DOI:
10.1039/C8DT02916D
(Paper)
Dalton Trans., 2018,
47, 16139-16144
pH-Control as a way to fine-tune the Cu/Cu2O ratio in radiation induced synthesis of Cu2O particles†
Received
17th July 2018
, Accepted 26th October 2018
First published on 29th October 2018
Abstract
In this work we have optimized the γ-radiation induced synthesis of Cu–Cu2O particles from aqueous CuSO4 solution by investigating the effect of pH. The obtained precipitate was analyzed by XRD and SEM techniques. The results indicated that at solution pH lower than 3.75, quasi-spherical Cu agglomerates can be formed while at pH higher than 4.40 only octahedron-shaped Cu2O particles are produced. At solution pH in the range from 3.75 to 4.40, a Cu–Cu2O mixture is produced. It was found that the relative amount of Cu2O in the Cu–Cu2O precipitate increases with pH in the studied range. The influence of solution pH on the Cu/Cu2O ratios in the product can be explained on the basis of pH-dependent competition kinetics between the reactions leading to either Cu or Cu2O formation. As a consequence, the composition and morphology of the Cu–Cu2O precipitate can be tuned by controlling pH of the aqueous CuSO4 solution during the γ-radiation induced synthesis.
Introduction
Metal and metal oxide particles have received increasing attention during the last decades due to their catalytic,1 magnetic,2 conductive3 and optical4 properties. Among these materials, cuprous oxide (Cu2O) has been investigated extensively since it is an important p-type semiconductor with a band gap of 2.2 eV.5–9 Cu2O particles have potential applications in solar energy conversion,10 photocatalysis,5 biosensing11 and organic synthesis.12 The expanding application of Cu2O requires the materials to be produced with well controlled size, shape, morphology and composition. One of the possible routes to synthesis of Cu2O is to precipitate it from solution by changing the redox potential. Kumar et al.13 prepared Cu2O nanocubes with a hierarchical structure by a one-pot synthesis method employing polyethylene glycol and glucose as a structure-directing agent and reductant, respectively. Xu et al.14 synthesized octahedral Cu2O crystal by reducing copper hydroxide with hydrazine. Yu et al.15 prepared CuO/Cu2O composite hollow microspheres with controlled diameter and composition by hydrothermal synthesis using Cu(CH3COO)2·H2O as a precursor.
In the current study, a γ-radiation induced method is used to produce Cu2O particles. The use of ionizing radiation in synthesizing particles has several advantages compared to other methods. Among other things, the method can be used to synthesize both organic and inorganic particles in a process where the use of harmful chemicals can be avoided and the synthesis scheme becomes quite simple.16–20 Gold nanoparticles,21 Palladium catlysts22 and Pt/CNTs catalysts23 have been produced by γ-radiation induced synthesis methods. Though most of the products were noble metal particles, there were some studies about preparing metal oxides by this method, such as MnO2,24 Fe2O3,25 UO2,26 Co3O4
20 and Cr2O3.27,28 There are several studies about γ-radiation induced synthesis of Cu2O particles. He et al.29 investigated the size-controlled preparation of Cu2O octahedron nanocrystals by γ-irradiation and found that the average edge of the octahedron Cu2O nanocrystals varied from 45 to 95 nm as a function of the γ-radiation dose rate. Furthermore, Liu et al.30 reported a shape controlled synthesis of Cu2O particles, such as eight-pod cubic, six-armed star-like, octahedral and spindle-like structure, by γ-irradiation with cetyltrimethylammonium bromide (CTAB) as a nucleation and growth controller.
It is well known that the pH value of the precursor solution can have a significant effect on the structural properties of the particles formed in aqueous systems.31–36 Also, solution pH influences the yield of the obtained Cu precipitate.37
The aim of the current study is to investigate the influence of pH of the Cu precursor solution on the composition, conversion and morphology of the Cu–Cu2O precipitate obtained by γ-radiation induced synthesis, as well as to work out the optimal conditions for synthesis of crystalline Cu2O without Cu admixtures.
