Responses of deposition and bioaccumulation in the Great Lakes region to policy and other large-scale drivers of mercury emissions†
Received
13th November 2017
, Accepted 2nd January 2018
First published on 23rd January 2018
Abstract
Mercury (Hg) emissions pose a global problem that requires global cooperation for a solution. However, neither emissions nor regulations are uniform world-wide, and hence the impacts of regulations are also likely to vary regionally. We report here an approach to model the effectiveness of regulations at different scales (local, regional, global) in reducing Hg deposition and fish Hg concentrations in the Laurentian Great Lakes (GL) region. The potential effects of global change on deposition are also modeled. We focus on one of the most vulnerable communities within the region, an Indigenous tribe in Michigan's Upper Peninsula (UP) with a high fish consumption rate. For the GL region, elements of global change (climate, biomass burning, land use) are projected to have modest impacts (<5% change from the year 2000) on Hg deposition. For this region, our estimate of the effects of elimination of anthropogenic emissions is a 70% decrease in deposition, while our minimal regulation scenario increases emissions by 35%. Existing policies have the potential to reduce deposition by 20% with most of the reduction attributable to U.S. policies. Local policies within the Great Lakes region show little effect, and global policy as embedded in the Minamata Convention is projected to decrease deposition by approximately 2.8%. Even within the GL region, effects of policy are not uniform; areas close to emission sources (Illinois, Indiana, Ohio, Pennsylvania) experience larger decreases in deposition than other areas including Michigan's UP. The UP landscape is highly sensitive to Hg deposition, with nearly 80% of lakes estimated to be impaired. Sensitivity to mercury is caused primarily by the region's abundant wetlands. None of the modeled policy scenarios are projected to reduce fish Hg concentrations to the target that would be safe for the local tribe. Regions like Michigan's UP that are highly sensitive to mercury deposition and that will see little reduction in deposition due to regulations require more aggressive policies to reduce emissions to achieve recovery. We highlight scientific uncertainties that continue to limit our ability to accurately predict fish Hg changes over time.
Environmental significance
Here, we project the responses within the Great Lakes region of rates of atmospheric deposition and fish mercury concentrations to changes in policy at multiple jurisdictional scales and to global change. We demonstrate that even within this region, responses to policy and global change drivers vary spatially with the largest reductions in deposition occurring in closer proximity to major Hg emission sources. Projected responses to atmospheric deposition are greater for U.S. policies than for either local or global Hg control policies. We focus further on the environmental justice implications of policy changes for fish mercury contamination facing one of the most vulnerable communities within the region, a tribe in Michigan's Upper Peninsula with a high fish consumption rate. The Upper Peninsula is shown to be a highly sensitive landscape that readily converts atmospherically deposited Hg to bioaccumulated methylmercury, but one that will respond little to any of the policies evaluated. Thoughtful and more aggressive policy changes would be required to alleviate the existing environmental injustice.
|
1. Introduction
Mercury (Hg) is a toxic pollutant that, when emitted to the environment, undergoes volatilization, long-range transport and deposition in its oxidized form, transformation to its more toxic form, methylmercury (MeHg), and bioaccumulation in ecosystems. It is at this point that Hg poses threats to human health through consumption of fish containing high levels of MeHg. In the Laurentian Great Lakes (GL) region, fish tissue concentrations are unsafe for GL residents as evidenced by statewide fish consumption advisories.1–3 Fishing communities are burdened with the majority of negative impacts,4–8 and Native American fishing rights and cultures, in particular, have been severely impacted by Hg contamination;4,7,9–12 thus Hg contamination is an environmental and social justice issue. Most stakeholders involved in the effort to reduce Hg contamination recognize that action is needed at the global as well as the regional level. Toward this end, nations have signed and ratified the Minamata Convention on mercury, which sets expectations associated with controls on emissions of Hg.13 A challenge now is to estimate when it will be possible to safely consume fish in places such as the GL region as nations implement the Minamata Convention. In partnership with the Keweenaw Bay Indian Community (KBIC), an Indigenous tribe in Michigan's Upper Peninsula (UP), we formulated14 and conducted an integrated assessment to address the question, “Can we, by 2050, meet the goal of safe fish consumption?”
Because of the atmospheric residence times of different Hg species, the answer to this question is location dependent. Much of the Hg deposition to forests is via dry deposition of Hg0 which because of its long atmospheric residence time (2.7–12 months)15,16 can be due to either regional or global sources. In contrast, deposition (wet and dry) of the much shorter lived (0.5–2 days)15 Hg(II) species is affected primarily by local and regional emissions. Understanding the importance of Hg deposition from local and regional sources in the U.S. (the largest fraction of which comprise coal-fired power plants), in particular within the GL region, continues to evolve. In the 1980s some thought that atmospheric residence times of Hg were long (12–18 months),17 and that atmospheric Hg deposition was due to global-scale processes. In 1992 it was shown that local and regional anthropogenic sources of Hg in the GL region could determine Hg concentrations in the litter and mineral soil horizons;18 those concentrations paralleled changes in wet sulfate deposition and human activity. In 2004, using a combination of a global chemical transport model (CTM) and a nested regional CTM, Seigneur et al.19 showed that Hg deposition in the contiguous U.S. was, on average, comprised of ∼30% emissions from North America.19 By constraining the estimates of wet deposition by a global transport model (GEOS-Chem) with measurements, Selin and Jacob20 estimated that North American anthropogenic emissions contributed 50% of total deposition in the Midwest and Eastern U.S. Cohen et al.21 used trajectory analysis (HYSPLIT) to discern differences in emission sources of Hg to the five GL. They found that Lake Erie, which is downwind of significant local/regional emission sources, was the most impacted by local and regional emissions (58% of the base case total deposition), while Lake Superior, with the fewest upwind local/regional sources, was the least impacted by these sources (27%). Recently, Zhou et al. showed that decreased atmospheric Hg concentrations measured during the past decade in the northeastern U.S. are consistent with decreased Hg emissions from regional point sources, and that increasing global emissions have not overwhelmed those decreases.22 Potential effects of local, regional and global regulation of Hg emissions on deposition across the GL region are investigated in this paper.
The concept of landscape sensitivity to Hg deposition is well established in the literature.23–25 It is clear that landscapes with abundant forests and wetlands, as well as low nutrients and alkalinity in runoff are predisposed to having high MeHg concentrations in lakes and fish.24,26 Such regions require lower rates of atmospheric Hg deposition than other regions to avoid high MeHg concentrations in fish. Studies also suggest that some of these regions may respond more slowly to decreases in Hg deposition because the Hg accumulated in organic-rich soils may continue to be mobilized to lakes by the high and potentially increasing27–29 concentrations of dissolved organic matter in runoff typical of these regions. Multiple studies have shown that some lakes can recover quickly from Hg contamination30–32 or reduced Hg or sulfate deposition.33–37 However, modeling studies,38–40 large surveys36 and synthesis studies41 revealed considerable variability among individual lakes that is mediated by biogeochemical conditions in a lake and/or its watershed as well as by the mechanism for Hg input. In northern Wisconsin, a seepage lake with little catchment showed a rapid decline in water and fish Hg over the same time that a drainage lake with considerable wetland in the catchment showed a decline in total Hg but little change in MeHg.42 Within Michigan, concentrations of Hg in multiple lakes and rivers increased from 1998 to 2009 while atmospheric deposition of Hg and sulfate decreased.43 In England, the leaching of Hg from catchment soils continues to prolong lake recovery.44 The combination of variability among lakes and our level of understanding of key watershed features results in a wide range (a few years to centuries) in model-predicted times for lake recovery.45–47 In this paper, we highlight spatial variability in the landscape that results in different responses of lakes to Hg deposition.
The objectives of this paper are to present an approach to estimate effects of policy and global changes on Hg concentrations in fish, to apply this approach to the GL region, to examine spatial differences in response to global and policy change, and to evaluate implications of the predicted lake responses particularly for the inhabitants of Michigan's Upper Peninsula. We restrict our regional scale comparisons to changes in atmospheric deposition and landscape sensitivity, two of the primary determinants of bioaccumulation in lakes. We evaluate lake responses and their implications on a local scale, focusing on Michigan's UP.
2. Experimental
2.1 Description of study area
This study contrasted forcings (global change, policies) and responses on scales ranging from global to regional (hundreds to a few thousand kilometers) to local (tens to a few hundred kilometers). We present here only the regional (Great Lakes region) and local (UP, Adirondack area) scale responses (atmospheric deposition of Hg, fish Hg concentrations). We defined the Great Lakes region as bounded by 40° and 50° N latitude and by 73° to 95° W longitude. The UP (44 × 103 km2) is defined spatially by the land border between Wisconsin and Michigan to the west, Lake Superior to the north, and Lake Michigan–Huron to the south and east. The 11.3 × 103 km2 Adirondack area defined for this project was the rectangle bounded south to north by 43.7 to 44.3° N and east to west by 73.9 to 75.3° W. Both local areas are largely covered with mixed deciduous-coniferous forests (UP – 77%, Adirondacks – 74%; National Land Cover Database101), and both have a high density of lakes (UP – 3.8% of area; Adirondacks – 5.1% of area). The UP has more wetlands (29% vs. 11%; National Wetland Inventory), while the Adirondacks have higher elevation, steeper topography, and higher rates of sulfate deposition.
2.2 Modeling approach to estimate atmospheric deposition and fish Hg responses to global change and emissions policies
In general terms, our approach to estimating lake responses to forcings was to construct multiple scenarios embodying different regulatory approaches or global change conditions, to estimate Hg emissions for these scenarios, to use GEOS-Chem to predict the spatial distribution of Hg deposition rates within the Great Lakes region, and then to utilize the predicted Hg deposition rates in a model of watershed and lake Hg cycling. Scenarios used the approximate year 2000 as the “present day” situation with which to compare alternate emission scenarios. To obtain a significant difference from the present but avoid projecting too far into an uncertain future, we selected the year 2050 as the common future time at which scenarios would be compared. Because Hg emissions and distributions are influenced by other drivers of global change (climate change, land-use/cover, biomass burning), we created scenarios to estimate the changes in Hg deposition caused by these forcings. For all scenarios, we evaluated the predicted spatial changes in Hg deposition within the Great Lakes region, and for selected policy scenarios we contrasted atmospheric deposition in two local areas to highlight spatial variability within the region.
