DOI:
10.1039/D2QI01152B
(Research Article)
Inorg. Chem. Front., 2022,
9, 4952-4961
Size exclusion propyne/propylene separation in an ultramicroporous yet hydrophobic metal–organic framework†
Received
30th May 2022
, Accepted 25th July 2022
First published on 27th July 2022
Abstract
Propyne/propylene separation is important in the petrochemical industry but challenging due to their similar physical properties and close molecular sizes. Herein, we present two isoreticular ultramicroporous Zn(II)-MOFs, Zn2(ATZ)2(TPDC) (BUT-305, H2TPDC = [1,1′:3′,1′′-terphenyl]-4,4′′-dicarboxylic acid, HATZ = 3-amino-1,2,4-triazole) and Zn2(ATZ)2(MeTPDC) (BUT-306, H2MeTPDC = 5′-methyl-[1,1′:3′,1′′-terphenyl]-4,4′′-dicarboxylic acid). The pore aperture of BUT-306 (∼1.6 Å) is smaller than that of BUT-305 due to the presence of extra gate-like methyl groups in the 1D channels of the former. With a narrow and hydrophobic pore aperture, BUT-306 exhibits high hydrophobicity and hydrolytic stability and adsorbs C3H4 but excludes C3H6 in a wide temperature range. The C3H6 and C3H4 adsorption capacities of BUT-306 at 298 K and ∼1 bar were 2.4 and 29.6 cm3 g−1, respectively. Dynamic column breakthrough experiments confirmed the high capability of BUT-306 to remove C3H4 from the equimolar binary gas mixture of C3H4 and C3H6. The C3H4/C3H6 separation performance of BUT-306 was largely retained even when the binary C3H4/C3H6 gas for breakthrough experiments was pre-saturated with water vapor. In addition, the single-crystal structure of C3H4-loaded BUT-306 was determined, which revealed that the adsorbed C3H4 molecules were located in the center of channel cavities and interacted with the MOF by multiple weak Cδ−⋯Cδ+ dipole–dipole interactions and C–H⋯п interactions. This work demonstrates the high potential of an ultramicroporous, hydrophobic, and hydrolytically stable MOF in the removal of C3H4 from the C3H4/C3H6 gas mixture by size exclusion adsorption. The structure and gas adsorption studies shed light on the design and synthesis of new adsorbents for the separation of light hydrocarbons.
Introduction
Propylene (C3H6), as a raw material of many chemical products, plays a key role in the petrochemical industry.1 C3H6 is mostly produced from the cracking of large hydrocarbon molecules or crude oil in the petrochemical industry commonly accompanied by propyne (C3H4) as an undesirable byproduct, which can poison the catalysts for propylene polymerization.2 Efficient removal of C3H4 from the C3H4/C3H6 mixture is thus of high importance. Traditional C3H4/C3H6 separation methods, such as cryogenic distillation or catalytic partial hydrogenation, suffer from high cost and high energy penalties.3,4 The physical adsorption method based on porous materials is attractive for gas separation owing to its environmentally friendly processes and energy efficiency.5–11 Highly efficient C3H4/C3H6 separation using traditional porous materials (such as zeolites and activated carbons) remains challenging because of highly similar physical properties, molecular sizes (C3H6: 4.65 × 4.16 × 6.44 Å3 and C3H4 4.01 × 4.16 × 6.51 Å3) and kinetic diameters (C3H6: 4.68 Å and C3H4: 4.76 Å) of the two hydrocarbons.12
As a class of newly emerged porous materials, metal–organic frameworks (MOFs)13–20 have been widely used for gas separation owing to their structural diversity,21–26 adjustable pore size27–30 and designable adsorption sites.31–37 Most reported MOFs used for C3H4/C3H6 separation are based on their open metal sites or functional groups which can strongly interact with C3H4. For example, the MOF-74 series (Mg, Co, Ni, and Fe-MOF-74)38 with open metal sites and the SIFSIX series (SIFSIX-3-Ni,39 SIFSIX-1-Cu,40 and SIFSIX-2-Cu-i19) with SiF62− anion pillars can afford high adsorption capacity and strong interaction for C3H4.34 However, these MOFs allow diffusion of both C3H4 and C3H6 into their pores,2,34,41–46 resulting in a compromised separation efficiency. In addition, the regeneration processes are relatively energy-intense due to the high affinity of these MOFs to C3H4. Therefore, the development of adsorbents with high performance in selective adsorption of C3H4 over C3H6 primarily relying on the molecular sieving effect would be complementary. However, among the reported MOFs, only UTSA-200 showed molecular sieving C3H4/C3H6 separation performance with a high adsorption selectivity of over 20
000.32 In addition, stability and recyclability are also important for porous materials in gas separation applications,47 especially when the gas mixtures to be purified contain reactive non-hydrocarbon impurities, e.g. water, which is ubiquitous.