Experimental
Radiation induced synthesis method
Radiation-induced formation of particles in solution is in general based on reactions between solvent radiolysis products and solute precursors. To produce metal or metal oxide particles, metal ions are usually used as solute precursors. In this work, water is used as solvent for the reaction system. Upon absorption of ionizing radiation water is decomposed into oxidizing and reducing species:24 |  | (1) |
The chemical change induced by γ-irradiation can be quantified via the radiation chemical yield or G-value which is the number of moles of a given species produced or consumed per absorbed Joule of radiation energy [mol J−1].24 Among the water radiolysis products, e−aq and OH˙ are produced with the highest yield at pH 7 in γ-irradiated solutions.24 The G-values of e−aq and OH˙ are both 0.28 μmol J−1 at times >10−6 s (ref. 24) after the initial absorption of the radiation energy. The G-values are pH independent in pH range from 3 to 13.38 The hydrated electron, e−aq, is a very strong reductant, E0(H2O/e−aq) = −2.87 V vs. SHE, capable of reducing most metal ions to lower valence states.39 It acts as the most effective reducing agent in the system, the rate constant of the reaction between e−aq and Cu2+ ions is 8.2 × 109 dm3 mol−1 S−1.40 OH˙ is a very strong oxidant capable of oxidizing metal ions to a higher oxidation states.24 For γ-radiation induced synthesis, the redox conditions of solution can be tuned to either oxidizing or reducing condition by adding radical scavengers prior to irradiation. The scavengers can react with undesired radicals and sometimes also convert them into other radicals with the desired properties. In the present work, the reducing route was selected to produce Cu2O particles in Cu2+ solution. This was achieved by using isopropanol which can react with OH˙ and produces the reducing radical (CH3)2CO˙H, E0((CH3)2CO/(CH3)2CO˙H) = −1.8 V vs. SHE:41
| (CH3)2CHOH + OH˙ → (CH3)2CO˙H + H2O. | (2) |
Furthermore, via the reaction (2) the isopropanol scavenges the oxidizing radical OH˙ and produce same amount of reducing radical (CH3)2CO˙H. Hence, the total G-value for reducing radicals in this system will be doubled (G(red) = G(e−aq) + G(OH˙)) and equal to 5.6 × 10−7 mol J−1.
Synthesis condition
In a typical preparation, the aqueous solution (Millipore Milli-Q) contained 0.01 M CuSO4·5H2O (Merck, 99.8%), 2.0 M isopropanol (Sigma-Aldrich, 99.9%) and 0.50 M CH3COOH/CH3COO− buffer. 0.008 M sodium dodecyl sulfate (SDS, Fluka, 99.0%) was used as surfactant in every experiments. The CH3COOH/CH3COO− ratio was varied to adjust pH between 3.75 and 4.40. The solutions were purged with high purity nitrogen (Standmollen, 99.999%) for 30 min to remove oxygen from the solution prior to irradiation. Thereafter, the solutions were irradiated using Cs-137 γ source at a dose rate of 0.124 Gy s−1 for 20 hours. The total absorbed dose was 8.9 kGy for each sample. After irradiation, the obtained red precipitate was washed with deionized water several times and filtered.
Characterization
Optical absorption spectra were acquired using a JASCO V-730 spectrophotometer to measure the Cu(II)-concentration in the copper sulfate solutions before and after irradiation. The intensities of the absorption peaks at a wavelength of 777 nm typical for Cu2+aq were used.42 Powder X-ray diffraction (XRD) patterns of synthesized particles were recorded by a PANalytical X'Pert PRO diffraction system using Cu Kα radiation (λ = 1.54 Å) in Bragg–Brentano geometry. XRD scans were recorded in 2θ range from 5° to 95° with a step of 0.01°. Scanning electron microscopy (SEM) and energy dispersive spectroscopy (EDS) were performed using a VP-SEM S3700N setup.