2.2.a Target MeHg concentration in fish.
To contextualize the current and predicted mercury concentrations in fish, project social scientists engaged our community partner, the KBIC, as described in detail by Gagnon et al.14 The high fish consumption rate of this tribal community renders it one of the most vulnerable subpopulations within the Great Lakes region. The community partners defined ‘desired’ walleye consumption as two 8-oz. (227 gram) meals per day, or a total of 0.454 kg fish per day. This represents a rate typical of the spring walleye harvest, when regional fishing activities are highest.14 This fish consumption rate was entered into the equation to compute a target MeHg concentration in fish as follows.
where, for illustration purposes, we use a body weight of 70 kg to be consistent with the fish consumption rate, and, as a benchmark, the U.S. Environmental Protection Agency (EPA) reference dose48 RfD of
. We acknowledge that recent studies have identified mercury impacts at levels that are lower than the studies used to set the EPA's guideline,49 and that advocacy groups have called for lowering this limit.50 The target concentration provided a benchmark against which to judge the effects of policy scenarios, but clearly the target varies with the target population, is not uniform across the region and will evolve as knowledge of health impacts improves.
2.2.b Formulation of global change scenarios.
We used a scenario approach to consider the potential impact of four global change drivers on future atmospheric deposition and fish tissue concentrations of Hg in the GL region: policy, climate change, land-use and land-cover change, and biomass burning. Although there are interactions between these four drivers, we evaluated the impact of each separately, holding the other factors constant, to better isolate influences on Hg biogeochemical cycling. These scenarios draw from previous publications, so they are described only briefly and summarized in Table 1.
Table 1 Summary of global change scenarios
Driver |
Scenario name |
Description |
Policy51 |
2000s |
Anthropogenic emissions for 2005 over the U.S. and Canada and for 2006 elsewhere in the world are used as representative present day emissions at the turn of the 21st century. |
Future: aspirational |
Assumed cessation of anthropogenic emissions of Hg in 2050. |
Future: policy-in-action |
Recent policy proposals targeting Hg emissions (in the GL, in the U.S., and globally) are assumed to be fully implemented by 2050. |
Future: minimal regulation |
Limited global progress on Hg and other sustainability goals are assumed by 2050. |
Climate change52,53 |
2000s |
Meteorology simulated with the NASA GISS GCM. An average of simulated 1998–2002 meteorology is used to represent present, turn of the 21st century climate. |
Future |
NASA GISS GCM used with greenhouse gas trends following the IPCC A1B scenario. An average of simulated 2048–2052 meteorology, is used to represent mid-century climate. |
Land-use and land-cover change54,55 |
2000s |
Land use simulated by the IMAGE model. Land cover (1998–2002) simulated using the LPJ dynamic global vegetation model driven with the GISS GCM meteorology and CO2 concentrations. |
Future |
Future (2050s) represents the period 2048–2052. Changes in land use follow the IPCC A1B scenario. Land cover distributions generated from the LPJ model with GISS GCM meteorology and CO2 concentrations following the IPCC A1B scenario. |
Biomass burning56,57 |
2000s |
Biomass burning emissions estimated from a fire emissions model. Average emissions for the period 1998–2002 representing present day are used. |
Future |
Refers to the 2050s. Fire emissions model driven with 2050s fire frequencies, land use-land cover distributions, meteorology and burned area following the IPCC A1B scenario. Average emissions for the period 2048–2052 are used. |
2.2.c Policy scenarios.
To better understand the impact of anthropogenic forcing on future atmospheric deposition and fish tissue concentrations, we considered present day emissions, and three future emissions scenarios capturing a range of policy ambition: aspirational, policy-in-action, and minimal-regulation. We implemented the anthropogenic emission scenarios defined below in spatially resolved emissions inventories that are described fully by Corbitt et al.58 and Giang and Selin.51 Our present day, turn-of-the-21st-century emissions are based on year 2005 for the U.S. and Canada,51,59 and 2006 emissions elsewhere in the world.58,60
Aspirational.
Our aspirational scenario assumed cessation of anthropogenic emissions of Hg in 2050. Preventing these emissions would require transitions to Hg-free alternatives (e.g., renewable energy sources such as wind and solar, LED light bulbs), and advanced capture and control technologies, some of which may not currently exist. In this scenario and all others, we assume that legacy emissions remain at their year 2000 value, to facilitate comparison of the direct anthropogenic component.
Policy-in-action.
This scenario assumed that by 2050, recent policy proposals targeting Hg emissions are fully implemented. This scenario was developed at three nested geographic domains: local (Lake Superior Basin), regional (U.S.), and global. The global and regional scenarios are described fully in Giang and Selin.51 Globally, we assumed the recently adopted United Nations Minamata Convention on mercury is fully in force in the global community.13 Moreover, we assumed some global cooperation on broad sustainability issues like climate change, following the IPCC SRES B1 scenario, as described by Streets et al.60 Current projections based on assessments of technology and energy trends suggest that with mitigation efforts consistent with the Minamata Convention text and transitions away from coal combustion, emissions in 2050 could be stabilized roughly at current-day levels.60–62 Additional benefits from more ambitious climate action could result in further reductions, though we do not consider those here.63 Regionally, within the U.S., we assumed that regulations targeting emissions under the Clean Air Act are in place and enforced through 2050, including the Mercury and Air Toxics Standards (MATS).51 Locally, in the Lake Superior Basin, we assumed that zero discharge goals have been achieved by 2050.64
Minimal-regulation.
In our minimal-regulation scenario, we modeled limited global progress on Hg and other sustainability goals by 2050. We assumed limited advances in the efficacy of air pollution control technologies, limited adoption of existing control technologies globally, and continued global growth in coal combustion consistent with the IPCC SRES A1B scenario, as described by Streets et al.60 Under these minimal-regulation conditions, Hg emissions in 2050 are close to double those of present day levels.60 Additional details for this scenario are provided by Giang and Selin.51
2.2.d GEOS-Chem simulation of atmospheric deposition of total Hg.
The GEOS-Chem coupled atmosphere-land-surface ocean Hg model, version v9-02, was used to simulate the impact of changes in emissions (anthropogenic, biomass burning), land use/land cover change, and climate on atmospheric Hg deposition to the GL.65–68 Additional details on model and simulation design are given by Zhang et al.,55 Giang and Selin,51 and Kumar et al.57
For anthropogenic emissions scenarios under all policy options, our GEOS-Chem simulations are driven with assimilated meteorology from the NASA Goddard Earth Observing System (GEOS-5). Using this meteorology allowed us to simulate Hg chemistry and transport at a global resolution of 4° × 5°, and at a finer 1/2° × 2/3° resolution over North America through a one-way nested grid model.59 For each of the four anthropogenic emissions scenarios for 2050 defined above, we simulated meteorological years 2007–2011, using the first two years as initialization. By holding meteorology constant, we isolated the effect of emissions changes alone. Results presented are interannual averages for meteorological years 2009–2011.
To examine the impacts from climate change and land use/land cover change in the coming decades, we carried out model simulations under various scenarios for climate, land use and land cover. For example, we compared simulation results with the present (year 2000) and future (mid-century: 2050) climate to derive the potential impact of climate change on Hg deposition to the GL region. More details about the scenarios can be found in Zhang et al.55 All GEOS-Chem simulations were driven by meteorological fields from the NASA Goddard Institute for Space Studies (GISS) General Circulation Model (GCM 3).69 The coupling between GISS GCM3 and GEOS-Chem model is described by Wu et al.52 We simulated meteorological years 1998–2002 for the present day and 2048–2052 for the future scenario at 4° × 5° horizontal resolution globally. We analyze the results based on five-year averages.
We also accounted for the potential impacts of global change (including changes in population, climate, land use and land cover) on Hg emissions from wildfires and consequently on Hg deposition. Changes in land use/land cover can affect vegetation type/availability while climate is an important determinant of conditions favorable for fire occurrence and natural ignitions (lightning). In addition, human interactions with natural vegetation (e.g. use of fire for vegetation clearing) are a source of anthropogenic ignitions for wildfires while fire suppression is greater in areas of high population density. We took advantage of a recently developed fire emission model for Hg.57 We calculated the Hg emissions from wildfires for the present day (2000s) and future (2050s), respectively, and implemented these emissions in the GEOS-Chem model to examine the perturbations to atmospheric Hg burden and deposition.
The GEOS-Chem model as used here represents impacts from near-term changes in emissions, but does not capture alterations in longer-term legacy biogeochemical cycling changes in response to emissions changes, that were held constant in all of our scenarios as noted above. The potential implications of these changes that can impact legacy emissions are discussed further below.
2.2.e Lake mass balance modeling.
The mass balance model for Hg of Hendricks et al.70 was used to predict aqueous concentrations based on the characteristics of a lake (depth, water retention time, trophic state) and its watershed (area, land cover and wetland extent), and the local rates of Hg atmospheric deposition (Fig. 1). Hg species included elemental, divalent, and MeHg in the lake compartments epilimnion, hypolimnion, and sediments; this resulted in a system of nine ordinary differential equations (ODE) that was solved using a numerical ordinary differential equation solver package for R.71 The model was based loosely on EPA's spreadsheet model SERAFM,72 but it was adjusted for non-steady state conditions to enable prediction of lake responses to changes in loadings. Chemical and physical processes of Hg in the lake that were modeled included redox reactions of divalent and elemental Hg, methylation, demethylation, photodemethylation, thermocline dispersion, partitioning (phases included DOC, abiotic solids, biotic solids, and sediments), diffusion in sediments, settling, burial, and resuspension. Seasonal changes in lake temperature, stratification, and ice cover were simulated. Redox reactions, methylation, and demethylation were corrected for these changes in temperature of the lake. Photodemethylation was corrected for the amount of light attenuation received in the water column. The rate of thermocline dispersion changed seasonally to allow for mixing and stratification in this dimictic lake. Atmospheric deposition during ice cover “accumulated” on top of the ice until spring melt when it entered the lake. The model does not include fish population dynamics; rather, it uses bioaccumulation factors for mixed feeders and piscivorous fish taken from Knightes.73 Hence, the model ignores the 3–7 years required for fish populations to come to steady state when loadings change.74,75 In the results presented in this paper, uncertainties reflect only the distribution of bioconcentration factors, rather than the total error calculated via Bayesian techniques by Hendricks.70 This model was validated for a lake in Michigan's UP for which Hg concentrations had been measured in the water, sediments and fish. Characteristics of this lake are summarized in Table 2.
