Liu, X. Quan, P. Tao, W. Shang, J. Wu, C. Song and T. Deng, The impact of surface chemistry on the performance of localized solar-driven evaporation system, Sci. Rep., 2015, 5, 13600 CrossRef PubMed.
- K. Bae, G. Kang, S. K. Cho, W. Park, K. Kim and W. J. Padilla, Flexible thin-film black gold membranes with ultrabroadband plasmonic nanofocusing for efficient solar vapour generation, Nat. Commun., 2015, 6, 10103 CrossRef CAS PubMed.
- L. Tian, J. Luan, K.-K. Liu, Q. Jiang, S. Tadepalli, M. K. Gupta, R. R. Naik and S. Singamaneni, Plasmonic Biofoam: A Versatile Optically Active Material, Nano Lett., 2016, 16, 609–616 CrossRef CAS PubMed.
- L. Zhou, S. Zhuang, C. He, Y. Tan, Z. Wang and J. Zhu, Self-assembled spectrum selective plasmonic absorbers with tunable bandwidth for solar energy conversion, Nano Energy, 2017, 32, 195–200 CrossRef CAS.
- C. Liu, J. Huang, C.-E. Hsiung, Y. Tian, J. Wang, Y. Han and A. Fratalocchi, High-Performance Large-Scale Solar Steam Generation with Nanolayers of Reusable Biomimetic Nanoparticles, Advanced Sustainable Systems, 2017, 1, 1600013 CrossRef.
- W. Sun, G. Zhong, C. Kübel, A. A. Jelle, C. Qian, L. Wang, M. Ebrahimi, L. M. Reyes, A. S. Helmy and G. A. Ozin, Size-Tunable Photothermal Germanium Nanocrystals, Angew. Chem., Int. Ed., 2017, 56, 6329–6334 CrossRef CAS PubMed.
- M. Chen, Y. He, J. Huang and J. Zhu, Synthesis and solar photo-thermal conversion of Au, Ag, and Au-Ag blended plasmonic nanoparticles, Energy Convers. Manage., 2016, 127, 293–300 CrossRef CAS.
- M. S. Zielinski, J.-W. Choi, T. La Grange, M. Modestino, S. M. H. Hashemi, Y. Pu, S. Birkhold, J. A. Hubbell and D. Psaltis, Hollow Mesoporous Plasmonic Nanoshells for Enhanced Solar Vapor Generation, Nano Lett., 2016, 16, 2159–2167 CrossRef CAS PubMed.
- M. Umlauff, J. Hoffmann, H. Kalt, W. Langbein, J. M. Hvam, M. Scholl, J. Söllner, M. Heuken, B. Jobst and D. Hommel, Direct observation of free-exciton thermalization in quantum-well structures, Phys. Rev. B: Condens. Matter Mater. Phys., 1998, 57, 1390–1393 CrossRef CAS.
- G. Zhu, J. Xu, W. Zhao and F. Huang, Constructing Black Titania with Unique Nanocage Structure for Solar Desalination, ACS Appl. Mater. Interfaces, 2016, 8, 31716–31721 CAS.
- M. Ye, J. Jia, Z. Wu, C. Qian, R. Chen, P. G. O'Brien, W. Sun, Y. Dong and G. A. Ozin, Synthesis of Black TiOx Nanoparticles by Mg Reduction of TiO2 Nanocrystals and their Application for Solar Water Evaporation, Adv. Energy Mater., 2017, 7, 1601811 CrossRef.
- D. Ding, W. Huang, C. Song, M. Yan, C. Guo and S. Liu, Non-stoichiometric MoO3-x quantum dots as a light-harvesting material for interfacial water evaporation, Chem. Commun., 2017, 53, 6744–6747 RSC.
- R. Chen, Z. J. Wu, T. Q. Zhang, T. C. Yu and M. M. Ye, Magnetically recyclable self-assembled thin films for highly efficient water evaporation by interfacial solar heating, RSC Adv., 2017, 7, 19849–19855 RSC.
- Y. Shi, R. Li, L. Shi, E. Ahmed, Y. Jin and P. Wang, A Robust CuCr2O4/SiO2 Composite Photothermal Material with Underwater Black Property and Extremely High Thermal Stability for Solar-Driven Water Evaporation, Advanced Sustainable Systems, 2018, 2(3), 1700145 CrossRef.