Results and discussion
The powder X-ray diffraction patterns of the precipitated particles produced at the different pH-values of the copper sulfate solutions are shown in Fig. 1. It should be noted that the solution pH before and after irradiation was found to be similar within the uncertainty of 0.05 pH-units. The pH values of the solutions are denoted in the figure. As seen in the figure, for the sample at pH 3.75, only the diffraction peaks attributed to metallic Cu were detected.43–45 While for the sample synthesized at pH 3.85, the characteristic peaks of Cu2O are observed13 in addition to those which belong to Cu. The two different copper species were also verified by SEM-EDS mapping, see Fig. S1.† With further increase of solution pH, the intensities of the diffraction peaks corresponding to Cu gradually decrease while those corresponding to Cu2O increase. Finally, at pH 4.4 only XRD peaks which belong to Cu2O are detected. To be able to quantify and compare the results from XRD measurements, the ratio ICu2O/(ICu + ICu2O) was introduced where ICu2O and ICu represented the sum of integral intensities of XRD peaks for Cu2O ((110), (111), (200) and (220) planes) and for Cu ((111) and (200) planes), respectively. Note, that the suggested ratio represented the relative content of Cu2O in the Cu–Cu2O crystalline phase precipitate. The XRD results were summarized in Table S1† and Fig. 3(a). Thus, by varying the copper sulfate solution pH without changing other synthesis parameters, one can tune the composition of the precipitate obtained by γ-radiation induced synthesis.
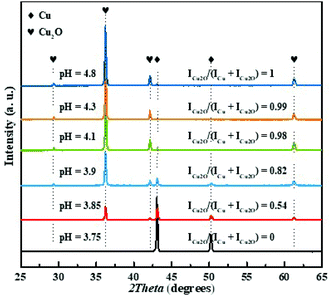 |
| Fig. 1 XRD patterns of the Cu–Cu2O powders synthesized at different pH values, rhombus and hearts denote XRD peaks for Cu and Cu2O, respectively. | |
The effect of pH can be understood from the E-pH diagram46 of the Cu–H2O system with 0.01 M Cu2+ shown in Fig. 2. As seen in the diagram at solution pH < 3.5, only metallic copper can be formed when lowering the potential, Cu2+ → Cu0. At 3.5 < pH < 5 there is a potential interval where Cu2O(s) is stable. However, upon further reduction of the potential Cu0 is formed.
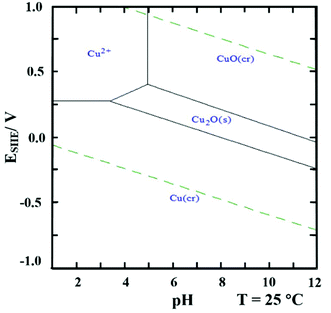 |
| Fig. 2 E-pH diagram showing predominant phases in the Cu–H2O system (0.01 M Cu2+ aqueous solution). | |
To elucidate the mechanism of Cu2O formation, we plotted the ratios of the integral intensities of the XRD peaks ICu2O/(ICu + ICu2O) as a function of solution pH, see Fig. 3(a). Also the conversion of Cu2+ (ΔCu2+), i.e., the change in Cu(II)-concentration upon irradiation, is plotted as a function of solution pH as well, see Fig. 3(b). As seen in Fig. 3(a) the ratio of ICu2O/(ICu + ICu2O) changes gradually from 0 to 1, indicating co-existence of pure crystalline Cu and Cu2O phases in the solution in the pH range used in the experiments. In the pH range from 3.75 to 4.0, the Cu–Cu2O precipitate can be obtained. It is also clear that the Cu2+ conversion increases gradually with solution pH and its value doubles when solely Cu2O forms.