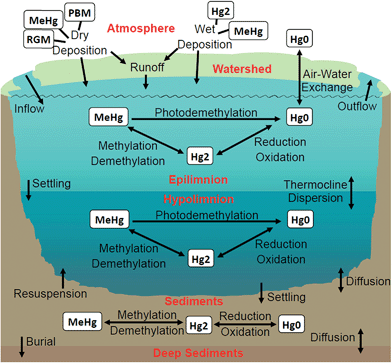 |
| Fig. 1 Schematic of mercury processes and pathways incorporated in the lake and watershed mercury model. The watershed is semi-distributed spatially to account for categories of land use (wetland, forest, urban). Results presented here assume runoff coefficients for each land use are fractions of annual deposition. In addition to the cycling of mercury, the model incorporates seasonal variations in temperature, lake mixing, DOC inputs, and phytoplankton abundance. | |
Table 2 Summary of characteristics of the modeled lake. Data from mixed sources as summarized by Hendricks86
Parameter |
Value or range |
Surface area |
9.7 × 106 m2 |
Volume |
1.4 × 108 m3 |
Mean depth |
15 m |
Watershed area |
1.9 × 108 m2 |
Wetland area |
2.7 × 107 m2 |
pH |
7.55 |
DOC |
7.4 mg L−1 |
Lake temperature |
1.2–18.1 °C |
Water residence time |
1 year |
Sediment Hg |
200–400 μg g−1 |
Lake Hg |
0.6–0.8 ng L−1 |
The model requires inputs of atmospheric concentrations of Hg(0), particulate bound mercury (PBM), MeHg, and reactive gaseous Hg (RGM) to calculate deposition to the lake as well as wet and dry deposition fluxes of all species to the watershed. In contrast to many previous studies,76–78 we did not assume that dry deposition would be the same to lakes as to their watersheds. The theoretical basis for our assumption is well established. The roughness length for lakes is smaller than for tree canopies resulting in a higher friction velocity.79,80 Alternatively, the difference may be understood in terms of the resistance components to deposition; for tree canopies these typically include surface (stomatal, cuticular), aerodynamic, and quasi-laminar boundary resistances81 while water has different surface components. Additionally, in small lakes air–water gas exchange is facilitated by convective mixing such that transfer velocities are faster than those estimated based on boundary layer or surface renewal models.82 To estimate dry deposition to lakes and watersheds, we used the Hg species- and landform-specific deposition velocities in GEOS-Chem.67 Gas exchange with the lake was estimated using deposition velocities for RGM and MeHg, and with a boundary layer-based transfer velocity for Hg0.70 Concentrations of inorganic Hg species were taken from the output of GEOS-Chem; MeHg concentrations in wet deposition were taken as a ratio to concentrations of total Hg as reported in the literature.83 Our predicted wet deposition rates compared favorably with nearby measurements by the Mercury Deposition Network, and our estimates of dry deposition to the watershed were consistent with nearby measured Hg fluxes in litterfall.84,85
The model also requires inputs from the watershed to the lake of Hg species and dissolved organic carbon (DOC). Inputs of DOC are modeled as a sine function to reflect seasonal variability; the mean and amplitude are specific to the lake being modeled. It has been reported in the literature that rates of total Hg runoff from catchments to lakes range from 1–35% of the rate of atmospheric Hg deposition.76,87,88 Multiple models of watershed Hg runoff of varying complexity are available,89,90 but inter-model comparison has shown large discrepancies in predictions.91 It is also clear that there may be considerable delays between deposition to a catchment and runoff to streams and lakes.41,92 The approach of setting the Hg runoff from catchments as proportional to atmospheric deposition72 assumes that only recently deposited Hg is susceptible to leaching; this approach yields a fast lake response to changes in Hg deposition. We use a semi-distributed spatial model that multiplies runoff coefficients for areas of land use categories by annual Hg deposition rates to estimate the maximum possible lake response to changes in atmospheric deposition. This approach allows us to say if it is not possible to reach safe fish concentrations by 2050. Specifically, for divalent Hg we use a runoff coefficient of 10% for “sensitive upland landscapes”, and a value of 5% for less sensitive upland regions. Coefficients of 20% are used for runoff of divalent Hg from wetlands in all areas, and a coefficient of 4.9 is used for MeHg runoff from wetlands.73 Urban and agricultural areas were negligible in the modeled watersheds. Multiple factors (hydrology, flowpaths, soil characteristics) regulate runoff of Hg from upland soils;89,92–94 because it is widely observed that Hg runoff is proportional to runoff or concentrations of OC (both particulate and dissolved),87,89 and because runoff of DOC from forest soils is reported to be high in the UP95 the runoff coefficient for Hg from upland soils was set at 10% of atmospheric deposition rates based on the ratios reported by Babiarz et al.96
Responses of fish Hg concentrations to scenarios of policy change were evaluated, but not to scenarios of change of global climate, land use, and biomass burning. Modeling of atmospheric deposition was performed only for the present and the year 2050. To determine the maximum lake change likely under the chosen scenarios, we ran the lake model for the 2050 conditions. Because the Hg residence time in the modeled lake (excluding fish) is short (∼2 months),70 the lake responds quickly to changes in inputs. While the sediments take longer to reach steady state (20–60 years), low organic matter content of the study lake results in low in-lake methylation and a fast response in this lake to changes in inputs. As mentioned, we do not include the time for Hg to stabilize in the food web, and to estimate the maximum possible lake response we assumed a rapid response of the watershed to alterations in deposition.
2.2.f Virtual experiments: effect of landscape sensitivity.
Fish accumulation of Hg is a response to the interplay of rates of atmospheric deposition with watershed (i.e., landscape sensitivity) and lake characteristics. To highlight the effect of landscape sensitivity on lake response to deposition, we performed two virtual experiments. First, we compared predicted fish Hg concentrations for a UP lake under two regimes of atmospheric deposition (policy-in-action deposition for the UP and Adirondacks). Second, we also changed the watershed conditions to those typical of the Adirondacks area. To clarify the reasons for different lake responses in the two regions, we present a summary of lake and watershed characteristics and fish Hg concentrations in the two locations. The primary data source for fish Hg in the UP was Michigan's Fish Contaminant Monitoring Program (http://www.michigan.gov/deq/0,4561,7-135-3313_3681_3686_3728-32393--,00.html); only concentrations in walleye are summarized here. To eliminate fish size as a cause of variability among lakes, regressions of walleye Hg vs. walleye length were performed for each lake, and concentrations at a common length of 42 cm (the median size of all walleye sampled) were calculated and are reported here. Data sources for water quality included Michigan Surface Water Information Management System (http://www.mcgi.state.mi.us/miswims/), EPA National Lake Assessment (https://www.epa.gov/national-aquatic-resource-surveys/data-national-aquatic-resource-surveys), and a variety of reports and published papers.97–100 Although many data sources are available for the Adirondacks, we used only data presented by Yu et al.24 Data on the abundance of lakes and wetland areas in lake catchments for both regions were gathered from the National Wetland Inventory (https://www.fws.gov/wetlands/Data/State-Downloads), and forest cover was taken from the National Land Cover Database.101
3. Results
3.1 Atmospheric deposition of Hg to the GL region, Michigan's UP, and New York's Adirondacks region in the present vs. 2050
Changes in total (wet + dry) Hg deposition estimated from GEOS-Chem simulations from the present to the future (2050) are summarized in Table 3, along with % contributions to the policy-in-action scenario from the three policy scales (global, U.S., or Lake Superior). Total deposition is estimated to decrease by 70% due to the aspirational policy change scenario in the GL region (Fig. S1†), while it is estimated to decrease by 65% in Michigan's Upper Peninsula (UP) and 78% in the New York Adirondack region. These large estimated decreases are reasonable given that for the aspirational scenario, all anthropogenic emissions in the 2050 simulation were turned off. Because legacy emissions are held constant, this scenario does not represent pre-industrial emissions.
Table 3 Change in total Hg atmospheric deposition from the present to 2050 for the given region and scenario, and % contribution of a given scale region to the policy-in-action scenario
Region |
Scenario |
Great Lakes |
Michigan Upper Peninsula |
New York Adirondacks |
Increase (+) or decrease (−) in total Hg deposition (%) |
% contribution to policy-in-action scenario |
Increase (+) or decrease (−) in total Hg deposition (%) |
% contribution to policy-in-action scenario |
Increase (+) or decrease (−) in total Hg deposition (%) |
% contribution to policy-in-action scenario |
2050 aspirational |
−70 |
|
−65 |
|
−78 |
|
2050 policy-in-action |
−20 |
|
−15 |
|
−29 |
|
World |
|
14 |
|
25 |
|
0.1 |
U.S. |
|
85 |
|
70 |
|
97 |
Lake Superior |
|
1 |
|
5 |
|
2.9 |
2050 minimal-regulation |
+35 |
|
+34 |
|
|
|
2050 climate change |
+3.8 |
|
+5.2 |
|
|
|
2050 land use change |
−0.2 |
|
+0.7 |
|
|
|
2050 biomass burning |
+1.9 |
|
+2.3 |
|
|
|
The combined policy-in-action scenario leads to 20%, 15%, and 29% decreases in atmospheric deposition in 2050 in the GL, Michigan's UP, and New York Adirondack regions, respectively (Table 3). Fig. 2 presents the effects of individual scales of policies (Lake Superior, U.S., and global) on the combined policy-in-action deposition benefit for the GL region. Approximately 85% of the total decrease is due to U.S. Clean Air Act regulations including MATS, while the local (Lake Superior Basin) and global (Minamata Convention) policies each account for 1% and 14% of the total decrease, respectively. Fig. 2B demonstrates the effect of reducing U.S. emissions on GL regional deposition. The two times greater reduction in deposition in the Adirondacks compared to the UP for this scenario is therefore largely related to differences in the influence of U.S. policies.