Herein, we report two ultramicroporous MOFs, Zn2(ATZ)2(TPDC) (BUT-305, H2TPDC = [1,1′:3′,1′′-terphenyl]-4,4′′-dicarboxylic acid, HATZ = 3-amino-1,2,4-triazole) and Zn2(ATZ)2(MeTPDC) (BUT-306, H2MeTPDC = 5′-methyl-[1,1′:3′,1′′-terphenyl]-4,4′′-dicarboxylic acid), which are 3D framework structures consisting of 2D [Zn(ATZ)] layers pillared by TPDC2− and MeTPDC2− ligands (Scheme 1), respectively. The difference between the two isoreticular MOFs is that there are extra gate-like methyl groups in the 1D channels of BUT-306. Owing to its narrow and hydrophobic pore aperture, BUT-306 exhibits high hydrolytic stability, hydrophobicity, and potential in the removal of C3H4 from the C3H4/C3H6 mixture. In addition, the location of C3H4 molecules inside the pore of BUT-306 and the host–guest interactions have been elucidated by single-crystal structural analyses.
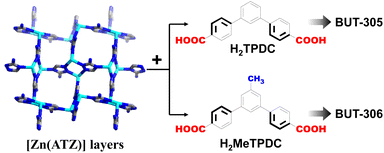 |
| Scheme 1 The construction and building blocks of BUT-305 and -306. | |
Results and discussion
Single-crystal structures and chemical stability
As-synthesized crystals of BUT-305 and -306 were obtained through the solvothermal reactions of zinc salt and organic ligands (HATZ and H2MeTPDC or HATZ and H2MeTPDC) in the mixture of DMF and a small amount of aqueous HBF4 solution. The as-synthesized crystals were guest-exchanged in MeOH solvent for 3 days at 120 °C followed by heating at 120 °C for 18 hours to remove guest molecules, resulting in the formation of BUT-305 and -306 samples. The SCXRD measurement of BUT-306 revealed that it crystallizes in the tetragonal I4/m space group (Table S1†) and it is isoreticular to Zn2(ATZ)2(iPA) (H2iPA = isophthalic acid) as reported by Chen and co-workers.48 The 2D [Zn(ATZ)] layers are linked by the MeTPDC2− pillars to form a 3D pillar-layered framework with 1D channels along the crystallographic c-axis (Fig. 1a). The cross-sectional diameters of the channel are estimated to be 1.6 to 5.0 Å by Materials Studio.49 It is worthy of note that the channel is highly tortuous with three types of cavities (A, B, and C), and it is divided into parts by sets of gate-like methyl groups, each set of which are from 4 neighboring MeTPDC2− ligands (Fig. 1b). The aperture defined by the 4 gate-like methyl groups is only about 1.6 Å in diameter. Regardless of the small aperture size, BUT-306 is still potentially porous as the total potential solvent area volume accounts for 23.6% of the unit cell volume of BUT-306 and the pore volume is estimated to be 0.17 cm3 g−1 by Platon.50
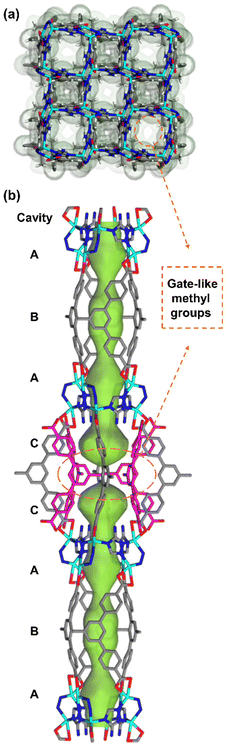 |
| Fig. 1 The crystal structure of BUT-306: (a) the 3D framework view along the c axis and (b) side-view of the channel. Color codes: Zn, turquoise; O, red; N, blue; C, grey; H, white; and Connolly surface (probe radius 1.7 Å), green. The ligands coordinated with the metal ions on the walls of neighboring channels are shown in pink for clarity. | |
Due to the thin-plate-like shape of BUT-305 crystals, attempts to determine their structure by SCXRD failed. The PXRD pattern of BUT-305 resembled that of BUT-306, suggesting that BUT-305 is likely isoreticular to BUT-306 (Fig. 2a). Indexing and Pawley refinement results for the PXRD pattern of BUT-305 showed that it also crystallizes in the same space group (I4/m) with a slightly larger unit cell relative to that of BUT-306 (Fig. 2b and Table S2†). Due to the absence of methyl groups on the ligands of BUT-305, it is expected that the channels of BUT-305 are larger than those of BUT-306. The difference in the channels of the two MOFs results in their distinctive gas separation performance, which will be discussed in detail below. The PXRD patterns of BUT-305 and -306 samples well matched the simulated one from the single-crystal structure of BUT-306 (Fig. 2a), which confirmed that the prepared BUT-305 and -306 samples were pure phases. In addition, the PXRD measurements showed that the highly crystalline structures of BUT-305 and -306 samples remained essentially unchanged after they were treated with methanol at 120 °C for 3 days (Fig. S1†) or treated with water at room temperature for 24 hours (Fig. 2a), demonstrating their high hydrolytic stability.