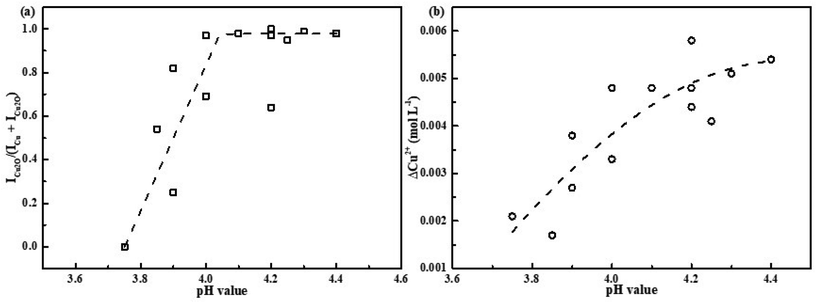 |
| Fig. 3 The ratios of relative XRD intensities ICu2O/(ICu + ICu2O), ICu2O/(ICu + ICu2O) was the ratios of integral intensities of XRD peaks, which ICu2O and ICu represented the sum of integral intensities of peaks for Cu2O ((110), (111), (200) and (220)) and for Cu ((111) and (200)), respectively. (a) and Cu2+ conversion yield ΔCu2+ (b) as a function of solution pH, dash lines are guide for eyes. | |
Cu2+ will be reduced to form Cu2O through the following reactions (3)–(5).42,47 Metallic Cu particles could either be produce by two consecutive one-electron transfer steps from Cu2+via Cu+ to Cu0, reactions (3) and (6),40 or through disproportionation of Cu+, reaction (7):
| Cu2+ + e−aq or (CH3)2CO˙H → Cu+ | (3) |
|  | (6) |
The change in ICu2O/(ICu + ICu2O) with pH implies that there is a pH-dependent competition between the reaction yielding Cu2O (4) and the reactions yielding Cu (6) and (7), where reaction (4) is favored at higher pH. The apparent pH-effect on the Cu2+ conversion at the given absorbed dose directly follows from this since two electrons are required to convert Cu2+ to Cu while only one electron per Cu2+ is required to produce Cu2O according to proposed scheme. This is confirmed by the results shown in Fig. 3.
The influence of scavenger (isopropanol) concentration on Cu2O formation was also investigated and the results are shown in Fig. 4. As the results indicate, the ratio of ICu2O/(ICu + ICu2O) increases with increasing isopropanol concentration in the range 0.5 M to 1.0 M. This could partly be attributed to hydroxyl radicals reacting with the buffer in competition with the desired reaction with isopropanol, reaction (2). However, based on the relative rate constants of these reactions (ki = 2.3 × 10−9 mol−1 dm3 s−1 for hydroxyl radicals react with isopropanol, ka = 9.2 × 10−6 mol−1 dm3 s−1 for hydroxyl radicals react with acetic acid), this effect is expected to be quite small.48,49 Given the magnitude of the isopropanol concentration effect, it is more likely that it can be attributed to solvent effects on the relative rates of the competing reactions. Since the isopropanol concentration effect is only observed below 1.00 M, 2.00 M of isopropanol was selected for the current study.
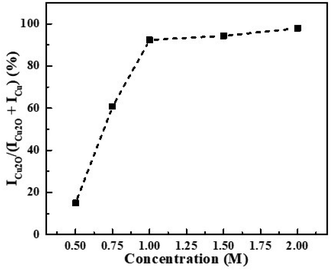 |
| Fig. 4 The isopropanol concentration as a function of the ratios of ICu2O/(ICu + ICu2O) prepared at the solution pH 4.40. ICu2O/(ICu + ICu2O) was the ratios of integral intensities of XRD peaks, which ICu2O and ICu represented the sum of integral intensities of peaks for Cu2O ((110), (111), (200) and (220)) and for Cu ((111) and (200)), respectively. | |
It was also found that solution pH influences the morphologies of produced Cu2O particles. SEM images of the precipitate were collected and are shown in Fig. 5(a)–(e). The Cu2O particle size distributions obtained from the SEM images are shown in Fig. 5(f). Fig. 5(a) shows the SEM image of the metallic Cu particles obtained at pH 3.75. It was found that the Cu particles were quasi-spherical agglomerates with a 2.9 μm average size. When solution pH is increased to 3.85, two different types of particles are formed: agglomerated Cu particles and the octahedron-shaped particles which correspond to the Cu2O phase.7,8,14 The surfaces of the octahedrons have (111) orientation. It has been reported previously that SO42− ions50 and SDS51,52 can increase the stability of {111} planes of Cu2O which could result in the formation octahedron-shaped Cu2O particles. As shown in Fig. 5(a)–(e), with increasing solution pH, the fraction of quasi-spherical Cu particles is gradually decreased, whereas the Cu2O octahedrons becomes the main phase of the produced particles. Note that the average size of the Cu2O particles is decreased from 13.5 μm to around 7 μm (Fig. 5(f)). This could be due to the fact that at high solution pH the nucleation of Cu2O is favorable and therefore, likely to more nucleation centers of Cu2O are produced. This could lead to smaller particle size and likely to more narrow size distribution.29,30,53 Solely Cu2O particles with octahedron shape are synthesized at pH 4.40 and is shown in Fig. 5(e).
