Mutual effects and in planta accumulation of co-existing cerium oxide nanoparticles and cadmium in hydroponically grown soybean (Glycine max (L.) Merr.)†
Received
5th October 2017
, Accepted 11th November 2017
First published on 14th November 2017
Abstract
Cadmium (Cd) is a metal toxic to humans even at very low concentrations. Elevated levels of Cd in soil due to various anthropogenic activities have led to higher Cd concentrations in various crop tissues, making it a food safety concern. With the progressive production and continued accumulation of engineered nanoparticles (ENPs) in agricultural soils, understanding the effect of ENPs on the uptake and accumulation of metals by plants is imperative. The goal of this study was to determine the mutual effects of cerium oxide nanoparticles (CeO2NPs) and Cd2+ on their uptake and accumulation by soybean seedlings (Glycine max (L.) Merr.) in a hydroponic system. Soybean seedlings were exposed to four treatments (1.0 mg L−1 Cd2+, 1.0 mg L−1 Cd2+ + 100 mg L−1 CeO2NPs, 100 mg L−1 CeO2NPs and 0 mg L−1 Cd and CeO2NPs as the control) for 10 days. At termination, plant roots and shoots were separated and the concentrations of Cd and Ce in these tissues were determined. In addition, the amounts of ionic Ce and particulate Ce within soybean roots were determined. Significant interactions between co-existing CeO2NPs and Cd were found with regard to their accumulation in plant tissues. While CeO2NPs did not affect the total Cd associated with soybean roots, they significantly reduced the translocation of Cd from roots to shoots by 70%. In contrast, the co-presence of Cd lowered the concentration of Ce in soybean roots by 45% but significantly increased the concentration of Ce in soybean shoots by 60%. The altered plant accumulation of co-existing Cd and Ce was attributed to various physical, chemical and biological processes that occurred in the plant rhizosphere. Specifically, the co-presence of Cd and CeO2NPs led to higher excretion of plant root exudates, which might have altered the chemical environment in the plant rhizosphere and enhanced CeO2NP dissolution, leading to different plant accumulation of both chemicals.
Environmental significance
The rapid growth of nanotechnology is accompanied by a significant increase of engineered nanoparticles (ENPs) in the environment. Their novel properties have led to a broad scrutiny of their environmental impacts. However, the potential impact of ENPs on co-occurring environmental pollutants has not been extensively explored. This manuscript reports the reciprocal effects of co-existing cerium oxide nanoparticles and Cd on the plant uptake of these chemicals and discusses the chemical mechanisms in the plant root region for their altered plant uptake. The study is expected to profoundly impact future investigations on the environmental health and safety of ENPs.
|
Introduction
Cadmium (Cd) is a ubiquitous environmental pollutant toxic to humans. Chronic exposure to Cd has been shown to be associated with a variety of health problems such as renal dysfunction, cancer, osteoporosis, and cardiovascular disease.1,2 Consumption of Cd-tainted food is a predominant pathway for human exposure to Cd due to its efficient soil-to-plant transfer.3,4 Food consumption accounts for almost 90% of Cd exposure in the general non-smoking population, rendering it an urgent task to understand processes which may potentially aggravate the plant accumulation of Cd.2
With the current embrace of nanotechnology in many industries including agriculture, engineered nanoparticles (ENPs) are increasingly detected in agricultural soils. They affect plant physiological and biochemical processes at various levels and alter the plant uptake of co-existing environmental pollutants.5,6 Earlier efforts in investigating ENPs and co-occurring environmental pollutants are mostly focused on organic contaminants.6 Few studies have evaluated the effects of ENPs on the plant uptake of co-existing heavy metals. The plant uptake of heavy metals involves complex transport processes and some are highly regulated. For example, Cd2+ is taken up by plant roots both apoplastically and symplastically.3,7 In the symplastic pathway, Cd2+ may diffuse into plant root cells passively or be actively transported in them via different transporters embedded in the root plasma membrane.3,7,8 Two types of Cd2+ transporters have been identified including the more specific Cd2+ ZIP transporters (zinc-regulated transporter/iron-regulated transporter-like proteins) and the less selective calcium permeable channels.7,9,10 Once Cd is in the plant root cells, it can be translocated to plant leaves. The translocation from roots to shoots is greatly affected by the vacuolar compartmentation of Cd–phytochelatin complexes as well as the transporters involved in the xylem loading of Cd.3,8,11,12
ENPs may potentially interrupt many of the physiological processes involved in heavy metal uptake and transport. Consequently, investigating the impact of ENPs on the plant uptake of co-existing heavy metals is of great interest. Cerium oxide nanoparticles (CeO2NPs) have been broadly used over the past years, particularly as a fuel additive.13,14 The main pathways for CeO2NPs to enter into agricultural soils include deposition of vehicle exhausts, municipal run-off and land application of bio-solids.13,14 Simultaneous exposure to both CeO2NPs and heavy metals such as Cd2+ is increasingly possible for crops. Due to the disruptive impacts of CeO2NPs on the cellular membrane as well as the plant physiology and root anatomy,15,16 CeO2NPs may substantially change the plant uptake and accumulation of heavy metals.