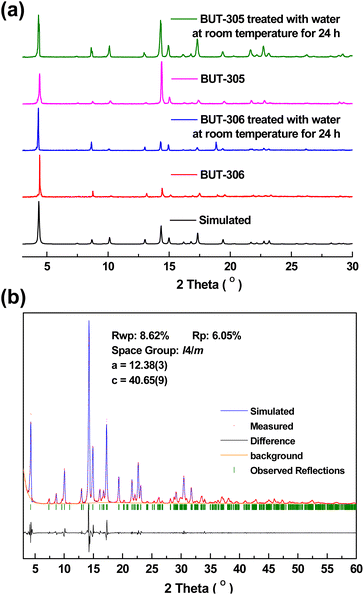 |
| Fig. 2 (a) The PXRD patterns of BUT-305 and BUT-306. (b) The results of Pawley refinement for the PXRD data of BUT-305. For comparison, the space group and cell parameters of BUT-306 are as follows: I4/m, a = 12.3420(2) Å, and c = 40.6826(9) Å. | |
Adsorption studies
To verify the accessibility of their pores, N2 adsorption isotherms of BUT-305 and -306 were recorded at 77 K (Fig. S2†). Although the calculated pore volumes of the two MOFs are over 0.17 cm3 g−1, type I N2 adsorption isotherms were not obtained. The N2 uptake at P/P0 ≈ 1 was only 1.2 cm3 g−1 for BUT-306, suggesting that N2 molecules could not diffuse into its channels at 77 K. The N2 uptake of BUT-305 gradually increased as the pressure is increased with an N2 uptake of 77.1 cm3 g−1 at P/P0 ≈ 1, which might result from the comparable size of the N2 molecule and the pore of BUT-305. The CO2 adsorption isotherms of BUT-305 and -306 at 195 K were then recorded. As shown in Fig. S3,† the two MOFs showed an abrupt increase in CO2 uptake (to ∼70 cm3 g−1) at low pressures (P/P0 < 0.1). At higher pressures, the CO2 uptake of BUT-306 only slightly increased, while that of BUT-305 increased to 193 cm3 g−1 at P/P0 ≈ 1 by two additional stepwise increases, indicating the presence of some difference in the pore size, shape and/or flexibility of the two MOFs. According to the adsorption data at low pressures, the BET surface areas of BUT-305 and -306 were estimated to be 351 and 305 m2 g−1, respectively. Water adsorption isotherms were also recorded for the MOFs at 298 K. Type III water adsorption isotherms were obtained for both MOFs (Fig. 3a and S4†), and the water uptakes at P/P0 ≈ 1 were 160 and 80 cm3 g−1 for BUT-305 and BUT-306, respectively. These results indicated that the two MOFs were capable of accommodating small molecules and their pore surface was relatively hydrophobic.