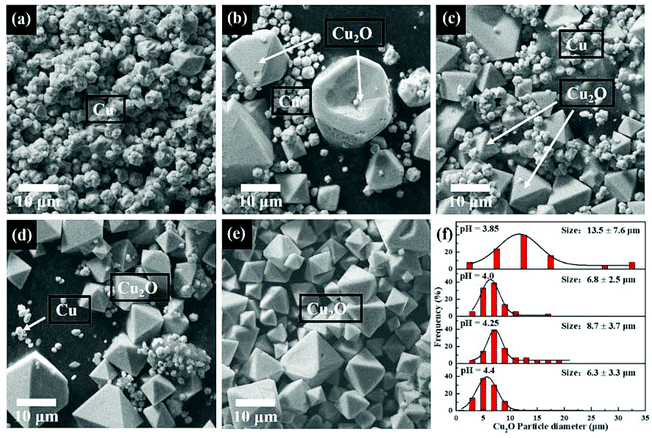 |
| Fig. 5 SEM image of the synthesized particles by γ-radiation induced method at pH value of 3.75 (a), 3.85 (b), 4.0 (c), 4.25 (d), 4.4 (e) and the Cu2O particles size distribution at different pH (f). | |
Conclusions
Cuprous oxide particles were synthesized by the reduction of dissolved Cu2+ ions from CuSO4 aqueous solution using a γ-radiation induced synthesis route. It was found that by changing the solution pH the composition and morphology of the obtained precipitate can be tuned. Thus, at solution pH < 3.75 metallic copper quasi-spherical agglomerates are formed while at pH > 4.40 just Cu2O octahedron-shaped particles are precipitated. In the pH range from 3.75 to 4.40, a mixture of both metallic Cu particles and Cu2O particles are produced and the relative amount of crystalline Cu2O in the precipitate increases with increasing pH. As a direct consequence, the overall conversion of Cu2+ is increased with increasing solution pH. The observed pH effect can be attributed to pH-dependent competition between the reactions yielding Cu and the reactions yielding Cu2O. Thus, by controlling pH of the CuSO4 solution during γ-radiation induced synthesis one can tune the composition and morphology of the obtained precipitate.
Conflicts of interest
There are no conflicts to declare.
Acknowledgements
The China Scholarship Council (CSC) (Grant Number: 201700260193) is gratefully acknowledged for financial support. Pavel Yushmanov is acknowledged for his help.
References
- B. Hvolbæk, T. V. W. Janssens, B. S. Clausen, H. Falsig, C. H. Christensen and J. K. Nørskov, Nano Today, 2007, 2, 14–18 CrossRef.
- S. Laurent, D. Forge, M. Port, A. Roch, C. Robic, L. V. Elst and R. N. Muller, Chem. Rev., 2008, 108, 2064–2110 CrossRef CAS PubMed.
- D. A. Dinh, K. S. Hui, K. N. Hui, Y. R. Cho, W. Zhou, X. Hong and H. H. Chun, Appl. Surf. Sci., 2014, 298, 62–67 CrossRef CAS.
- S. Zinatloo-Ajabshir, M. Salavati-Niasari and M. Hamadanian, RSC Adv., 2015, 5, 33792–33800 RSC.
- J.-Y. Ho and M. H. Huang, J. Phys. Chem. C, 2009, 113, 14159–14164 CrossRef CAS.
- K. Pan, H. Ming, H. Yu, Y. Liu, Z. Kang, H. Zhang and S. T. Lee, Cryst. Res. Technol., 2011, 46, 1167–1174 CrossRef CAS.