In addition, CeO2NPs display a high adsorption capacity for hazardous heavy metals such as arsenic, lead and Cd.17,18 Consequently, CeO2NPs may serve as carriers for Cd2+ to enter into plant tissues or retain Cd2+ in the plant rhizosphere when they are not taken up by plants.19,20 The complex interactions of CeO2NPs and other metals suggest that CeO2NPs are likely to alter the plant uptake and accumulation of co-present heavy metals. A few available studies in the literature with different ENPs corroborate this assumption. For example, TiO2NPs (100 mg L−1) were shown to significantly reduce the phytotoxicity of Cd to rice (Oryza sativa L.), which is likely due to the adsorption of Cd on TiO2.21 However, comprehensive studies and mechanistic insights into ENP–heavy metal interactions in the plant rhizosphere are lacking.
The plant uptake of ENPs may be affected by various abiotic stresses. Salt stress and nutrient deficiencies increased the plant uptake of ENP elements.22–24 ENP accumulation in plant tissues has been a food safety concern because dietary consumption of ENP contaminated plant tissues is an important pathway for human exposure to these emerging chemicals. As a result, the plant uptake of ENPs in the presence of heavy metals (a source of abiotic stress) would complement the research.
The objectives of this research were to investigate the reciprocal effects of CeO2NPs and Cd on the plant uptake of these materials by soybeans and to explore the underlying physical and chemical mechanisms leading to the altered plant uptake of these materials. Soybean (Glycine max. (L.) Merr.) was used in this study because it is a main protein source of the human diet that can accumulate metals (including Cd) in the grains.25 Soybean was reported by the Food and Agricultural Organization (FAO) as the fifth most produced crop worldwide that provides about 30% vegetable oil and 77% nitrogen fixation. The concentration of Cd in soybean seeds is positively correlated with its concentration in soil and has surpassed the maximum permissible concentration (0.2 mg kg−1) stipulated by the Codex Alimentarius Commission in some studies (FAO).26
Material and methods
CeO2NPs and Cd
CeO2NPs coated with polyvinylpyrrolidone were purchased from US Research Nanomaterials, Inc. (Houston, TX). The average size of the nanoparticles was 41.7 ± 5.2 nm, calculated by averaging the diameter of over 100 individual nanoparticles measured with ImageJ using transmission electron microscopy images of the NPs as reported earlier.27 The pH of the 100 mg L−1 CeO2NP dispersion was 7.1 and the zeta potential of the nanoparticles was −48.6 mV, determined using a dynamic light scattering Zetasizer Nano ZS90 (Malvern, UK). X-ray photoelectron spectroscopy (XPS) indicated that about 9.7% of Ce on the nanoparticle surface exists as Ce3+ (Fig. S1†).
The concentration of CeO2NPs (100 mg L−1) was chosen based on many previous nanotoxicity studies with terrestrial plants, which used concentrations mostly in the range of 1–1000 mg L−1.28 CeO2NPs at this concentration display a significant impact on plant physiological processes but not lethal effects.29,30 Cd sulfate (CdSO4) was purchased from Fisher Scientific Inc. (Pittsburgh, PA) and was dissolved in tap water to obtain a final concentration of 1.0 mg L−1 Cd.
Plant species and growth conditions
Glycine max (L.) Merr. (soybean) cv. Tohya seeds were purchased from Johnny's Selected Seeds (Winslow, ME). The soybean seeds were germinated in moist sand for 5 days. After germination, young seedlings were individually transplanted into 50 mL plastic centrifuge tubes (Becton, Dickinson and Co., Franklin Lakes, NJ, USA) filled with 25% Hoagland solution31 (Phyto Technology Lab, Shawnee Mission, KS). The transpiration rate was recorded in terms of daily water loss (mL h−1), as shown in Fig. S2.† When the water transpiration reached 50 mL per day (after 10 days), the plants were transferred from the Hoagland solution to new tubes filled with solutions containing 1.0 mg L−1 Cd2+ and/or 100 mg L−1 CeO2NPs in tap water. The Hoagland solution was avoided during the exposure period because the high ionic strength of the Hoagland solution causes nanoparticle aggregation.32 Soybean seedlings were cultivated in these solutions for an additional 8 days at room temperature under fluorescent bulbs providing 250 μmol m2 s−1 photosynthetic photon flux density (16 h light–8 h dark photoperiod). Due to water transpiration, the growth media were replenished with the same treatment solutions at least daily (on certain days, the solution was replenished twice). Seedlings grown in just tap water were used as controls. Six seedlings were grown under each treatment.
After the exposure, the pH of the growth medium was measured with a pH meter (Thermo Orion Star A121, Fisher Scientific Int., Pittsburgh, PA) and the pH of the root surface was measured with a pH strip by gently attaching the strip on the root surface until the color of the strip was stable. The strips were then compared with the matching pH chart provided by the vendor (Fisher Scientific Int., Pittsburgh, PA).