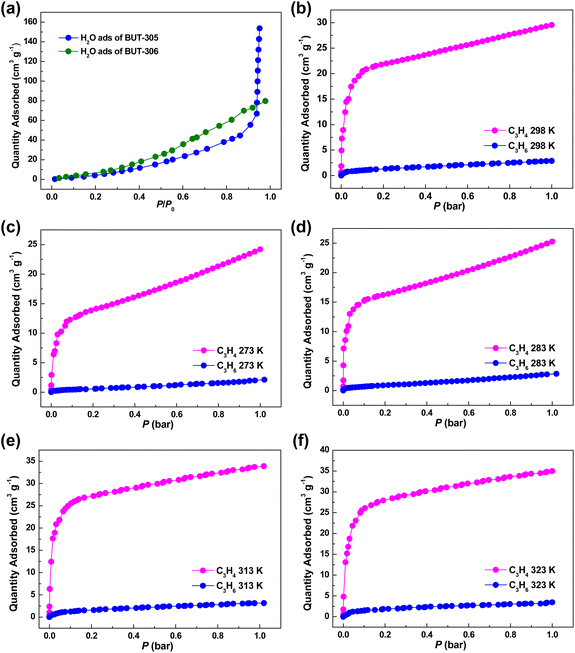 |
| Fig. 3 Adsorption and separation performance of BUT-306. (a) The H2O adsorption isotherms of BUT-305 and -306 at 298 K. Single-component C3H4 and C3H6 adsorption isotherms of BUT-306 at (b) 298 K, (c) 273 K, (d) 283 K (e) 313 K and (f) 323 K, respectively. | |
Encouraged by the high stability, accessible porosity and small pore apertures of the two MOFs, we explored their performances in light hydrocarbon separations. Adsorption isotherms for C2H2, C2H4, C2H6, C3H4, C3H6, and C3H8 were recorded at 298 K, as shown in Fig. S5.† The adsorption capacities of BUT-305 for C2H2, C2H4 and C2H6 at ∼1 bar were similar (42.3–44.6 cm3 g−1), and its adsorption capacities for C3H4 (44.4 cm3 g−1) and C3H6 (43.9 cm3 g−1) at ∼1 bar were also close, but that for C3H8 (33.8 cm3 g−1) was lower. These results suggested that all these hydrocarbons could enter the pore of BUT-305, and the differences in adsorption capacities of BUT-305 for these hydrocarbons were not profound. In contrast, strong size exclusion effects were observed in the hydrocarbon adsorption isotherms of BUT-306. For C2 hydrocarbons, the uptake of BUT-306 for C2H2 (36.6 cm3 g−1) or C2H4 (30.3 cm3 g−1) was obviously higher than that for C2H6 (11.0 cm3 g−1) at ∼1 bar. Moreover, the uptakes of BUT-306 for C3H6 (2.4 cm3 g−1) and C3H8 (0.33 cm3 g−1) at ∼1 bar were nearly negligible (Fig. S5d†), but a type I-like C3H4 adsorption isotherm was obtained for BUT-306 with a relatively high uptake of 29.6 cm3 g−1 at ∼1 bar (Fig. 3b and S6†), indicating its potential in the removal of C3H4 from the C3H4/C3H6 mixture. Although the C3H4 adsorption capacity of BUT-306 is lower than those of some reported MOFs under similar conditions, e.g., SIFSIX-2-Cu-i (84.4 cm3 g−1), UTSA-200 (80.2 cm3 g−1) and ELM-12 (62.1 cm3 g−1), BUT-306 hardly permits the entrance of C3H6 molecules into its pores. The C3H6 adsorption capacity of BUT-306 was only 2.4 cm3 g−1 at ∼1 bar and 298 K, significantly lower than those of the reported MOFs (Table S3†),32,51,52 including SIFSIX-2-Cu-i (58.9 cm3 g−1), UTSA-200 (26.9 cm3 g−1), and ELM-12 (32.0 cm3 g−1). The size exclusion effects of BUT-306 on the adsorption of C3H4 over C3H6 should be related to the small apertures defined by gate-like methyl groups in its 1D channels.
For a better comparison, the uptake ratios of BUT-306 for C3H4 over C3H6 at 0.99 bar or at 0.01/0.99 bars (0.01 bar for C3H4 and 0.99 for C3H6) and 298 K were calculated, being 10.75 and 3.67, respectively, which are much higher than those of the reported porous materials (1.05–3.07 at 0.99 bar; 0.03–2.49 at 0.01/0.99 bars) under similar conditions (Table S3†). Furthermore, ideal adsorbed solution theory (IAST) was used to predict the C3H4/C3H6 adsorption selectivity of BUT-306. Assuming the gas mixture with C3H4/C3H6 ratios (v/v) of 1
:
1, 1
:
9 and 1
:
99, the IAST C3H4/C3H6 adsorption selectivities of BUT-306 at ∼1 bar and 298 K were calculated to be 636, 717, and 989, respectively (Fig. S7†).