- Y. S. Panova, A. S. Kashin, M. G. Vorobev, E. S. Degtyareva and V. P. Ananikov, ACS Catal., 2016, 6, 3637–3643 CrossRef CAS.
- X. Zhang, Y. Xie, F. Xu, X. Liu and D. Xu, Inorg. Chem. Commun., 2003, 6, 1390–1392 CrossRef CAS.
- H. B. N. Choon and Y. F. Wai, J. Phys. Chem. B, 2006, 110, 20801–20807 CrossRef PubMed.
- R. Wick and S. D. Tilley, J. Phys. Chem. C, 2015, 119, 26243–26257 CrossRef CAS.
- Y. H. Won and L. A. Stanciu, Sensors, 2012, 12, 13019–13033 CrossRef CAS PubMed.
- Y. Moglie, E. Mascaró, V. Gutierrez, F. Alonso and G. Radivoy, J. Org. Chem., 2016, 81, 1813–1818 CrossRef CAS PubMed.
- S. Kumar, C. M. A. Parlett, M. A. Isaacs, D. V. Jowett, R. E. Douthwaite, M. C. R. Cockett and A. F. Lee, Appl. Catal., B, 2016, 189, 226–232 CrossRef CAS.
- H. Xu, W. Wang and W. Zhu, J. Phys. Chem. B, 2006, 110, 13829–13834 CrossRef CAS PubMed.
- H. Yu, J. Yu, S. Liu and S. Mann, Chem. Mater., 2007, 19, 4327–4334 CrossRef CAS.
- M. Wang, L. Xu, M. Zhai, J. Peng, J. Li and G. Wei, Carbohydr. Polym., 2008, 74, 498–503 CrossRef CAS.
- D. Wu, X. Ge, Y. Huang, Z. Zhang and Q. Ye, Mater. Lett., 2003, 57, 3549–3553 CrossRef CAS.
- P. A. Yakabuskie, J. M. Joseph, P. Keech, G. A. Botton, D. Guzonas and J. C. Wren, Phys. Chem. Chem. Phys., 2011, 13, 7198 RSC.
- D. C. Clifford, C. E. Castano and J. V. Rojas, Radiat. Phys. Chem., 2017, 132, 52–64 CrossRef CAS.
- I. L. Soroka, N. V. Tarakina, A. Hermansson, L. Bigum, R. Widerberg, M. S. Andersson, R. Mathieu, A. R. Paulraj and Y. Kiros, Dalton Trans., 2017, 46, 9995–10002 RSC.
- M. E. Meyre, M. Tréguer-Delapierre and C. Faure, Langmuir, 2008, 24, 4421–4425 CrossRef CAS PubMed.
- J. Vinod Kumar, N. Lingaiah, K. S. Rama Rao, S. P. Ramnani, S. Sabharwal and P. S. Sai Prasad, Catal. Commun., 2009, 10, 1149–1152 CrossRef CAS.
- H. Wang, X. Sun, Y. Ye and S. Qiu, J. Power Sources, 2006, 161, 839–842 CrossRef CAS.
-
J. W. T. Spinks and R. J. Woods, An introduction to Radiation Chemistry, Wiley, New York-London-Sydney, 1990 Search PubMed.
- S. Bzdon, J. Góralski, W. Maniukiewicz, J. Perkowski, J. Rogowski and M. Szadkowska-Nicze, Radiat. Phys. Chem., 2012, 81, 322–330 CrossRef CAS.
- O. Roth, H. Hasselberg and M. Jonsson, J. Nucl. Mater., 2009, 383, 231–236 CrossRef CAS.
- L. M. Alrehaily, J. M. Joseph, A. Y. Musa, D. A. Guzonas and J. C. Wren, Phys. Chem. Chem. Phys., 2013, 15, 98–107 RSC.
- L. M. Alrehaily, J. M. Joseph and J. C. Wren, J. Phys. Chem. C, 2015, 119, 16321–16330 CrossRef CAS.
- P. He, X. Shen and H. Gao, J. Colloid Interface Sci., 2005, 284, 510–515 CrossRef CAS PubMed.