Growth analyses and biomass partitioning
Immediately after the exposure, plant roots were gently removed from the tubes and washed with 50 mL CaCl2 solution (5 mM) five times to remove the Ce and Cd elements deposited on the root surface. The washing solutions and the growth media were collected for further analysis. Fresh leaves and root samples of three replicates from each treatment were collected to quantify the dissolved vs. particulate Ce in these tissues. Detailed descriptions of the analytical parameters and procedures are provided below. The remaining three replicates from each treatment were rinsed with DI three times, divided into roots and shoots and dried in an oven at 70 °C for 7 days to determine the total concentrations of Cd and Ce associated with soybean roots and shoots.
Determination of dissolved and particulate Ce in soybean roots
Enzymatic extraction of ionic and particulate Ce from plant tissues was performed following a published protocol.33 Briefly, calcium chloride-washed fresh roots were cut into small pieces with mini-scissors, from which 0.5 g was weighed and mixed with 9 mL of 20 mM 2-(N-morpholino)ethanesulfonic acid (MES) buffer (pH = 5, adjusted with NaOH). The mixture was homogenized in a centrifuge tube using a handheld homogenizer (TH-01, OMNI International, Kennesaw, GA). One mL of 30 mg mL−1 Macerozyme R-10 enzyme (bioWORLD, Dublin, OH) (prepared in 20 mM MES) was then added into the mixture. The mixture (10 mL) was shaken at 37 °C for 24 h (New Brunswick Scientific, Edison, NJ). Afterwards, 4 mL of the digestate was filtered through a 10 kDa Amicon Ultra-4 centrifugal filter unit. The Ce in the filtrate was quantified as the ionic Ce in plant tissues by inductively coupled plasma mass spectrometry (ICP-MS) (Perkin Elmer mod. DRCII, Waltham, MA). Another 4 mL aliquot of the enzyme digestate was acid-digested to determine the total Ce in root tissues with ICP-MS. Particulate Ce in plant roots was calculated as the difference between the total Ce and the ionic Ce.
Cadmium and cerium content analyses
An aliquot of 0.25 g subsample of dry roots and 0.5 g of the shoot tissues were ground and digested with 4 mL of 70% (v/v) nitric acid using a DigiPREP MS hot block digester (SCP Science, Clark Graham, Canada) at 95 °C for 4 hours following the EPA method 3050b.34 After cooling to room temperature, 2 mL of 30% (w/v) H2O2 were added to the mixture and heated in the hot block at 95 °C for 2 hours. The Ce and Cd amounts in a final concentration of 1% nitric acid were quantified by ICP-MS.
Total organic carbon determination
Total organic carbon (TOC) in the growth media was measured as an indicator of the excretion of plant root exudates. The growth medium at termination was first diluted with tap water to 50 mL. The diluted solution was then shaken for 12 hours on a shaker table. Subsequently, the TOC in those samples was measured using a TOC analyzer (Shimadzu TOC-VWP Analyzer, Japan) following reported procedures.35,36 The TOC in the growth medium was then obtained by correcting the measured TOC with the dilution factor for each sample. Three replicates from each treatment were measured.
Investigating the association of Cd with CeO2NPs and plant root exudates
The potential chemical interactions of Cd with CeO2NPs and biomolecules in plant exudates were examined. Forty-two soybean seedlings were grown in 20% strength Hoagland solution in 50 mL centrifuge tubes for 21 days. Transpired water was replenished with Hoagland solution of the same strength during cultivation. At termination, plant seedlings were removed, and the growth medium containing root exudates was centrifuged at 10
000 rpm for 10 min. Afterwards, the supernatant was decanted and the pellet at the bottom of each centrifuge tube was gently collected and transferred to a new tube in which the pellet was combined with pellets from six other tubes to further concentrate them. The pellets were assumed to contain large molecular weight molecules from exudates such as proteins, polysaccharides and border cells. Low molecular weight molecules in exudates such as short-chain aliphatic acids were expected to remain in the supernatant and were decanted. The combined pellets were re-suspended in 40 mL DI water and altogether six such tubes were prepared. They were divided into three groups. Different combinations of Cd and CeO2NPs were introduced to these tubes. The first group contained 30 mg L−1 Cd2+, the second group 100 mg L−1 CeO2NPs and the third group both chemicals at the same concentrations. The tubes were shaken for 24 h on a shaker table and then centrifuged at 10
000 rpm for 15 min. The supernatant was then decanted, and the residual was desiccated at 90 °C for 4 hours to form a dried pellet for X-ray photoelectron spectroscopy (XPS). XPS was carried out using an Omicron ESCA (Scienta Omicron GmbH, Germany) connected to a probe spectrometer with polychromatic Mg Kα X-rays (hν = 1253.6 eV). The ground sample was mounted on a stainless-steel sample holder using double sided adhesive conductive carbon tape. The X-ray was performed at 225 W where the pass energy was set to 100 eV for survey scans and 50 eV for specific regions to ensure sufficient sensitivity under a base pressure of 10−6 Pa. The calibration of the binding energies of the core levels was fixed at 284.8 eV for the main C 1s B.E. peak. The peaks of the XPS spectrum were fitted by a Gaussian function using Origin software 2017 (OriginLab, Northampton, MA).