To explore the adsorption performances of BUT-306 for C3H4 and C3H6 in a broader temperature range, C3H4 and C3H6 adsorption isotherms were measured at 273, 283, 313 and 323 K, respectively. As shown in Fig. 3c–f, type I-like adsorption isotherms were obtained for C3H4 at all four temperatures, and the adsorption capacities at ∼1 bar were all over 25 cm3 g−1, while the C3H6 uptakes were all relatively low (<5 cm3 g−1). It is indicated that the size exclusion adsorption behavior of BUT-306 is not highly sensitive to the temperature. The adsorption capacity of BUT-306 for C3H4 or C3H6 gradually increased as the temperature increased from 273 to 323 K. For example, the C3H4 uptake at ∼1 bar increased from 24.2 cm3 g−1 at 273 K to 35.0 cm3 g−1 at 323 K. The adsorption data at these temperatures were further verified by repeated adsorption isotherm measurements (Fig. S8†). It is not common because physisorption is essentially exothermic, and the adsorption capacities at a certain equilibrium pressure should decrease when increasing the adsorption temperature for typical physical adsorption adsorbents. The abnormal adsorption behavior suggests the existence of structural flexibility in BUT-306.53,54 To verify this, SCXRD measurements were carried out for the same one single crystal of BUT-306 at 273 K, 313 K and 298 K, respectively. As shown in Fig. S9 and Table S1,† the unit cell parameters were nearly unchanged and no obvious structural change was observed during varying the measurement temperatures, indicating that the structural flexibility was induced by the adsorption of hydrocarbons rather than the change of temperature. Otherwise, according to the static equilibrium crystal structure of BUT-306, the narrowest pore aperture is too small (diameter: 1.6 Å) to let the hydrocarbon molecules diffuse into the channels. The increase of measurement temperature should be beneficial for the diffusion of C3H4 molecules into BUT-306 as there is an energy barrier between the guest-free state and the guest-included state originating from the structural flexibility of BUT-306, which leads to the uncommon adsorption behavior of BUT-306 as mentioned above.
For a better understanding of the C3H4 adsorption process of BUT-306, the single-crystal structure of C3H4-loaded BUT-306 was determined. A single crystal of BUT-306 preheated at 50 °C under an N2 atmosphere for 6 hours was exposed to the atmosphere of C3H4 at ambient pressure and temperature for 30 minutes to load the hydrocarbon molecules, which was then mounted on a single-crystal X-ray diffractometer under a low-temperature N2 flow bath (200 K) for data collection. Structure determination and refinement results revealed that the framework of BUT-306 was essentially unchanged after C3H4 loading (Fig. S9 and Table S1†) and two crystallographically independent C3H4 molecules were found inside its channels. As the framework was retained, the narrowest channel apertures defined by the methyl groups of MeTPDC2− ligands kept unchanged after C3H4 loading, indicating that there was a transient structural variation to open the gate-like apertures when C3H4 molecules diffused into the channels. The transient gate-opening might have resulted from the swing or distortion of the MeTPDC2− ligands.
The two types of C3H4 molecules are located inside cavities A and B with a linear molecular shape along the 4-fold rotation axis, respectively (Fig. 4a). The C–C triple bond and single bond of the C3H4 molecule located at cavity A (C3H4-A) are 1.08(8) and 1.54(8) Å, respectively. The C3H4 molecule located at cavity B (C3H4-B) is normal to the reflection plane crossing its center C atom. Due to the symmetry-imposed disorder, the two C–C bonds of C3H4-B are same in length, being 1.24(8) Å, close to the average length of a C–C triple bond and a C–C single bond. Occupations of the two C3H4 molecules were set to be 20%, and no geometrical restraints were used in the structure refinements. The C3H4 molecules are confined inside the cavities by multiple weak host–guest interactions with the framework. Specifically, the distance between the terminal sp-hybridized C atom (with a negative charge) of C3H4-A and the 3-position C atoms (with a positive charge) of four neighboring ATZ− ligands on the channel walls is 3.50 Å (Fig. 4b), slightly larger than the van der Waals radii of two C atoms (1.7 × 2 Å), indicating the presence of weak Cδ−⋯Cδ+ dipole–dipole interactions. In addition, there are fourfold weak C–H⋯п interactions between C3H4-B and the aromatic H atoms of four neighboring MeTPDC2− ligands (CC3H4⋯H distances: 3.76, 3.95 Å; <C–H⋯C angles: 85.9, 86.1°) (Fig. 4c). Though every single interaction is weak, the overall interactions between C3H4 and MOF should be moderate because each C3H4 molecule is interacting with four neighboring channel walls simultaneously. Assuming that the two adsorption sites are fully occupied, the calculated C3H4 uptake of BUT-306 is 26.8 cm3 g−1, which is slightly lower than the experimentally observed uptake (29.6 cm3 g−1) at ∼1 bar and 298 K. It is noteworthy that C3H4 uptake of BUT-306 at ∼1 bar gradually increased from 24.2 to 35.0 cm3 g−1 as the temperature increased from 273 to 323 K. This fact suggests that the adsorbed C3H4 molecules could be also located inside the cavity C besides cavities A and B. When each of the cavities is occupied by one C3H4 molecule, the calculated C3H4 uptake of BUT-306 is 35.7 cm3 g−1, close to the uptake (35.0 cm3 g−1) observed at ∼1 bar and 323 K, further justifying the assignment of adsorption sites.