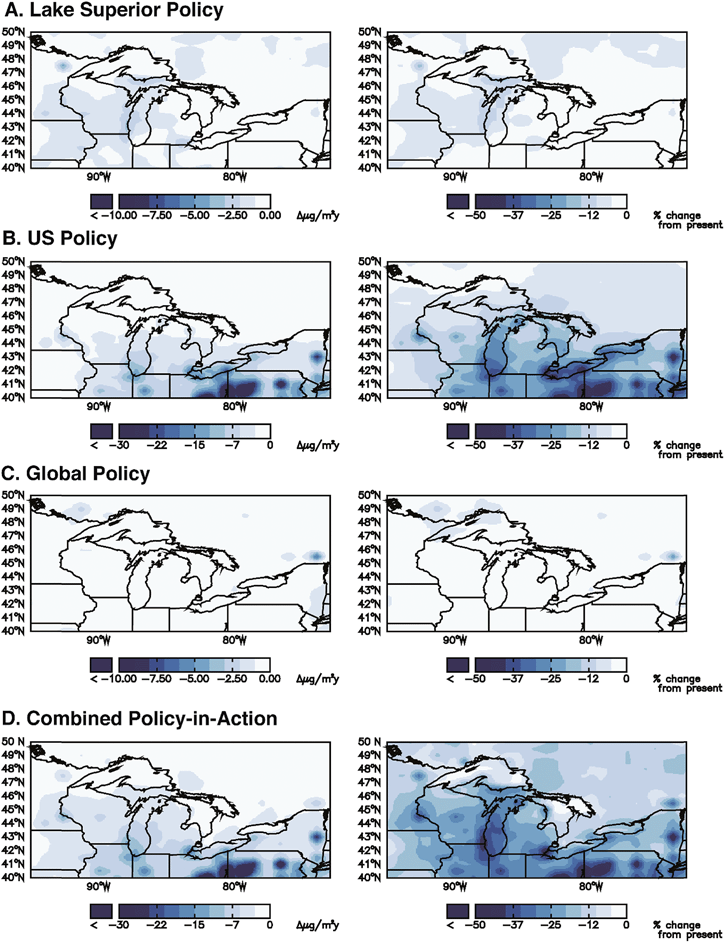 |
| Fig. 2 Lake Superior (A), U.S. (B), global (C), and combined (D) policy total Hg deposition benefits from present day. The panels on the left represent the policy benefits in Δμg per m2 per year, while those on the right represent them as % change from the present. | |
Compared to the GL region policy-in-action scenario contributions of 14% from global policies, 85% from U.S., and 1% from Lake Superior, the UP receives 25% of total deposition decrease from global sources, 70% from the U.S., and 5% from Lake Superior (Table 3). In the Adirondacks region, approximately 97% of the total decrease in deposition for this scenario is due to Clean Air Act regulations in the U.S., while local (Lake Superior Basin) and global (Minamata Convention) each account for 2.9% and 0.1% of the total decrease, respectively. These values clearly show the large differences in contributions that proximity to up-wind sources make. The global contribution (as a percentage) of emissions reductions to decreased atmospheric deposition is over two orders of magnitude greater for the UP than for the Adirondacks (Table 3). Sources within the U.S. (mostly coal-fired power plants) provide a greater fraction of the deposition to the Adirondacks as compared to the UP.
The minimal-regulation scenario leads to increases in total deposition of 35% and 34% in the GL (Fig. S2†) and Michigan's UP region, respectively (Table 3). The similarity in these estimates is likely coincidental. Based on the policy-in-action scenario, differences in deposition benefit are largely related to U.S.-scale changes in emissions, and the largely U.S. emissions that comprise the minimal-regulation total deposition estimates happen to lead to similar increases in deposition.
The climate change, land use/land cover change, and biomass burning scenarios lead to smaller total Hg deposition changes in 2050 as compared to the policy scenarios (Table 3). In the GL region, these estimates are 3.8% (Fig. S3†), ∼0% (Fig. S4†), and 1.9% (Fig. S5†), respectively, whereas for Michigan's UP, the changes are 5.2%, ∼0%, and 2.3%, respectively. The climate change increase is primarily due to an increase in Hg(0) dry deposition and total wet deposition fluxes. The negligibly small land use/land cover changes are a result of both increases and decreases in land cover in 2050 that influence the surfaces to which Hg deposits (Fig. S4†). The increase in deposition from biomass burning is a result of higher biomass burning emissions in the boreal parts of North America and Western U.S. These increases are, in turn, a result of greater fire activity caused by more vegetation availability and a warmer climate in 2050.
3.2 Modeled responses of fish MeHg concentrations to changes in deposition and watershed characteristics
The lake Hg cycling model was validated with measured mercury concentrations in Torch Lake.70 Measured Hg concentrations in Torch Lake water and sediments were used in calibrating the model, and the more numerous measurements of fish Hg concentrations were used as validation. Hg concentrations in brown bullhead, walleye, northern pike and smallmouth bass have been measured approximately every six years since 1988.102 Model predictions for both mixed feeders and piscivorous fish overlapped with the range of measured concentrations in these fish groups, although predictions underestimated mean fish concentrations. The model does not explicitly account for fish size; rather, it predicts concentrations for fish with a distribution of sizes about an invariant mean.
In the UP lake, modeled fish Hg content decreases in proportion to changes in atmospheric deposition. The aspirational scenario reduced fish Hg content by 65%, and the policy-in-action scenario led to an 11% decrease (Fig. 3). These responses are lake-specific and result from the combination of atmospheric deposition, in-lake processes, and catchment characteristics. If policy-in-action rates of atmospheric deposition from the Adirondacks area are applied, the model predicts fish concentrations 34% higher than those with UP deposition rates for the same scenario. If, in addition to Adirondacks deposition rates, watershed characteristics are changed to values representative of a landscape less sensitive than the UP (i.e., reduced wetland area by 38%, reduced runoff from upland soils from 10% of deposition to 5%), then fish Hg concentrations are predicted to be only 70% of the original prediction for UP conditions. In other words, the effects of varying landscape characteristics within the GL region are larger than the effects of the variable rates of atmospheric deposition found in this region.
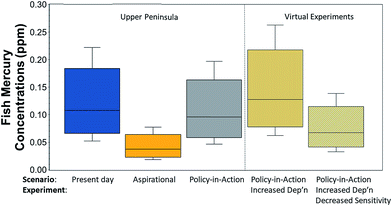 |
| Fig. 3 Predicted Hg concentrations in piscivorous fish in the UP lake under three policy scenarios and two virtual experiments. The aspirational scenario decreases fish Hg by 65% while the policy-in-action scenario leads to an 11% decrease. The first virtual experiment increases deposition over the policy-in-action scenario by 32% and fish Hg increases by 35%. The second virtual experiment maintains the high deposition rate but considers a less sensitive watershed (fewer wetlands, less runoff from forests); fish Hg is only 70% of the policy-in-action scenario with UP conditions. | |
3.3 Spatial distribution of Hg in Michigan's UP lakes and walleye
If the 74 lakes sampled for walleye Hg are representative of the ∼15
000 UP lakes,103 the survey results (Fig. 4) suggest that 77% of UP lakes are impaired with respect to EPA's fish Hg criterion (0.3 ppm wet wt). The percentage of impaired lakes in the UP is considerably above the national average of 49% (ref. 104) and above the 23% reported as impaired in the Adirondacks.24 Possibly as a result of historical mining activities,105 concentrations of Hg in walleye are higher in western than in eastern UP lakes (t-test, p = 0.037), although fewer lakes in the east were sampled than in the west. Multiple linear regression and principal component analyses indicated that fish Hg concentrations are best predicted by watershed area and trophic state for large (>3 km2) lakes, and by pH, wetland area in catchment, lake area, maximum depth and total phosphorus for small lakes.106
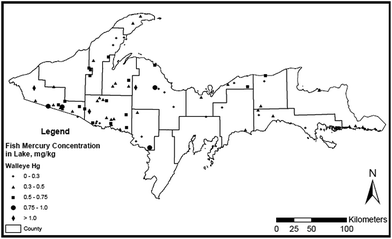 |
| Fig. 4 Spatial distribution of walleye Hg concentrations in Michigan's UP. Depicted are all 74 lakes for which data were located. Concentrations in each lake were calculated for walleye of 42 cm length. | |
4. Discussion
4.1 Regional nature of deposition responses to policy changes
Mapping of the projected changes in deposition due to environmental changes (climate, land use/land cover, biomass burning) and policy clearly shows that even within the GL region there is spatial variability (Fig. 2 and Table 3). Areas in close proximity to upwind sources show a larger response to controls of those sources than do areas far from sources. In all locations, the majority of the simulated decrease in deposition is due to decreased Hg(II) deposition rather than Hg(0) deposition; for the GL region as a whole, 75% of the decrease is due to Hg(II) and 25% due to Hg(0). However, in the Adirondacks, 80% of the decrease is due to Hg(II) while in the UP 70% is due to Hg(II). This projected outcome results from the short atmospheric residence time of Hg(II) emissions (0.5–2 days15) and does not reflect regional differences in availability of oxidants for Hg(0).16 The projected large decrease in Hg(II) deposition is at odds with the absence of a trend in wet deposition of Hg but an observed decrease in litterfall Hg fluxes in the Adirondacks37 that are postulated to result from recent declines in Hg(0) deposition. According to our scenario modeling, those areas currently receiving the highest rates of atmospheric deposition show the largest percentage decreases in deposition due to enactment of existing policies in the U.S. In contrast, the UP is not downwind of major Hg emission sources. U.S. policy-in-action changes in emissions still represent the majority of changes in deposition in the UP (70%), but the rest of the world contributes 1.8 times more to UP deposition than to the GL region, and 250 times more than to the Adirondacks.
One implication of these regional differences in trajectories of Hg deposition is that it will be easiest to detect system responses to policy in those regions projected to show the largest responses. This study suggests that it will be difficult to detect policy-related changes in lake monitoring data in Michigan's UP over the next 50 years, unless additional policy steps are taken beyond those included in the scenarios of this project. Similarly, because the predicted changes in deposition due to climate- and land-use changes are small relative to those associated with policy changes, the likelihood is small that those changes can be detected in the GL region unless anthropogenic emissions stabilize. Within the GL region, changes will be easiest to detect in northern Illinois, Indiana, Ohio and Pennsylvania (Fig. 2).