Statistical analyses
Data were subjected to analysis of variance using a 2 × 2 factorial experiment set-up. Two-way ANOVA was carried out to differentiate the significance of independent variables: CeO2NPs and Cd. One-way ANOVA was performed and mean separation between treatments was obtained via the Games–Howell nonparametric post hoc test. Data were analyzed using the IBM SPSS Statistics software (Version 22.0. Armonk, NY).
Results
Growth analysis and plant uptake of CeO2NPs and Cd
Neither CeO2NPs nor Cd at the applied concentrations, separately or in combination, caused any significant changes in the root and shoot dry biomass of soybean seedlings (Fig. 1). The Ce concentration increased in roots and shoots of CeO2NP treated plants compared to the controls (Fig. 2). Interestingly, the co-existence of Cd markedly decreased the concentration of Ce in soybean roots but not in shoots (Fig. 2). The Ce associated with soybean roots decreased by 45% in CeO2NPs + Cd treated plants but increased in the shoots by 44% compared to plants treated with CeO2NPs alone. Two-way ANOVA showed a significant interaction between CeO2NPs and Cd for Ce accumulation in soybean tissues. The total Cd associated with soybean roots was unaffected by the presence of CeO2NPs (Fig. 3). In contrast, the presence of CeO2NPs led to a significant decrease of Cd in plant shoots by 78%. Significant interactions between CeO2NPs and Cd were only found in the shoots for Cd accumulation.
 |
| Fig. 1 Dry weight of Glycine max grown in the presence of 100 mg L−1 CeO2NPs and 1 mg L−1 Cd. A: Root dry weight; B: shoot dry weight. The Games–Howell post hoc test reported no statistically significant differences between treatments (p < 0.05). Error bars represent the standard deviation (n = 3). Two-way ANOVA is reported in Table S1.† | |
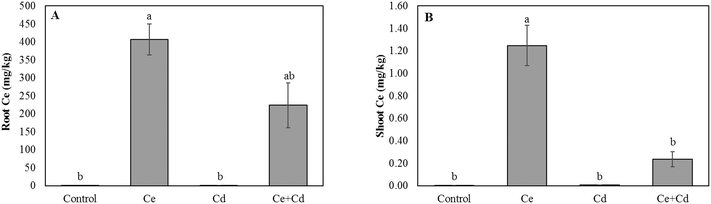 |
| Fig. 2 Total cerium concentration in roots (A) and shoots (B) of Glycine max grown in the presence of 100 mg L−1 CeO2NPs and 1 mg L−1 Cd. Means labeled with different letters are significantly different based on the Games–Howell nonparametric post hoc test (p < 0.05). Error bars represent the standard deviation (n = 3). Two-way ANOVA is reported in Table S1.† | |
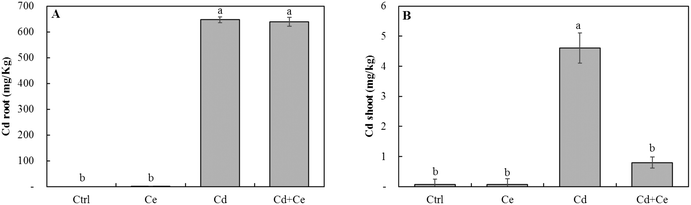 |
| Fig. 3 The cadmium concentration (mg kg−1) in roots (A) and shoots (B) of Glycine max increased in the presence of 100 mg L−1 CeO2NPs and 1 mg L−1 Cd. Means labeled with different letters are significantly different based on the Games–Howell post hoc test (p < 0.05). Error bars represent the standard deviation (n = 3). Two-way ANOVA is reported in Table S1.† | |
Speciation of CeO2NPs in soybean roots
To gain further insights into the mutual effects of CeO2NPs and Cd, in planta speciation of Ce in plant roots was determined. The concentration of ionic Ce in soybean roots increased by 65% in the presence of both CeO2NPs and Cd compared to soybeans exposed to CeO2NPs alone. (Fig. 4A). Conversely, in the presence of CeO2NPs + Cd, the concentration of particulate Ce decreased by 45% (Fig. 4B), and the CeO2NPs × Cd interaction was significant. The total Ce concentrations in the growth media and root washing solutions were determined through acid digestion and ICP-MS quantification. The Ce concentration in the growth medium was not altered by the presence of Cd and no interactions were found (Fig. 4C), but the total Ce concentration in the washing solution, presumably all forms of Ce deposited on the root surface, was increased by 47% in the co-presence of CeO2NPs and Cd2+, compared to plants exposed to CeO2NPs (Fig. 4D).