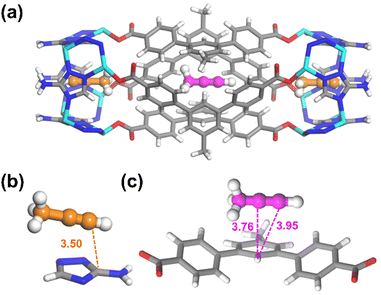 |
| Fig. 4 (a) The two types of C3H4 adsorption sites in the cavities of BUT-306. The interaction between the BUT-306 framework and the C3H4 molecules located in cavity A (b) and in cavity B (c). Color codes: Zn, turquoise; O, red; N, blue; C, grey; and H, white. For clarity, the C atoms of C3H4-A and C3H4-B are in gold and pink colors, respectively. | |
Dynamic column breakthrough studies
Dynamic column breakthrough experiments of a binary gas of C3H4/C3H6 (1
:
1, v/v) were carried out at ambient pressure and temperature to further evaluate the separation performance of BUT-306 for a real gas mixture. As the binary gas flowed through the column packed with BUT-306, purified C3H6 was detected in the outlet of the column, while C3H4 was trapped in the column until the retention time reached ∼10 min g−1 (Fig. 5a). After saturation of gas adsorption, the MOF was regenerated at 393 K under a He gas flow. Meanwhile, the gas eluted from the outlet was monitored. As shown in Fig. 5b, the peak area of desorbed C3H4 was significantly larger than that of desorbed C3H6, indicating the high adsorption selectivity of C3H4 over C3H6. The dynamic C3H4 productivity is estimated to be 0.87 mol kg−1 h−1 with a purity of 90.3% by analyzing the breakthrough and desorption curves. It is important to note that during the C3H4/C3H6 separation process, the fewer the C3H6 molecules the adsorbent adsorbs, the higher the C3H6 purification productivity and efficiency. The BUT-306 sample was regenerated and used for the C3H4/C3H6 breakthrough experiment for two more cycles, and its separation performance essentially unchanged (Fig. 5a), which indicated that BUT-306 could be fully regenerated for repeated use in the removal of C3H4 from the C3H4/C3H6 mixture. Breakthrough experiments were also carried out at 273, 313 and 323 K. As shown in Fig. 5c, the final retention times of C3H4 nearly kept unchanged after varying the measurement temperatures. Since BUT-306 shows high hydrolytic stability and hydrophobicity, the effect of water vapor on its C3H4/C3H6 separation performance was also investigated. The breakthrough experiments were carried out with the binary C3H4/C3H6 gas pre-saturated with water vapor (RH ≈ 100% at ambient temperature). It was found that the separation performance of BUT-306 was not lost under such a high humidity (Fig. 5d). The retention time of C3H4 was ∼6 min g−1, about 60% of that under dry conditions. The breakthrough experiment under humid conditions was repeated after regeneration of the MOF, and the two breakthrough curves obtained from the two successive runs well overlapped, suggesting the high and stable separation performance of BUT-306 due to its ultramicroporous, stable and hydrophobic nature.