- R. Jiang, S. Cheng, L. Shao, Q. Ruan and J. Wang, Mass-Based Photothermal Comparison Among Gold Nanocrystals, PbS Nanocrystals, Organic Dyes, and Carbon Black, J. Phys. Chem. C, 2013, 117, 8909–8915 CAS.
- S. Ishii, R. P. Sugavaneshwar and T. Nagao, Titanium Nitride Nanoparticles as Plasmonic Solar Heat Transducers, J. Phys. Chem. C, 2016, 120, 2343–2348 CAS.
- X. Gao, H. Ren, J. Zhou, R. Du, C. Yin, R. Liu, H. Peng, L. Tong, Z. Liu and J. Zhang, Synthesis of Hierarchical Graphdiyne-Based Architecture for Efficient Solar Steam Generation, Chem. Mater., 2017, 29, 5777–5781 CrossRef CAS.
- C. Zhang, C. Yan, Z. Xue, W. Yu, Y. Xie and T. Wang, Shape-Controlled Synthesis of High-Quality Cu7S4 Nanocrystals for Efficient Light-Induced Water Evaporation, Small, 2016, 12, 5320–5328 CrossRef CAS PubMed.
- Z. Hua, B. Li, L. Li, X. Yin, K. Chen and W. Wang, Designing a Novel Photothermal Material of Hierarchical Microstructured Copper Phosphate for Solar Evaporation Enhancement, J. Phys. Chem. C, 2017, 121, 60–69 CAS.
- L. Zhang, B. Tang, J. Wu, R. Li and P. Wang, Hydrophobic Light-to-Heat Conversion Membranes with Self-Healing Ability for Interfacial Solar Heating, Adv. Mater., 2015, 27, 4889–4894 CrossRef CAS PubMed.
- X. Y. Huang, Y. H. Yu, O. L. de Llergo, S. M. Marquez and Z. D. Cheng, Facile polypyrrole thin film coating on polypropylene membrane for efficient solar-driven interfacial water evaporation, RSC Adv., 2017, 7, 9495–9499 RSC.
- Q. S. Jiang, H. G. Derami, D. Ghim, S. S. Cao, Y. S. Jun and S. Singamaneni, Polydopamine-filled bacterial nanocellulose as a biodegradable interfacial photothermal evaporator for highly efficient solar steam generation, J. Mater. Chem. A, 2017, 5, 18397–18402 CAS.
- X. Wu, G. Y. Chen, W. Zhang, X. Liu and H. Xu, A Plant-Transpiration-Process-Inspired Strategy for Highly Efficient Solar Evaporation, Advanced Sustainable Systems, 2017, 1, 1700046 CrossRef.
- Q. Chen, Z. Pei, Y. Xu, Z. Li, Y. Yang, Y. Wei and Y. Ji, A durable monolithic polymer foam for efficient solar steam generation, Chem. Sci., 2018, 9, 623–628 RSC.
- H. E. S. Fath, Solar distillation: a promising alternative for water provision with free energy, simple technology and a clean environment, Desalination, 1998, 116, 45–56 CrossRef CAS.
- G. N. Tiwari, H. N. Singh and R. Tripathi, Present status of solar distillation, Sol. Energy, 2003, 75, 367–373 CrossRef CAS.
- G. Ni, N. Miljkovic, H. Ghasemi, X. Huang, S. V. Boriskina, C.-T. Lin, J. Wang, Y. Xu, M. M. Rahman, T. Zhang and G. Chen, Volumetric solar heating of nanofluids for direct vapor generation, Nano Energy, 2015, 17, 290–301 CrossRef CAS.
- D. Zhao, H. Duan, S. Yu, Y. Zhang, J. He, X. Quan, P. Tao, W. Shang, J. Wu, C. Song and T. Deng, Enhancing Localized Evaporation through Separated Light Absorbing Centers and Scattering Centers, Sci. Rep., 2015, 5, 17276 CrossRef CAS PubMed.
- X. Li, R. Lin, G. Ni, N. Xu, X. Hu, B. Zhu, G. Lv, J. Li, S. Zhu and J. Zhu, Three-dimensional artificial transpiration for efficient solar waste-water treatment, Natl. Sci. Rev., 2018, 5(1), 70–77 CrossRef.
- G. Ni, G. Li, Svetlana V. Boriskina, H. Li, W. Yang, T. Zhang and G. Chen, Steam generation under one sun enabled by a floating structure with thermal concentration, Nat. Energy, 2016, 1, 16126 CrossRef CAS.