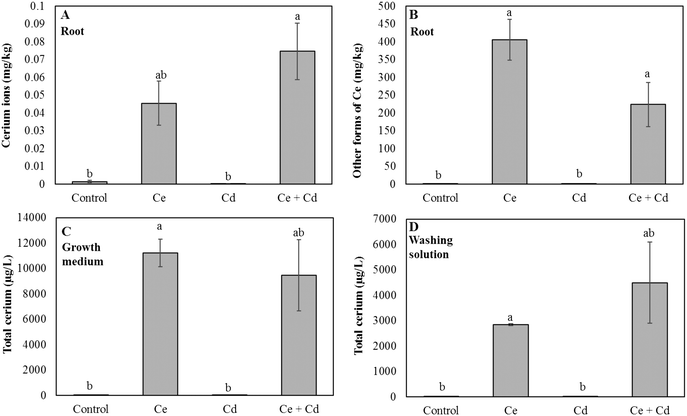 |
| Fig. 4 Cerium ions (A) and cerium particulates (B) in roots. Total cerium concentration in the growth medium (C) and in the root washing solution (D) of Glycine max grown in the presence of 100 mg L−1 CeO2NPs and 1 mg L−1 Cd. Means labeled with different letters are significantly different based on the Games–Howell's post hoc test (p < 0.05). Error bars represent the standard deviation (n = 3). Two-way ANOVA is reported in Table S1.† | |
Total organic carbon and pH
The TOC in the growth media exposed to CeO2NPs and/or Cd was higher than in control plants. The TOC in the growth medium from the joint treatment of CeO2NPs and Cd was noticeably higher than that from the treatments with CeO2NPs or Cd alone, even though the differences were not statistically significant (Fig. 5). The pH values in the growth media and on the root surface were significantly reduced by the joint exposure to CeO2NPs and Cd (Table 1), and interactions between CeO2NPs and Cd were found based on the pH on the root surface. However, no significant interactions were found between CeO2NPs and Cd in the growth media (Table 1).
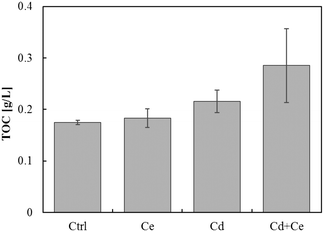 |
| Fig. 5 Total organic carbon (TOC) in Glycine max grown in the presence of 100 mg L−1 CeO2NPs and 1 mg L−1 Cd. No statistical differences were found by the Games–Howell post hoc test (p < 0.05). Error bars represent the standard deviation (n = 3). Two-way ANOVA is reported in Table S1.† | |
Table 1 Root surface and growth medium pH values of Glycine max grown in the presence of 100 mg L−1 CeO2NPs and 1 mg L−1 Cd. Means ± SD (n = 3) labeled with different letters are significantly different based on the Games–Howell post hoc test (p < 0.05)
Treatments |
Root pH |
Medium pH |
Control |
7.40 ± 0.10ab |
7.69 ± 0.15ab |
CeO2NPs |
7.53 ± 0.06a |
7.80 ± 0.06a |
Cd |
7.40 ± 0.10ab |
7.66 ± 0.13ab |
Ce NPs + Cd |
7.20 ± 0.10b |
7.50 ± 0.07b |
Two-way ANOVA |
p
|
p
|
CeO2NPs |
0.527 |
0.633 |
Cd |
0.014 |
0.025 |
CeO2NPs × Cd |
0.015 |
0.057 |
X-ray photoelectron spectroscopy
The cadmium spectra are shown in Fig. 6. Two fitting peaks (u and u′) at the binding energy of 404.82 eV and 411.82 eV were identified and were assigned to Cd3d5/2 and Cd3d3/2, respectively.37,38 No Cd specific peaks were found in CeO2 samples, but Cd peaks were detected in samples containing root exudates and 30 mg L−1 Cd alone and in samples containing part of the root exudates as well as 30 mg L−1 Cd and 100 mg L−1 CeO2NPs. Some Cd might have complexed with large molecules such as proteins and polysaccharides in plant root exudates or adsorbed on the surfaces of border cells, and some Cd might have adsorbed on the surface of CeO2NPs. The evidence provided here is only qualitative, and quantitative characterization on the interactions between Cd and exudates and CeO2NPs requires additional study, yet the results provided here do shed light on the possible mechanisms for the interactions of CeO2NPs and Cd in the plant root rhizosphere.
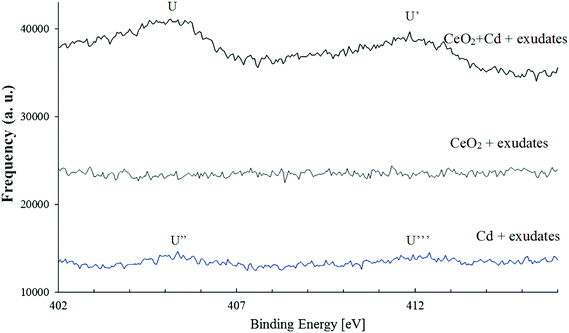 |
| Fig. 6 X-ray photoelectron spectroscopy spectra indicating the presence of cadmium (30 mg L−1) on the surface of CeO2NPs (100 mg L−1) or its association with large molecular weight molecules in soybean root exudates. The horizontal axis shows the associated binding energy [eV] and the arbitrary unit (a.u.) represents the frequency of the peaks. Two peaks at B.E. 404.82 eV and 411.82 eV were identified as characteristic peaks of Cd3d5/2 and Cd3d3/2, respectively. | |
Discussion
Interest in the interactions between ENPs and co-present environmental pollutants in different environmental systems are growing28,29,39 because these interactions may affect their accumulation in plant tissues. Consumption of food crops contaminated with various environmental pollutants is a significant pathway for human exposure to these materials.40–42
In this study, we examined the reciprocal effects of CeO2NPs and Cd in an aqueous system, avoiding many of the complications caused by soil particles and allowing a mechanistic investigation on the interactions of CeO2NPs and Cd in the soybean rhizosphere. CeO2NPs, Cd and their interactions did not affect the overall dry weight and water transpiration, confirming that CeO2NPs and Cd at the tested concentrations do not have any detrimental effects on plant growth and biomass, separately or together. Similar results have been reported in the literature. In fact, Gui et al.41 reported that lettuce (Lactuca sativa L.) treated with 100 mg kg−1 CeO2NPs grew much faster than the controls. Similarly, Cao et al.43 demonstrated that 100 mg kg−1 CeO2NPs improved the net photosynthesis rate and the Rubisco activity of soybean seedlings and enhanced the soybean water use efficiency (WUE).