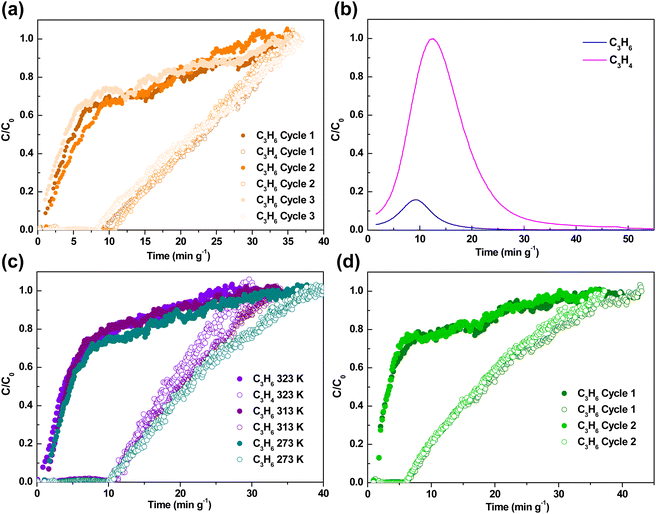 |
| Fig. 5 Dynamic column breakthrough curves of BUT-306 for a binary C3H4/C3H6 (1 : 1, v/v) gas (a) at room temperature, (c) at 273, 313 and 323 K, and (d) under RH ≈ 100% at room temperature, respectively. (b) The MS signal peaks of C3H4 and C3H6 at the outlet when the MOF column was purged with a He gas flow at 393 K after a breakthrough test at room temperature. | |
Conclusions
Two new MOFs BUT-305 and -306 with isoreticular ultramicroporous structures have been synthesized. The pore aperture of BUT-306 (∼1.6 Å) is smaller than that of BUT-305 due to the presence of extra gate-like methyl groups in the channels of the former. This structural feature endows BUT-306 with a high adsorption selectivity of C3H4 over C3H6. The C3H6 and C3H4 adsorption capacities of BUT-306 at 298 K and ∼1 bar were 2.4 and 29.6 cm3 g−1, respectively, indicating a size exclusion adsorption behavior. Based on the single-component gas adsorption isotherms, the IAST C3H4/C3H6 adsorption selectivity at 298 K and 1 bar was calculated to be 636 for an equimolar mixture of C3H4 and C3H6. Dynamic column breakthrough experiments of a binary gas confirmed the high capability of BUT-306 to remove C3H4 from a real C3H4/C3H6 mixture. Thanks to its high hydrolytic stability and hydrophobicity, the C3H4/C3H6 separation performance of BUT-306 was largely retained even when the binary C3H4/C3H6 gas for breakthrough experiments was pre-saturated with water vapor. In addition, the single-crystal structure of C3H4-loaded BUT-306 revealed that the adsorbed C3H4 molecules were located in the center of channel cavities and interacted with the MOF by multiple weak Cδ−⋯Cδ+ dipole–dipole interactions and C–H⋯п interactions. In short, this study reports an ultramicroporous, hydrophobic, and hydrolytically stable MOF with high performance in the removal of C3H4 from a C3H4/C3H6 gas mixture by size exclusion adsorption. Its structure and gas adsorption studies shed light on the design and synthesis of new adsorbents for light hydrocarbon separations.
Experimental section
Materials and characterization
All general reagents and solvents (AR grade) were purchased commercially and used directly. The single-crystal X-ray diffraction data (SCXRD) of BUT-306 (at 273, 298, and 313 K) and C3H4-loaded BUT-306 (at 200 K) were collected on a Rigaku Supernova CCD diffractometer equipped with a graphite-monochromatic enhanced Cu-Kα radiation source (λ = 1.54184 Å). These SCXRD data for BUT-306 have been deposited in the Cambridge Crystallographic Data Centre (CCDC accession numbers: 2168051–2168054†). The PXRD pattern (2θ range: 3–60°) of BUT-305 was measured with a Bruker D8 Advance X-ray powder diffractometer and Pawley refinement55 of the PXRD data was performed in Materials Studio. The peak profiles, background, zero-shift, asymmetry, and unit cell parameters were together refined. The final Pawley refinement results are shown in Fig. 2a and Table S2.† The simulated PXRD pattern was obtained from single-crystal data of BUT-306via the Mercury program.56 The other experimental PXRD data were recorded on a Rigaku SmartLab3 X-ray powder diffractometer that was equipped with a Cu sealed tube (λ = 1.54178 Å) with a scanning rate of 10° min−1. The gas sorption measurements were performed using a Micromeritics ASAP 2020 surface area and pore analyzer.
Synthesis and activation
A mixture of HATZ (18 mg), H2MeTPDC (30 mg) or H2MeTPDC (32 mg) and Zn(CH3COO)2·(90 mg) was dissolved in 18 mL of DMF and placed in a 20 mL glass vial containing 720 μL of 48% aqueous HBF4 solution. Then the mixture sealed in the vial was heated in an oven to 120 °C for 48 hours and the as-synthesized colorless crystals of BUT-305 or -306 were obtained. After the solution was cooled, the crystals were collected and washed several times with CH3OH and dried under vacuum.