4.2 Local nature of lake sensitivity
Surveys23,24,107,108 have shown that lakes in certain geographic areas are more susceptible to having high MeHg concentrations in fish. This sensitivity is manifested in the geographic distribution of state-wide fish consumption advisories for Hg in the U.S.;109 each U.S. state bordering the GL or Canada has a state-wide advisory although many of these states have rates of atmospheric deposition lower than states to the south.20 Factors responsible for this sensitivity have been shown to include prevalence of forests and wetlands in the catchment, low alkalinity, low pH, high sulfate loading, low nutrients and long water residence times.23,107,110 Importantly, the susceptibility of lakes to having high fish MeHg content is determined more by factors leading to production of MeHg than by the total supply of Hg to lakes,111 although factors affecting accumulation of MeHg in biota (e.g., fish growth rate, length of food web, OC content of sediments) are also important.110,112
This study suggests that Michigan's UP is a sensitive landscape that readily converts atmospherically deposited Hg into MeHg that is bioaccumulated in fish. Among 74 lakes for which walleye Hg concentrations are available, 77% had concentrations above the U.S. EPA criterion value of 0.3 ppm, and the median concentration was 0.43 ppm.109 Comparisons among regions are difficult because of different fish species present, different sampling strategies, and different analytical protocols. Yu et al.24 measured Hg concentrations in multiple fish species (not including walleye but including large- and small-mouth bass, fish of comparable trophic level) from 44 Adirondack lakes, a region repeatedly called a biological hotspot for Hg.23,24,113 They found that in only 10 of 44 lakes were fish found with concentrations above 0.3 ppm, and in less than 10% of lakes were fish found with concentrations above 0.42 ppm, the median value for UP walleye. This comparison supports the assertion that Michigan's UP is a highly sensitive landscape with respect to promoting Hg bioaccumulation in fish.
The virtual experiments, performed to quantify the magnitude of the landscape effect, suggest that landscape sensitivity differences within the GL region may cause larger differences in fish Hg than do the variations in rates of atmospheric Hg deposition. The virtual experiments kept conditions within the lake (Hg process rates, pH, alkalinity, sulfate, etc.) the same, but added sequentially an increase in atmospheric deposition (32%) followed by a decrease in landscape sensitivity (reduced wetland area, reduced Hg runoff from forests). As expected, an increase in atmospheric deposition increased fish Hg concentrations (35%); the similarity in increases in deposition rates and fish Hg concentrations reflects the lake and watershed conditions used. Importantly, when the landscape sensitivity is changed, the fish Hg drops more than enough to counter the increased atmospheric deposition. The magnitude of the drop again reflects the particular conditions that were modeled (watershed
:
lake area ratio, wetland areas, runoff coefficients used), but the conditions were selected to be representative of areas within the GL region.
The factor rendering UP lakes vulnerable to high bioaccumulation of MeHg is primarily the abundance of wetlands. In the UP, 29% of the land area is occupied by wetlands; among 85 lakes, the median percentage of the catchment occupied by wetlands was 18%. Wetlands are important sites of Hg methylation; the MeHg may then be transported to lakes.114,115 Wetlands also export DOC to lakes; the DOC can bind with and transport inorganic Hg to lakes.114 UP lakes are characterized by relatively high DOC; among 61 UP lakes, the median DOC was 7.7 mg L−1. High DOC in lakes results in reduced light penetration that can reduce algal production, and microbial degradation of DOC can consume oxygen in lake hypolimnia thereby rendering them suitable for Hg methylation. It is instructive to compare characteristics promoting Hg bioaccumulation between the UP and the Adirondacks (Table 4); data for 44 Adirondack lakes were taken from Yu et al.24 The sampled lakes in both areas are similar in having largely forested catchments, and being of comparable size. The Adirondack lakes have low pH as expected in low alkalinity waters at high elevations that receive significant acid deposition. The UP lakes have high DOC, consistent with the high percentage of wetlands in the catchments, and higher chlorophyll. Yu et al. concluded that low pH and low alkalinity were the most prominent factors contributing to Hg bioaccumulation in the Adirondack lakes. Based on comparison with the Adirondack lakes, these factors do not appear to be the controlling factors in the sampled UP lakes; rather, the UP lakes are distinguished by having higher DOC and higher wetland areas.
Table 4 Comparison of features affecting Hg bioaccumulation in UP and Adirondack lakesa
Parameter |
UP lakes |
Adirondack lakesb |
Reported values are the median followed by the standard deviation and number of lakes in parentheses. Forest area is region-wide.
Data from Yu et al.24
|
Non-wetland forest area (%)101 |
77% |
74% |
Wetland in catchment (%) |
18% (12%, 85) |
11% |
DOC (mg L−1) |
7.7 (4.9, 61) |
3.9 (1.4, 44) |
PH |
7.5 (0.7, 99) |
6.6 (0.6, 44) |
Alkalinity (μeq L−1) |
854 (820, 146) |
86 (82, 44) |
Chlorophyll a (mg m−3) |
3.7 (4.8, 105) |
2.0 (3.0, 44) |
THg (ng L−1) |
0.8 (2.5, 61) |
1.5 (0.9, 44) |
Lake area (ha) |
116 (795, 117) |
132 (481, 44) |
The UP lakes will not “recover” to the point that Indigenous peoples can safely consume fish in the quantities desired by 2050. Even low rates of atmospheric Hg deposition will lead to high bioaccumulation in fish. The modeled lake does not approach the target concentration of <0.015 ppm under any scenario. The aspirational scenario reduced Hg deposition approximately three-fold, nearly the magnitude of the increase commonly observed in lake sediments since pre-industrial times.116,117 However, our modeling approach used for the aspirational scenario would not have brought Hg deposition down to pre-industrial levels, because of the continuing influence of legacy emissions. The insensitivity of the UP to the policy-in-action scenario (15% decrease in deposition) indicates that this region will require more aggressive policies to achieve significant reductions in deposition. The combination of the sensitive landscape and the insensitivity of Michigan's UP to current policies are likely to considerably prolong the time required to see substantial decreases in fish Hg.
4.3 Implications for Indigenous communities
These research results are particularly important for Indigenous communities that rely on harvesting and consuming fish as a means of maintaining cultural identity and socio-cultural well-being; the fish harvest has been sustaining the original GL people for nearly two millennia.118 The sensitivity of the UP landscape to increased Hg deposition, methylation, and MeHg bioaccumulation is further confounded by the sensitivity of the population. The presence of other airborne bioaccumulative toxics in fish further compounds the problem. Native American tribes have some of the highest documented fish consumption rates in the U.S., with GL Indigenous populations currently consuming MeHg at rates that are well above human health criteria.118,119 For the KBIC in particular, more than seventy-five-percent of tribal members presently report fish as a primary source of subsistence.118 Subsistence harvesting is a political right. As the oldest and largest federally-recognized Indian tribe in Michigan, the KBIC retains treaty-protected homelands and harvesting rights across ten-million acres of the Lake Superior watershed, including the majority of the western UP118,120,121 (the KBIC is one of thirty-six Anishinaabe tribes that retain harvest treaty rights throughout the GL region).
Harvesting fish is not only considered a treaty right, but also an inherent right. That is, harvesting from the region's waters is integral to maintaining Anishinaabe identity rooted in a traditional way-of-life. Contamination of the fish threatens this way-of-life and also compromises food sovereignty – an issue that is common for Indigenous communities throughout the world, but particularly salient for fishing communities.122 Fish consumption advisories for Hg are widely disseminated in the GL region, but advisories are minimally effective for populations that are most dependent on fish.4–8 The socio-cultural reliance and dependence on fish often leads to a lack of adherence to advisory recommendations where Indigenous fishing communities are put in a very difficult position. To forgo harvesting and fish consumption practices suppresses important cultural norms and prevents important knowledge transmission to future generations, yet consuming fish places individuals at increased risk of physical harm. As a result, many Anishinaabe people feel that they cannot, or will not, adhere to advisory recommendations – it is in essence a ‘false choice’.
Consumption advisories have been used to address the problem of fish contamination for almost five decades in the U.S., however, it is important to know that these advisories were only meant to be short-term means to manage fish contamination.119 Initially, within the GL there was an assumption that Hg sources were regional and that regional policy could reduce and prevent Hg contamination to a point that advisories would no longer be necessary. We now know that atmospheric sources of Hg to the UP contain a significant fraction (25%) of global source, and, based on the modeling results presented here, policy oriented toward the Lake Superior Basin will only be minimally effective in achieving safe fish for vulnerable populations in the UP. Although it is important to consider implications for vulnerable populations in landscape sensitivity assessments, we must also emphasize the socio-cultural and political factors that significantly increase the magnitude of Hg contamination consequences and Hg reduction policy required at differing scales to address them. The ‘fixity to place’ (i.e., inability to relocate) that many Indigenous peoples have to their homelands, both because of long-term connections and reserved treaty rights, requires a Hg policy approach that considers the spatial distribution of vulnerable populations worldwide.123,124
Multiple limitations remain in our ability to model lake responses to changes in Hg emissions. The scenarios used here do not include potential longer-term changes in legacy emissions of Hg; hence our predictions overestimate the real-world rate of response to changes in anthropogenic emissions, and omit the impact of legacy emissions occurring between 2000 and 2050.125,126 Legacy emissions of Hg to surface waters also were not included in our modeling effort; work elsewhere indicates that such emissions prolong the time to recovery by decades.45–47 Application of more complicated watershed models91 will be required to predict the timeframe of watershed responses to changes in deposition. The lake Hg model can only crudely predict responses to changes in lake pH; such changes are likely in response to changes in atmospheric deposition of acids and sulfate and to changes in DOC inputs to lakes.127,128 The model does not yet include sulfate deposition as a driver of Hg methylation in wetlands or in lakes.35,42,129 All of these current shortcomings, however, do not negate our prediction that recovery will not occur in response to the policy scenarios considered here; they do prevent us from predicting the timeframe for recovery.