- H. Liu, W. Miao, S. Yang, Z. Zhang and J. Chen, Cryst. Growth Des., 2009, 9, 1733–1740 CrossRef CAS.
- S. Das and V. C. Srivastava, Mater. Lett., 2015, 150, 130–134 CrossRef CAS.
- K. Okitsu, K. Sharyo and R. Nishimura, Langmuir, 2009, 25, 7786–7790 CrossRef CAS PubMed.
- A. P. A. Faiyas, E. M. Vinod, J. Joseph, R. Ganesan and R. K. Pandey, J. Magn. Magn. Mater., 2010, 322, 400–404 CrossRef CAS.
- X. Li, W. X. Chen, J. Zhao, W. Xing and Z. De Xu, Carbon, 2005, 43, 2168–2174 CrossRef CAS.
- A. V. Nikam, A. Arulkashmir, K. Krishnamoorthy, A. A. Kulkarni and B. L. V. Prasad, Cryst. Growth Des., 2014, 14, 4329–4334 CrossRef CAS.
- T. M. D. Dang, T. T. T. Le, E. Fribourg-Blanc and M. C. Dang, Adv. Nat. Sci.: Nanosci. Nanotechnol., 2011, 2, 015009 Search PubMed.
- F. S. Ohuchi, T. J. Lin, J. A. Antonelli and D. J. Yang, Mater. Res. Bull., 1994, 6090, 10–16 Search PubMed.
-
A. Mozumder, Fundamentals of Radiation Chemistry, Academic Press, USA, 1999 Search PubMed.
- H. A. Schwarz, J. Chem. Educ., 1981, 58, 101 CrossRef CAS.
- G. R. Dey, Radiat. Phys. Chem., 2005, 74, 172–184 CrossRef CAS.
- R. E. Huie, C. L. Clifton and P. Neta, Int. J. Radiat. Appl. Instrum. Part, 1991, 38, 477–481 CAS.
- I. L. Soroka, A. Shchukarev, M. Jonsson, N. V. Tarakina and P. A. Korzhavyi, Dalton Trans., 2013, 42, 9585 RSC.
- A. Karelovic and P. Ruiz, Catal. Sci. Technol., 2015, 5, 869–881 RSC.
- D. Vargas-Hernández, J. M. Rubio-Caballero, J. Santamaría-González, R. Moreno-Tost, J. M. Mérida-Robles, M. A. Pérez-Cruz, A. Jiménez-López, R. Hernández-Huesca and P. Maireles-Torres, J. Mol. Catal. A: Chem., 2014, 383–384, 106–113 CrossRef.
- I. V. Korolkov, O. Güven, A. A. Mashentseva, A. B. Atıcı, Y. G. Gorin, M. V. Zdorovets and A. A. Taltenov, Radiat. Phys. Chem., 2017, 130, 480–487 CrossRef CAS.
- J. M. Steigerwald, S. P. Murarka, R. J. Gutmann and D. J. Duquette, Mater. Chem. Phys., 1995, 41, 217–228 CrossRef CAS.
- Q. Chen, X. Shen and H. Gao, J. Colloid Interface Sci., 2007, 312, 272–278 CrossRef CAS PubMed.
- A. J. Elliot and A. S. Simsons, Radiat. Phys. Chem., 1984, 24, 229–231 CrossRef CAS.
- J. K. Thomas, Trans. Faraday Soc., 1965, 61, 702–707 RSC.
- M. J. Siegfried and K. S. Choi, J. Am. Chem. Soc., 2006, 128, 10356–10357 CrossRef CAS PubMed.
- M. J. Siegfried and K. S. Choi, Angew. Chem.,Int. Ed., 2005, 44, 3218–3223 CrossRef CAS PubMed.
- M. J. Siegfried and K. S. Choi, Adv. Mater., 2004, 16, 1743–1746 CrossRef CAS.
- J. Belloni, Catal. Today, 2006, 113, 141–156 CrossRef CAS.
Footnote |
† Electronic supplementary information (ESI) available. See DOI: 10.1039/c8dt02916d |
|
This journal is © The Royal Society of Chemistry 2018 |
Click here to see how this site uses Cookies. View our privacy policy here.