The plant uptake and accumulation of Cd and Ce were affected by the co-presence of the other chemical in our study, but the extent and pattern of alteration by co-existing chemicals differed. Cd led to a significant decrease of Ce in the roots but a significant increase in the shoots. For Cd, however, the co-occurrence of CeO2NPs significantly reduced its concentration in the shoots but did not affect Cd associated with the roots. This was attributed to the complicated physical, chemical and biological processes occurring concurrently in the soybean rhizosphere. First of all, the pH in the rhizosphere and on the plant root surface decreased in the co-presence of these two materials. The pH in the rhizosphere is affected by various biological processes and the lower pH observed in this study might be partially attributed to the increased root exudation which contains a broad spectrum of acids. A lower pH generally favors the dissolution of metals.32 In addition, the low molecular weight acids in plant root exudates can function as reducing agents and therefore enhance the reduction of Ce4+ to Ce3+, facilitating Ce uptake and transport to plant leaves as ionic Ce.33 In agreement with our results in Ce speciation, a higher concentration of ionic Ce was detected in soybean roots (Fig. 4). Ionic Ce is much more efficiently transported from roots to shoots than CeO2NPs.44 Consequently, a higher Ce concentration was detected in soybean shoots exposed to both CeO2NPs and Cd than in those exposed to CeO2NPs alone, even though the total Ce within root tissues was lower when the plants were exposed to both materials simultaneously.
The plant uptake of Cd is complicated and as described above, both apoplastic and symplastic pathways contribute to the internalization of Cd into plant root cells and then in the vascular system. The presence of CeO2NPs can affect both pathways. The co-occurrence of CeO2NPs and Cd altered the formation of “root apoplastic barriers” by depositing suberin on the cell wall of endodermal cells, compared to plants exposed to them separately.16 The altered apoplastic barriers could affect Cd entrance with the water flow along the cell wall. It is also possible that CeO2NPs affect the plant symplastic pathway by altering the activities of ion transporters or the integrity of root cell membranes, leading to different Cd accumulation in plants. Such mechanisms have not been extensively explored, but some previous studies have shown that CeO2NPs may disrupt root membrane integrity.30,45 The adsorption of organic compounds on ENP surfaces plays a crucial role in the altered plant accumulation of organic compounds.46 A similar process could occur between ENPs and heavy metal ions; ENP surfaces have a high capacity for adsorption of heavy metals.17,47 The adsorption capacity of Cd on CeO2NPs was four times higher than that on TiO2NPs.18 In our study, while a detailed adsorption study was not performed, we have conducted a qualitative study in which CeO2NPs and Cd were mixed in growth media containing some actual root exudates. Cd was detected in dry pellets containing CeO2NPs, suggesting a likely adsorption of Cd on CeO2NPs (Fig. 6). Because of the relatively less efficient root-to-shoot transport of CeO2NPs, the adsorbed Cd would penetrate into roots with CeO2NPs but was less effectively transported to shoots. This possible interaction between Cd and CeO2NPs is consistent with the lower Cd concentration in plant shoots in the co-presence of CeO2NPs and Cd, while Cd in the roots was unaffected. In addition, the co-existence of CeO2NPs and Cd resulted in greater release of exudates. Cd may form complexes with organic exudates outside plant roots. The results concerning the potential complexation between Cd and root exudate molecules were inconclusive due to the weak XPS signals. However, if it is true, this complexation can be another mechanism for the altered Cd uptake in the presence of CeO2NPs because Cd chelates are transported into plant root cells through different protein transporters than Cd2+.48,49 Chelated Cd is also more effectively sequestered in the vacuole of plant root cells, reducing the loading efficiency to xylem tissues and therefore resulting in less efficient translocation to shoots.3,8,11,12 The adsorption of Cd onto biomolecules has been shown to be strongest at pH > 5.37 The pH in the growth media in this study was above 6, suggesting that affiliation between Cd and large size biomolecules in root exudates was possible. However, further evidence is needed to substantiate this hypothesis.