5. Conclusions
The approach for assessing the effects of policy on safety of fish consumption developed in this project provided a means to use state-of-the-art science tools (rigorous modeling using carefully crafted scenario analysis) to answer a community-relevant question. While uncertainties in atmospheric, catchment, and lake models preclude an explicit prediction of recovery times for lakes, the conclusion that recovery will not follow quickly after implementation of the policies modeled in this study is robust. Our application of this approach to the GL region points out that recovery from Hg contamination is spatially variable. Regions such as Michigan's UP that are both highly sensitive to inputs from atmospheric deposition and not close to the emission sources of that deposition will require careful policy development to recover from current levels of contamination.
Author contribution
J. Perlinger contributed to the writing of the manuscript and its review and revisions. N. Urban conducted lake modeling, contributed to the writing of the manuscript, and its review and revision. A. Giang conducted GEOS-Chem modeling of policy scenarios and contributed to the Experimental section. N. Selin contributed to GEOS-Chem modeling of policy scenarios, and reviewed and revised the manuscript. A. Hendricks conducted lake modeling. H. Zhang conducted GEOS-Chem modeling of climate and land use/land cover scenarios and contributed to the Experimental section. A. Kumar conducted GEOS-Chem modeling of the biomass burning scenario and contributed to the Experimental section. S. Wu contributed to GEOS-Chem modeling related to the climate, land use, land cover, and biomass burning scenarios and reviewed the Experimental section. V. Gagnon conducted university-community research and engagement, and contributed to writing the manuscript, and its review and revision. H. Gorman conducted social science research and contributed to writing the manuscript. E. Norman conducted social science research and contributed to writing the manuscript.
Conflicts of interest
There are no conflicts to declare.
Acknowledgements
The authors acknowledge the valuable contributions of the anonymous reviewers to this paper. The authors also acknowledge the Keweenaw Bay Indian Community, with particular gratitude to the Tribal Council, the Natural Resources Department, and the Ojibwa Community College for sharing knowledge and expertise with our research team throughout the project. This research was funded by the U.S. National Science Foundation through Grant #ICER-1313755.
References
-
U. S. EPA, Great Lakes Fish Monitoring and Surveillance Program: Background, Trends, and General Conclusions, accessed September 9, 2017, http://https://www.epa.gov/great-lakes-monitoring/great-lakes-fish-monitoring-and-surveillance Search PubMed.
-
U. S. EPA, National Listing of Fish Advisories, Fish and Shellfish Advisories and Safe Eating Guidelines, accessed September 9, 2017, http://https://www.epa.gov/choose-fish-and-shellfish-wisely/fish-and-shellfish-advisories-and-safe-eating-guidelines Search PubMed.
-
GLIFWC, Guidance for Safe Consumption of Walleye from Inland Lakes within the Ceded Territories of Wisconsin, Michigan, and Minnesota, http://www.glifwc.org/Mercury/mercury.html, accessed September 9, 2017 Search PubMed.
- J. Cassady, Med. Anthropol. Q., 2010, 24, 451–471 CrossRef PubMed.
- J. Donatuto and B. Harper, Risk Anal., 2008, 28, 1497–1506 CrossRef PubMed.
- J. Donatuto, T. Satterfield and R. Gregory, Health Risk Soc., 2011, 13, 103–127 CrossRef.
- C. A. O'Neill, J. Environ. Law Litigation, 2007, 22, 131–151 Search PubMed.
- D. J. Ranco and D. Suagee, Antipode, 2007, 39, 691–707 CrossRef.
- V. S. Gagnon, Water History, 2016, 8, 365–384 CrossRef.
- E. Hoover, Ecol. Process, 2013, 2, 4 CrossRef PubMed.
- E. S. Norman, Area, 2013, 45, 179–187 CrossRef.
- D. J. Ranco, C. A. O'Neill, J. Donatuto and B. Harper, Environ. Justice, 2011, 4, 221–230 CrossRef.
-
UNEP, Minamata Convention on Mercury, http://www.mercuryconvention.org/Portals/11/documents/Booklets/Minamata%20Convention%20on%20Mercury_booklet_English.pdf, accessed November 13, 2017 Search PubMed.
- V. S. Gagnon, H. S. Gorman and E. S. Norman, Gateways: International Journal of Community Research and Engagement, 2017, 10, 164–184 CrossRef.
- C. T. Driscoll, Y. J. Han, C. Y. Chen, D. C. Evers, K. F. Lambert, T. M. Holsen, N. C. Kamman and R. K. Munson, Bioscience, 2007, 57, 17–28 CrossRef.
- H. M. Horowitz, D. J. Jacob, Y. X. Zhang, T. S. Dibble, F. Slemr, H. M. Amos, J. A. Schmidt, E. S. Corbitt, E. A. Marais and E. M. Sunderland, Atmos. Chem. Phys., 2017, 17, 6353–6371 CrossRef CAS.
- W. Seiler, C. Eberling and F. Slemr, Pure Appl. Geophys., 1980, 118, 964–974 CrossRef CAS.
- E. A. Nater and D. F. Grigal, Nature, 1992, 358, 139–141 CrossRef CAS.
- C. Seigneur, K. Vijayaraghavan, K. Lohman, P. Karamchandani and C. Scott, Environ. Sci. Technol., 2004, 38, 555–569 CrossRef CAS PubMed.
- N. E. Selin and D. J. Jacob, Atmos. Environ., 2008, 42, 5193–5204 CrossRef CAS.
- M. D. Cohen, R. R. Draxler, R. S. Artz, P. Blanchard, M. S. Gustin, Y. J. Han, T. M. Holsen, D. Jaffe, P. Kelley, H. Lei, C. P. Loughner, W. T. Luke, S. N. Lyman, D. Niemi, J. M. Pacyna, M. Pilote, L. Poissant, D. Ratte, X. Ren, F. Steenhuisen, A. Steffen, R. Tordon and S. J. Wilson, Elementa, 2016 DOI:10.12952/journal.elementa.000118.
- H. Zhou, C. L. Zhou, M. M. Lynam, J. T. Dvonch, J. A. Barres, P. K. Hopke, M. Cohen and T. M. Holsen, Environ. Sci. Technol. Lett., 2017, 4, 91–97 CrossRef CAS.
- D. C. Evers, Y. J. Han, C. T. Driscoll, N. C. Kamman, M. W. Goodale, K. F. Lambert, T. M. Holsen, C. Y. Chen, T. A. Clair and T. Butler, BioScience, 2007, 57, 29–43 CrossRef.
- X. Yu, C. T. Driscoll, M. R. Montesdeoca, D. Evers, M. Duron, K. Williams, N. Schoch and N. Kamman, Ecotoxicology, 2011, 20, 1543–1554 CrossRef CAS PubMed.
- D. C. Depew, N. M. Burgess and L. M. Campbell, Environ. Pollut., 2013, 176, 234–243 CrossRef CAS PubMed.
- K. R. Rolfhus, J. G. Wiener, R. J. Haro, M. B. Sandheinrich, S. W. Bailey and B. R. Seitz, Sci. Total Environ., 2015, 514, 192–201 CrossRef CAS PubMed.
- C. D. Evans, P. J. Chapman, J. M. Clark, D. T. Monteith and M. S. Cresser, Global Change Biol., 2006, 12, 2044–2053 CrossRef.
- M. Erlandsson, N. Cory, S. Kohler and K. Bishop, J. Geophys. Res.: Biogeosci., 2010, 115, G03004 Search PubMed.
-
N. R. Urban, E. S. Verry, S. J. Eisenreich, D. F. Grigal and S. Sebestyen, Element cycling in upland/peatland watersheds, Peatland Biogeochemistry and Watershed Hydrology at the Marcell Experimental Forest, ed. R. K. Kolka, S. Sebestyen, E. S. Verry and K. Brooks, CRC Press, Boca Raton, FL, 2011, pp. 213–241 Search PubMed.
- L. Lindestrom, Ambio, 2001, 30, 538–544 CrossRef CAS PubMed.
- M. J. Parsons, D. T. Long and S. S. Yohn, Appl. Geochem., 2010, 25, 1676–1687 CrossRef CAS.
- R. C. Harris, J. W. M. Rudd, M. Amyot, C. L. Babiarz, K. G. Beaty, P. J. Blanchfield, R. A. Bodaly, B. A. Branfireun, C. C. Gilmour, J. A. Graydon, A. Heyes, H. Hintelmann, J. P. Hurley, C. A. Kelly, D. P. Krabbenhoft, S. E. Lindberg, R. P. Mason, M. J. Paterson, C. L. Podemski, A. Robinson, K. A. Sandilands, G. R. Southworth, V. L. S. Louis and M. T. Tate, Proc. Natl. Acad. Sci. U. S. A., 2007, 104, 16586–16591 CrossRef CAS PubMed.
- C. J. Watras, K. A. Morrison, R. J. M. Hudson, T. M. Frost and T. K. Kratz, Environ. Sci. Technol., 2000, 34, 4051–4057 CrossRef CAS.
- T. R. Hrabik and C. J. Watras, Sci. Total Environ., 2002, 297, 229–237 CrossRef CAS PubMed.
- P. E. Drevnick, D. E. Canfield, P. R. Gorski, A. L. C. Shinneman, D. R. Engstrom, D. C. G. Muir, G. R. Smith, P. J. Garrison, L. B. Cleckner, J. P. Hurley, R. B. Noble, R. R. Otter and J. T. Oris, Environ. Sci. Technol., 2007, 41, 7266–7272 CrossRef CAS PubMed.
- N. Gandhi, R. W. K. Tang, S. P. Bhavsar and G. B. Arhonditsis, Environ. Sci. Technol., 2014, 48, 5404–5414 CrossRef CAS PubMed.
- J. R. Gerson and C. T. Driscoll, Environ. Sci. Technol., 2016, 50, 10943–10950 CrossRef CAS PubMed.
- C. D. Knightes, E. M. Sunderland, M. Craig Barber, J. M. Johnston and R. B. Ambrose, Environ. Toxicol. Chem., 2009, 28, 881–893 CAS.