- G. L. Zhu, J. J. Xu, W. L. Zhao and F. Q. Huang, Constructing Black Titania with Unique Nanocage Structure for Solar Desalination, ACS Appl. Mater. Interfaces, 2016, 8, 31716–31721 CAS.
- W. Xu, X. Hu, S. Zhuang, Y. Wang, X. Li, L. Zhou, S. Zhu and J. Zhu, Flexible and Salt Resistant Janus Absorbers by Electrospinning for Stable and Efficient Solar Desalination, Adv. Energy Mater., 2018, 1702884, DOI:10.1002/aenm.201702884.
- A. Alkhudhiri, N. Darwish and N. Hilal, Membrane distillation: A comprehensive review, Desalination, 2012, 287, 2–18 CrossRef CAS.
- L. Martínez-Díez and M. I. Vázquez-González, Temperature and concentration polarization in membrane distillation of aqueous salt solutions, J. Membr. Sci., 1999, 156, 265–273 CrossRef.
- A. Politano, P. Argurio, G. Di Profio, V. Sanna, A. Cupolillo, S. Chakraborty, H. A. Arafat and E. Curcio, Photothermal Membrane Distillation for Seawater Desalination, Adv. Mater., 2017, 29, 1603504 CrossRef PubMed.
- J. Wu, K. R. Zodrow, P. B. Szemraj and Q. Li, Photothermal nanocomposite membranes for direct solar membrane distillation, J. Mater. Chem. A, 2017, 5, 23712–23719 CAS.
-
M. M. Eliodoro Chiavazzo, F. Viglino, M. Fasano and P. Asinari, Passive high-yield seawater desalination at below one sun by modular and low-cost distillation, 2018 Search PubMed.
- P. D. Dongare, A. Alabastri, S. Pedersen, K. R. Zodrow, N. J. Hogan, O. Neumann, J. J. Wu, T. X. Wang, A. Deshmukh, M. Elimelech, Q. L. Li, P. Nordlander and N. J. Halas, Nanophotonics-enabled solar membrane distillation for off-grid water purification, Proc. Natl. Acad. Sci. U. S. A., 2017, 114, 6936–6941 CrossRef CAS PubMed.
- A. V. Dudchenko, C. Chen, A. Cardenas, J. Rolf and D. Jassby, Frequency-dependent stability of CNT Joule heaters in ionizable media and desalination processes, Nat. Nanotechnol., 2017, 12, 557 CrossRef CAS PubMed.
- M. Fujiwara and T. Imura, Photo Induced Membrane Separation for Water Purification and Desalination Using Azobenzene Modified Anodized Alumina Membranes, ACS Nano, 2015, 9, 5705–5712 CrossRef CAS PubMed.
- M. Fujiwara and M. Kikuchi, Solar desalination of seawater using double-dye-modified PTFE membrane, Water Res., 2017, 127, 96–103 CrossRef CAS PubMed.
- M. Fujiwara, Water desalination using visible light by disperse red 1 modified PTFE membrane, Desalination, 2017, 404, 79–86 CrossRef CAS.
- P. H. Yang, K. Liu, Q. Chen, J. Li, J. J. Duan, G. B. Xue, Z. S. Xu, W. K. Xie and J. Zhou, Solar-driven simultaneous steam production and electricity generation from salinity, Energy Environ. Sci., 2017, 10, 1923–1927 CAS.
- M. Gao, P. K. N. Connor and G. W. Ho, Plasmonic photothermic directed broadband sunlight harnessing for seawater catalysis and desalination, Energy Environ. Sci., 2016, 9, 3151–3160 CAS.
- L. Zhu, M. Gao, C. K. N. Peh, X. Wang and G. W. Ho, Self-Contained Monolithic Carbon Sponges for Solar-Driven Interfacial Water Evaporation Distillation and Electricity Generation, Adv. Energy Mater., 2018, 1702149, DOI:10.1002/aenm.201702149.
- C. N. Li, Y. Goswami and E. Stefanakos, Solar assisted sea water desalination: A review, Renewable Sustainable Energy Rev., 2013, 19, 136–163 CrossRef CAS.
|
This journal is © The Royal Society of Chemistry 2018 |
Click here to see how this site uses Cookies. View our privacy policy here.