In summary, the Cd × CeO2NPs interaction was statistically significant for plant accumulation of co-existing Cd and CeO2NPs. The co-presence of Cd with CeO2NPs led to higher excretion of plant root exudates, lowered the pH values on the root surface and enhanced CeO2NPs dissolution, and therefore resulted in higher accumulation of Ce in plant shoots compared to plants exposed to CeO2NPs alone. A higher Ce content in the root washing solution indicated that a higher amount of Ce was attached to the plant root surface but did not penetrate into the tissues. The presence of CeO2NPs may provide an adsorption site for Cd and reduces its uptake into plants. The elevated release of root exudates may be another possible reason for the reduced Cd in plant shoots due to the possible complexation between Cd and root exudate molecules. The study provides important new insights into the chemical mechanisms for Cd and CeO2NP interactions in the plant root region. Due to the complex processes involved in both materials, future studies should aim to elucidate more molecular mechanisms for the mutual effects of these chemicals on their uptake and accumulation by plants.
Conflicts of interest
There are no conflicts to declare.
Acknowledgements
This project has been funded by the Texas A&M Engineering Experiment Station, the Texas A&M University College of Engineering, and the Texas A&M University Division of Research Strategic Areas Interdisciplinary Research Seed Grants.
References
- S. Clemens and J. F. Ma, Annu. Rev. Plant Biol., 2016, 67, 489–512 CrossRef CAS PubMed
.
- S. Clemens, M. G. Aarts, S. Thomine and N. Verbruggen, Trends Plant Sci., 2013, 18, 92–99 CrossRef CAS PubMed
.
- L.-Z. Li, C. Tu, W. J. G. M. Peijnenburg and Y.-M. Luo, Environ. Pollut., 2017, 221, 351–358 CrossRef CAS PubMed
.
- L.-l. Lu, S.-k. Tian, X.-e. Yang, T.-q. Li and Z.-L. He, J. Plant Physiol., 2009, 166, 579–587 CrossRef CAS PubMed
.
- F. Cai, X. Wu, H. Zhang, X. Shen, M. Zhang, W. Chen, Q. Gao, J. C. White, S. Tao and X. Wang, NanoImpact, 2017, 5, 101–108 CrossRef
.
- R. De La Torre-Roche, J. Hawthorne, Y. Deng, B. Xing, W. Cai, L. A. Newman, Q. Wang, X. Ma, H. Hamdi and J. C. White, Environ. Sci. Technol., 2013, 47, 12539–12547 CrossRef CAS PubMed
.
- A. Lux, M. Martinka, M. Vaculík and P. J. White, J. Exp. Bot., 2010, 62, 21–37 CrossRef PubMed
.
- D. A. Cataldo, T. R. Garland and R. E. Wildung, Plant Physiol., 1983, 73, 844–848 CrossRef CAS PubMed
.
- L. Lu, S. Tian, M. Zhang, J. Zhang, X. Yang and H. Jiang, J. Hazard. Mater., 2010, 183, 22–28 CrossRef CAS PubMed
.
- M. L. Guerinot, Biochim. Biophys. Acta, Biomembr., 2000, 1465, 190–198 CrossRef CAS
.
- T. Shute and S. M. Macfie, Sci. Total Environ., 2006, 371, 63–73 CrossRef CAS PubMed
.
- L. Tudoreanu and C. Phillips, Adv. Agron., 2004, 84, 121–157 CAS
.
- A. C. Johnson and B. Park, Environ. Toxicol. Chem., 2012, 31, 2582–2587 CrossRef CAS PubMed
.
- Z. Hu, S. Haneklaus, G. Sparovek and E. Schnug, Commun. Soil Sci. Plant Anal., 2006, 37, 1381–1420 CrossRef CAS
.
- L. Zhao, B. Peng, J. A. Hernandez-Viezcas, C. Rico, Y. Sun, J. R. Peralta-Videa, X. Tang, G. Niu, L. Jin and A. Varela-Ramirez, ACS Nano, 2012, 6, 9615–9622 CrossRef CAS PubMed
.
- L. Rossi, W. Zhang, A. P. Schwab and X. Ma, Environ. Sci. Technol., 2017, 51, 12815–12824 CrossRef CAS PubMed
.
- C.-Y. Cao, Z.-M. Cui, C.-Q. Chen, W.-G. Song and W. Cai, J. Phys. Chem. C, 2010, 114, 9865–9870 CAS
.
- A. R. Contreras, A. Garcia, E. Gonzalez, E. Casals, V. Puntes, A. Sanchez, X. Font and S. Recillas, Desalin. Water Treat., 2012, 41, 296–300 CrossRef CAS
.
- E. Casals, T. Pfaller, A. Duschl, G. J. Oostingh and V. F. Puntes, Small, 2011, 7, 3479–3486 CrossRef CAS PubMed
.
- S. Schymura, T. Fricke, H. Hildebrand and K. Franke, Angew. Chem., Int. Ed., 2017, 56, 7411–7414 CrossRef CAS PubMed
.
- Y. Ji, Y. Zhou, C. Ma, Y. Feng, Y. Hao, Y. Rui, W. Wu, X. Gui, V. N. Le, Y. Han, Y. Wang, B. Xing, L. Liu and W. Cao, Plant Physiol. Biochem., 2017, 110, 82–93 CrossRef CAS PubMed
.
- S. Pradhan and D. Mailapalli, J. Agric. Food Chem., 2017, 65, 8279–8294 CrossRef CAS PubMed
.
-
A. Dev, A. K. Srivastava and S. Karmakar, in Nanoscience in Food and Agriculture 5, Springer, 2017, pp. 169–204 Search PubMed
.