- C. D. Knightes and R. B. Ambrose, Environ. Toxicol. Chem., 2007, 26, 807–815 CrossRef CAS PubMed.
- N. E. Selin, E. M. Sunderland, C. D. Knightes and R. A. Mason, Environ. Health Perspect., 2010, 118, 137–143 CAS.
- J. Munthe, R. A. Bodaly, B. A. Branfireun, C. T. Driscoll, C. C. Gilmour, R. Harris, M. Horvat, M. Lucotte and O. Malm, Ambio, 2007, 36, 33–44 CrossRef CAS PubMed.
- C. J. Watras and K. A. Morrison, Can. J. Fish. Aquat. Sci., 2008, 65, 100–116 CrossRef CAS.
-
MDEQ, Michigan's Water Chemistry Monitoring Program: A Report of Statewide Spatial Patterns 2005-2009 and Fixed Station Status and Trends 1998-2008, W. R. Division Report MI/DEQ/WRD-13/005, Michigan Department of Environmental Quality, Lansing, MI, 2013 Search PubMed.
- H. D. Yang, S. Turner and N. L. Rose, Environ. Pollut., 2016, 219, 1092–1101 CrossRef CAS PubMed.
- M. Meili, K. Bishop, L. Bringmark, K. Johansson, J. Munthe, H. Sverdrup and W. de Vries, Sci. Total Environ., 2003, 304, 83–106 CrossRef CAS PubMed.
- L. Hakanson, Ecol. Model., 1996, 93, 251–262 CrossRef CAS.
- K. Vijayaraghavan, L. Levin, L. Parker, G. Yarwood and D. Streets, Environ. Toxicol. Chem., 2014, 33, 1238–1247 CrossRef CAS PubMed.
- National listing of Fish Advisories, Fish and Shellfish Advisories and safe eating guidelines, viewed November 2016, http://https://www.epa.gov/choose-fish-and-shellfish-wisely/fish-and-shellfish-advisories-and-safe-eating-guidelines, accessed November 13 2017.
- E. Oken, R. O. Wright, K. P. Kleinman, D. Bellinger, C. J. Amarasiriwardena, H. Hu, J. W. Rich-Edwards and M. W. Gillman, Environ. Health Perspect., 2005, 113, 1376–1380 CrossRef CAS PubMed.
-
E. Groth, An Overview of Epidemiological Evidence on the Effects of Methylmercury on Brain Development, and A Rationale for a Lower Definition of Tolerable Exposure Zero Mercury Working Group, Brussels, Belgium, 2012 Search PubMed.
- A. Giang and N. E. Selin, Proc. Natl. Acad. Sci. U. S. A., 2016, 113, 286–291 CrossRef CAS PubMed.
- S. Wu, L. J. Mickley, D. J. Jacob, J. A. Logan, R. M. Yantosca and D. Rind, J. Geophys. Res., 2007, 112, D05302 Search PubMed.
- X. M. Zhang, K. R. Rygwelski, M. D. Rowe, R. Rossmann and R. G. Kreis, J. Great Lakes Res., 2016, 42, 62–69 CrossRef CAS.
- S. Wu, L. J. Mickley, J. O. Kaplan and D. J. Jacob, Atmos. Chem. Phys., 2012, 12, 1597–1609 CrossRef CAS.
- H. Zhang, C. D. Holmes and S. Wu, Atmos. Environ., 2016, 141, 230–244 CrossRef CAS.
- Y. X. Huang, S. L. Wu and J. O. Kaplan, Atmos. Environ., 2015, 121, 86–92 CrossRef CAS.
- A. Kumar, S. Wu, Y. Huang, H. Liao and J. Kaplan, Atmos. Environ., 2017, 173, 6–15 CrossRef.
- E. S. Corbitt, D. J. Jacob, C. D. Holmes, D. G. Streets and E. M. Sunderland, Environ. Sci. Technol., 2011, 45, 10477–10484 CrossRef CAS PubMed.
- Y. Zhang, L. Jaegle, A. van Donkelaar, R. V. Martin, C. D. Holmes, H. M. Amos, Q. Wang, R. Talbot, R. Artz, S. Brooks, W. Luke, T. M. Holsen, D. Felton, E. K. Miller, K. D. Perry, D. Schmeltz, A. Steffen, R. Tordon, P. Weiss-Penzias and R. Zsolway, Atmos. Chem. Phys., 2012, 12, 6095–6111 CrossRef CAS.
- D. G. Streets, Q. Zhang and Y. Wu, Environ. Sci. Technol., 2009, 43, 2983–2988 CrossRef CAS PubMed.
- P. Rafaj, I. Bertok, J. Cofala and W. Schopp, Atmos. Environ., 2013, 79, 472–479 CrossRef CAS.
- N. E. Selin, Environ. Toxicol. Chem., 2014, 33, 1202–1210 CrossRef CAS PubMed.
- J. M. Pacyna, O. Travnikov, F. De Simone, I. M. Hedgecock, K. Sundseth, E. G. Pacyna, F. Steenhuisen, N. Pirrone, J. Munthe and K. Kindbom, Atmos. Chem. Phys., 2016, 16, 12495–12511 CAS.
-
Lake Superior Binational Program, Lake Superior Zero Discharge Demonstration Program and Critical Chemical Reduction Milestones, 2017, https://www.epa.gov/sites/production/files/2015-11/documents/lake-superior-zero-discharge-demonstration-program-2012-8pp.pdf, accessed November 13, 2017 Search PubMed.
- H. M. Amos, D. J. Jacob, C. D. Holmes, J. A. Fisher, Q. Wang, R. M. Yantosca, E. S. Corbitt, E. Galarneau, A. P. Rutter, M. S. Gustin, A. Steffen, J. J. Schauer, J. A. Graydon, V. L. St Louis, R. W. Talbot, E. S. Edgerton, Y. Zhang and E. M. Sunderland, Atmos. Chem. Phys., 2012, 12, 591–603 CrossRef CAS.
- C. D. Holmes, D. J. Jacob, E. S. Corbitt, J. Mao, X. Yang, R. Talbot and F. Slemr, Atmos. Chem. Phys., 2010, 10, 12037–12057 CrossRef CAS.
- N. E. Selin, D. J. Jacob, R. M. Yantosca, S. Strode, L. Jaegle and E. M. Sunderland, Global Biogeochem. Cycles, 2008, 22, GB 2011 Search PubMed.
- A. L. Soerensen, E. M. Sunderland, C. D. Holmes, D. J. Jacob, R. M. Yantosca, H. Skov, J. H. Christensen, S. A. Strode and R. P. Mason, Environ. Sci. Technol., 2010, 44, 8574–8580 CrossRef CAS PubMed.
- D. Rind, J. Lerner, J. Jonas and C. McLinden, J. Geophys. Res.: Atmos., 2007, 112, D09315 CrossRef.
- A. N. Hendricks and N. R. Urban, Ecol. Model. Search PubMed , in prep.
- K. Soetaert, T. Petzoldt and R. W. Setzer, J. Stat. Software, 2010, 33, 1–25 Search PubMed.
-
C. D. Knightes and R. B. Ambrose, Development of an Ecological Risk Assessment Methodology for Assessing Wildlife Exposure Risk Associated with Mercury-Contaminated Sediments in Lake and River Systems, Report EPA/600/R-06/073, U.S. Environmental Protection Agency Office of Research and Development, Washington, D.C., 2006 Search PubMed.
- C. D. Knightes, Environ. Model. Software, 2008, 23, 495–510 CrossRef.
- L. Hakanson, Water, Air, Soil Pollut., 2000, 124, 301–317 CrossRef CAS.
- M. C. Barber, Environ. Toxicol. Chem., 2008, 27, 755–777 CAS.
- E. B. Swain, D. R. Engstrom, M. E. Brigham, T. A. Henning and P. L. Brezonik, Science, 1992, 257, 784–787 CAS.
- P. Lorey and C. T. Driscoll, Environ. Sci. Technol., 1999, 33, 718–722 CrossRef CAS.
- P. E. Drevnick, D. R. Engstrom, C. T. Driscoll, E. B. Swain, S. J. Balogh, N. C. Kamman, D. T. Long, D. G. C. Muir, M. J. Parsons, K. R. Rolfhus and R. Rossmann, Environ. Pollut., 2012, 161, 252–260 CrossRef CAS PubMed.
- J. Kwan and P. A. Taylor, Bound.-Layer Meteorol., 1994, 68, 339–356 CrossRef.
- D. E. Anderson, R. G. Striegl, D. I. Stannard, C. M. Michmerhuizen, T. A. McConnaughey and J. W. LaBaugh, Limnol. Oceanogr., 1999, 44, 988–1001 CrossRef CAS.
- T. R. Khan and J. A. Perlinger, Geosci. Model Dev., 2017, 10, 3861–3888 Search PubMed.
- J. S. Read, D. P. Hamilton, A. R. Desai, K. C. Rose, S. MacIntyre, J. D. Lenters, R. L. Smyth, P. C. Hanson, J. J. Cole, P. A. Staehr, J. A. Rusak, D. C. Pierson, J. D. Brookes, A. Laas and C. H. Wu, Geophys. Res. Lett., 2012, 39 DOI:10.1029/2012GL051886.
- B. D. Hall, H. Manolopoulos, J. P. Hurley, J. J. Schauer, V. L. St Louis, D. Kenski, J. Graydon, C. L. Babiarz, L. B. Cleckner and G. J. Keeler, Atmos. Environ., 2005, 39, 7557–7569 CrossRef CAS.
- M. R. Risch, J. F. Dewild, D. A. Gay, L. Zhang, E. W. Boyer and D. P. Krabbenhoft, Environ. Pollut., 2017, 228, 8–18 CrossRef CAS PubMed.
- M. R. Risch, D. A. Gay, K. K. Fowler, G. J. Keeler, S. M. Backus, P. Blanchard, J. A. Barres and J. T. Dvonch, Environ. Pollut., 2012, 161, 261–271 CrossRef CAS PubMed.
-
A. Hendricks, A Model to Predict Concentrations and Uncertainty for Mercury Species in Lakes, M.S. thesis, Michigan Technological University, 2018.
- D. F. Grigal, Environ. Rev., 2002, 10, 1–39 CrossRef CAS.