- S. Pradhan and D. R. Mailapalli, J. Agric. Food Chem., 2017, 65, 8279–8294 CrossRef CAS PubMed
.
- Y. Zhao, X. Fang, Y. Mu, Y. Cheng, Q. Ma, H. Nian and C. Yang, Bull. Environ. Contam. Toxicol., 2014, 92, 427–432 CrossRef CAS PubMed
.
- A. M. Newbigging, X. Yan and X. C. Le, J. Environ. Sci., 2015, 37, 157–162 CrossRef PubMed
.
- L. Rossi, W. Zhang, L. Lombardini and X. Ma, Environ. Pollut., 2016, 219, 28–36 CrossRef CAS PubMed
.
- P. A. Holden, F. Klaessig, R. F. Turco, J. H. Priester, C. M. Rico, H. Avila-Arias, M. Mortimer, K. Pacpaco and J. L. Gardea-Torresdey, Environ. Sci. Technol., 2014, 48, 10541–10551 CrossRef CAS PubMed
.
- M. N. Khan, M. Mobin, Z. K. Abbas, K. A. AlMutairi and Z. H. Siddiqui, Plant Physiol. Biochem., 2017, 110, 194–209 CrossRef CAS PubMed
.
- X. Ma, Q. Wang, L. Rossi and W. Zhang, Environ. Sci. Technol., 2015, 50, 6793–6802 CrossRef PubMed
.
- D. R. Hoagland and D. I. Arnon, Circ. - Calif. Agric. Exp. Stn., 1950, 347 Search PubMed
.
- F. Schwabe, R. Schulin, P. Rupper, A. Rotzetter, W. Stark and B. Nowack, J. Nanopart. Res., 2014, 16, 2668 CrossRef
.
- W. Zhang, Y. Dan, H. Shi and X. Ma, J. Environ. Chem. Eng., 2017, 5, 572–577 CrossRef CAS
.
-
USEPA, Hazardous Waste Test Methods/SW-846, Environmental Protection Agency, Office of Solid Waste, 1996, p. 12 Search PubMed.
- S.-Z. Wu, K. Li and W.-D. Zhang, Appl. Surf. Sci., 2015, 324, 324–331 CrossRef CAS
.
- C. A. Ming and T. Takako, Notes, 2003, 2, 0.9995 Search PubMed
.
- G. Tan and D. Xiao, J. Hazard. Mater., 2009, 164, 1359–1363 CrossRef CAS PubMed
.
- F.-Z. Wang, D.-C. Shang, M.-G. Wang, S.-G. Hu and Y.-Q. Li, J. Cleaner Prod., 2016, 112, 2292–2299 CrossRef CAS
.
- R. Grillo, A. H. Rosa and L. F. Fraceto, Chemosphere, 2015, 119, 608–619 CrossRef CAS PubMed
.
- M. A. Maurer-Jones, I. L. Gunsolus, C. J. Murphy and C. L. Haynes, Anal. Chem., 2013, 85, 3036–3049 CrossRef CAS PubMed
.
- X. Gui, Z. Zhang, S. Liu, Y. Ma, P. Zhang, X. He, Y. Li, J. Zhang, H. Li, Y. Rui, L. Liu and W. Cao, PLoS One, 2015, 10, e0134261 Search PubMed
.
- G. Mustafa and S. Komatsu, Biochim. Biophys. Acta, Proteins Proteomics, 2016, 1864, 932–944 CrossRef CAS PubMed
.
- Z. M. Cao, C. Stowers, L. Rossi, W. L. Zhang, L. Lombardini and X. Ma, Environ. Sci.: Nano, 2017, 4, 1086–1094 RSC
.
- W. Zhang, Y. Dan, H. Shi and X. Ma, ACS Sustainable Chem. Eng., 2016, 4, 5424–5431 CrossRef CAS
.
- X. Ma, Q. Wang, L. Rossi, S. Ebbs and J. White, NanoImpact, 2016, 1, 46–54 CrossRef
.
- X. Ma and C. Wang, Environ. Eng. Sci., 2010, 27, 989–992 CrossRef CAS
.
- A. Contreras, E. Casals, V. Puntes, D. Komilis, A. Sánchez and X. Font, Global NEST J., 2015, 17, 536–543 Search PubMed
.
- Y. Song, L. Jin and X. Wang, Int. J. Phytorem., 2017, 19, 133–141 CrossRef CAS PubMed
.
- Y.-F. Lin and M. G. Aarts, Cell. Mol. Life Sci., 2012, 69, 3187–3206 CrossRef CAS PubMed
.
Footnotes |
† Electronic supplementary information (ESI) available. See DOI: 10.1039/c7en00931c |
‡ Current Address: Center for Research in Energy and Environment, Dept. of Civil, Architectural and Environmental Engineering, Missouri University of Science & Technology, Rolla, MO, USA. |
§ These authors contributed equally to the manuscript. |
¶ Current Address: Department of Civil and Environmental Engineering, Hong Kong University of Science & Technology, Hong Kong, China. |
|
This journal is © The Royal Society of Chemistry 2018 |
Click here to see how this site uses Cookies. View our privacy policy here.