-
H. Hsu-Kim, C. S. Eckley, D. Acha, X. Feng, C. Gilmour, S. Jonsson and C. P. J. Mitchell, Ambio, in review Search PubMed.
- M. N. Futter, A. E. Poste, D. Butterfield, P. J. Dillon, P. G. Whitehead, A. P. Dastoor and D. R. S. Lean, Sci. Total Environ., 2012, 424, 219–231 CrossRef CAS PubMed.
- H. E. Golden, C. D. Knightes, P. A. Conrads, G. M. Davis, T. D. Feaster, C. A. Journey, S. T. Benedict, M. E. Brigham and P. M. Bradley, J. Geophys. Res.: Biogeosci., 2012, 117, G01006 Search PubMed.
- H. E. Golden, C. D. Knightes, P. A. Conrads, T. D. Feaster, G. M. Davis, S. T. Benedict and P. M. Bradley, Environ. Toxicol. Chem., 2013, 32, 2165–2174 CrossRef CAS PubMed.
- R. B. Mills, A. M. Paterson, J. M. Blais, D. R. S. Lean, J. P. Smol and G. Mierle, Can. J. Fish. Aquat. Sci., 2009, 66, 187–200 CrossRef CAS.
- J. T. Bushey, C. T. Driscoll, M. J. Mitchell, P. Selvendiran and M. R. Montesdeoca, Hydrol. Processes, 2008, 22, 4813–4826 CrossRef CAS.
- R. K. Kolka, D. F. Grigal, E. A. Nater and E. S. Verry, Soil Sci. Soc. Am. J., 2001, 65, 897–905 CrossRef CAS.
- P. C. Frost, J. H. Larson, G. A. Lamberti, C. A. Johnston, K. C. Young, P. A. Maurice and S. D. Bridgham, Aquat. Sci., 2006, 68, 40–51 CrossRef CAS.
- C. Babiarz, S. Hoffmann, A. Wieben, J. Hurley, A. Andren, M. Shafer and D. Armstrong, Environ. Pollut., 2012, 161, 299–310 CrossRef CAS PubMed.
- P. A. Soranno, K. S. Cheruvelil, T. Wagner, K. E. Webster and M. T. Bremigan, PLoS One, 2015, 10(8), e0135454 Search PubMed.
- K. S. Cheruvelil, P. A. Soranno, K. E. Webster and M. T. Bremigan, Ecol. Appl., 2013, 23, 1603–1618 CrossRef CAS PubMed.
-
J. E. Breck, Compilation of Databases on Michigan Lakes MDNR Fisheries Division Lansing, MI, 2004 Search PubMed.
-
D. Knauer, G. Pelkola, S. Casey, D. Krabbenhoft and J. F. Dewild, The distribution of total and methyl mercury in Michigan's Upper Peninsula Lakes, Michigan Dept. Environmental Quality, Lansing, MI, 2011 Search PubMed.
- National Land Cover Database 2011, http://https://www.mrlc.gov/nlcd2011.php, accessed November 13, 2017.
-
MDEQ, Status of Fish Contaminant Levels in the Torch Lake Area of Concern 2013, W. R. D. Michigan Department of Environmental Quality Report MI/DEQ/WRD-16/003, Michigan Dept. Environmental Quality, Lansing, Michigan, 2016 Search PubMed.
- C. P. McDonald, J. A. Rover, E. G. Stets and R. G. Striegl, Limnol. Oceanogr., 2012, 57, 597–606 CrossRef.
- L. L. Stahl, B. D. Snyder, A. R. Olsen and J. L. Pitt, Environ. Monit. Assess., 2009, 150, 3–19 CrossRef CAS PubMed.
- W. C. Kerfoot, N. R. Urban, C. P. McDonald, H. Zhang, R. Rossmann, J. A. Perlinger, T. Khan, A. Hendricks, M. Priyadarshini and M. Bolstad, Environ. Sci.: Processes Impacts, 2018 Search PubMed , in press.
-
M. Priyadarshini, Factors Contributing to Elevated Concentrations of Mercury and PCBs in Fish in the Inland Lakes of Michigan's Upper Peninsula and Lake Superior, M.S. thesis, Michigan Technological University, M.S., 2017.
- C. A. Eagles-Smith, J. T. Ackerman, J. J. Willacker, M. T. Tate, M. A. Lutz, J. A. Fleck, A. R. Stewart, J. G. Wiener, D. C. Evers, J. M. Lepak, J. A. Davis and C. F. Pritz, Sci. Total Environ., 2016, 568, 1171–1184 CrossRef CAS PubMed.
-
D. C. Evers, J. G. Wiener, C. T. Driscoll, D. A. Gay, N. Basu, B. A. Monson, K. F. Lambert, H. A. Morrison, J. T. Morgan, K. A. Williams and A. G. Soehl, Great Lakes Mercury Connections: The Extent and Effects of Mercury Pollution in the Great Lakes Region, Biodiversity Research Institute, Gorham, Maine, 2011 Search PubMed.
- National Listing of Fish Advisories, https://www.epa.gov/sites/production/files/2015-06/documents/maps-and-graphics-2011.pdf, accessed November 13, 2017.
- C. Y. Chen, C. L. Folt, R. S. Stemberger, N. C. Kamman and B. M. Mayes, Ecotoxicology, 2005, 14, 135–147 CrossRef CAS PubMed.
- C. A. Eagles-Smith, J. G. Wiener, C. S. Eckley, J. J. Willacker, D. C. Evers, M. Marvin-DiPasquale, D. Obrist, J. A. Fleck, G. R. Aiken, J. M. Lepak, A. K. Jackson, J. P. Webster, A. R. Stewart, J. A. Davis, C. N. Alpers and J. T. Ackerman, Sci. Total Environ., 2016, 568, 1213–1226 CrossRef CAS PubMed.
- C. Y. Chen, M. Dionne, B. M. Mayes, D. M. Ward, S. Sturup and B. P. Jackson, Environ. Sci. Technol., 2009, 43, 1804–1810 CrossRef CAS PubMed.
- D. C. Evers, R. P. Mason, N. C. Kamman, C. Y. Chen, A. L. Bogomolni, D. L. Taylor, C. R. Hammerschmidt, S. H. Jones, N. M. Burgess, K. Munney and K. C. Parsons, EcoHealth, 2008, 5, 426–441 CrossRef PubMed.
- P. Selvendiran, C. T. Driscoll, J. T. Bushey and M. R. Montesdeoca, Environ. Pollut., 2008, 154, 46–55 CrossRef CAS PubMed.
- P. Selvendiran, C. T. Driscoll, M. R. Montesdeoca and J. T. Bushey, J. Geophys. Res.: Biogeosci., 2008, 113, G00C01 Search PubMed.
- D. R. Engstrom, E. B. Swain, T. A. Henning, M. E. Brigham and P. L. Brezonik, Adv. Chem. Ser., 1993, 237, 33–66 CrossRef.
- D. R. Engstrom, W. F. Fitzgerald, C. A. Cooke, C. H. Lamborg, P. E. Drevnick, E. B. Swain, S. J. Balogh and P. H. Balcom, Environ. Sci. Technol., 2014, 48, 6533–6543 CrossRef CAS PubMed.
-
V. S. Gagnon, P. Nankervis and E. Johnston, Keweenaw Bay Indian Community Wildlife and Natural Resources Report, KBIC Natural Resources Department, Baraga, MI, 2013, http://nrd.kbic-nsn.gov/sites/default/files/KBIC%20WILDLIFE%20and%20NR%20REPORT%2011_2013%20%28hyperlinked%20TABLE%20of%20CONTENTS%29_0.pdf, accessed November 13, 2017 Search PubMed.
-
C. A. O'Neill, Mercury, risk & justice, Environmental Law Institute, Environmental Law Reporter News and Analysis, 2004 Search PubMed.
-
A. McCammon-Soltis and J. S. Kekek, Fulfilling Ojibwe treaty promises—an overview and compendium of relevant cases, statutes and agreement, Great Lakes Indian Fish & Wildlife Commission Division of Intergovernmental Affairs, 2009, http://www.glifwc.org/minwaajimo/Papers/Legal%20Paper%20-%20DIA.pdf, accessed November 13, 2017. Search PubMed.
-
C. F. Wilkinson, Blood Struggle: The Rise of Modern Indian Nations, NY, 2005 Search PubMed.
- E. S. Norman, Soc. Nat. Resour., 2017, 30, 537–553 CrossRef.
- E. S. Norman, Water Alternatives, 2012, 5, 138–160 Search PubMed.
-
E. S. Norman, Governing Transboundary Waters: Canada, the United States, and Indigenous Communities, Routledge, Florence, KY, 2014 Search PubMed.
- H. M. Amos, D. J. Jacob, D. G. Streets and E. M. Sunderland, Global Biogeochem. Cycles, 2013, 27, 410–421 CrossRef CAS.
- M. Jiskra, J. G. Wiederhold, U. Skyllberg, R. M. Kronberg, I. Hajdas and R. Kretzschmar, Environ. Sci. Technol., 2015, 49, 7188–7196 CrossRef CAS PubMed.
- M. Erlandsson, I. Buffam, J. Folster, H. Laudon, J. Temnerud, G. A. Weyhenmeyer and K. Bishop, Global Change Biol., 2008, 14, 1191–1198 CrossRef.
- B. L. Skjelkvale, H. Borg, A. Hindar and A. Wilander, Appl. Geochem., 2007, 22, 1174–1180 CrossRef CAS.
- J. D. Jeremiason, D. R. Engstrom, J. E. Almendinger, E. B. Swain, B. A. Monson, E. A. Nater, B. M. Johnson and R. K. Kolka, Environ. Sci. Technol., 2006, 40, 3800–3806 CrossRef CAS PubMed.
Footnotes |
† Electronic supplementary information (ESI) available. See DOI: 10.1039/c7em00547d |
‡ Currently at the Institute for Resources, Environment and Sustainability and Department of Mechanical Engineering, University of British Columbia, Vancouver, BC V6T 1Z4 |
§ Currently at the Center for Global and Regional Environmental Research, University of Iowa, Iowa City, IA, 52242, USA. |
|
This journal is © The Royal Society of Chemistry 2018 |