The as-synthesized samples of the two MOFs were first soaked in fresh DMF at 60 °C for 12 hours to remove the excess organic ligands and metal salts. Then, these samples were immersed in MeOH for 3 days at 120 °C. In order to ensure the activation effect, the extract was replaced with fresh methanol three times a day. Finally, the samples in MeOH were collected and dried at 120 °C under vacuum for 18 hours for gas adsorption measurements.
Gas adsorption measurements
The N2 adsorption isotherms of BUT-305 and -306 were measured at 77 K, while their water adsorption isotherms were recorded at 298 K. Single-component gas (C2H2, C2H4, C2H6, C3H4, C3H6, and C3H8) adsorption isotherms of the two MOFs were measured at 298 K. In addition, the C3H4 and C3H6 adsorption isotherms of BUT-306 were also recorded at 273, 283, 313 and 323 K, respectively. The C3H4 and C3H6 adsorption isotherms of BUT-306 at 298 were fit by the Langmuir–Freundlich equation, respectively (Fig. S10†). Then, based on the single-component gas adsorption isotherms of BUT-306 for C3H4 and C3H6 at 298 K, ideal adsorbed solution theory (IAST) was used for the prediction of adsorption equilibria of the binary gas mixtures.57 The selectivity of preferential adsorption of component 1 (C3H4) over 2 (C3H6) in a mixture containing 1 and 2 can be formally defined as an equation (eqn (1)), where q1 and q2 are the absolute loadings at the partial pressures p1 and p2, respectively. To calculate the C3H4/C3H6 adsorption selectivity of BUT-306 for gas separation, the gas mixture composition is assumed to be 1
:
99, 1
:
9 and 1
:
1, respectively. |  | (1) |
Breakthrough experiments
The breakthrough experiments for the binary C3H4/C3H6 gas mixture (1
:
1, v/v) were carried out using a setup as shown in Fig. S11.† A powder sample of BUT-306 (330 mg) was packed in a quartz tube (o.d. 6 mm, i.d. 3 mm, and length 150 mm). The temperature of the MOF sample packed in the quartz tube was controlled by a heating jacket (298, 313, 323, and 393 K) or by an ice/water bath (273 K). The gas mixture flow rate was set to be 2 mL min−1 by the mass flow controllers (MFC). The outlet gas compositions from the column were determined continuously using a mass spectrometer (MS, Hiden, HPR-20). For the breakthrough experiments with a humid gas mixture, the C3H4/C3H6 mixture was pre-saturated with water vapor (relative humidity (RH) ≈ 100% at room temperature) by bubbling it through deionized water before it passed through the MOF column. The sample was regenerated for repeated tests under a He flow for 4 hours at 393 K.
Conflicts of interest
The authors declare no conflict of interest.
Acknowledgements
We would like to acknowledge the financial support from the National Natural Science Foundation of China (No. 22038001 and 51621003).
References
- M. F. Friedrich, M. Lucas and P. Claus, Selective hydrogenation of propyne on a solid Pd/Al2O3 catalyst modified with ionic liquid layer (SCILL), Catal. Commun., 2017, 88, 73–76 CrossRef CAS.
- L. Li, R.-B. Lin, R. Krishna, X. Wang, B. Li, H. Wu, J. Li, W. Zhou and B. Chen, Flexible-Robust Metal-Organic Framework for Efficient Removal of Propyne from Propylene, J. Am. Chem. Soc., 2017, 139, 7733–7736 CrossRef CAS PubMed.
- B. Bridier, N. López and J. Pérez-Ramírez, Partial hydrogenation of propyne over copper-based catalysts and comparison with nickel-based analogues, J. Catal., 2010, 269, 80–92 CrossRef CAS.
- R. P. Lively and D. S. Sholl, Seven chemical separations to change the world, Nature, 2016, 532, 435–437 CrossRef PubMed.
- J. Jiang, Z. Lu, M. Zhang, J. Duan, W. Zhang, Y. Pan and J. Bai, Higher Symmetry Multinuclear Clusters of MOFs for Highly Selective CO2 Capture, J. Am. Chem. Soc., 2018, 140, 17825–17829 CrossRef CAS PubMed.
- K. Sumida, D. L. Rogow, J. A. Mason, T. M. McDonald, E. D. Bloch, Z. R. Herm, T.-H. Bae and J. R. Long, Carbon Dioxide Capture in Metal-Organic Frameworks, Chem. Rev., 2012, 112, 724–781 CrossRef CAS PubMed.
- L.-H. Xie and M. P. Suh, High CO2-Capture Ability of a Porous Organic Polymer Bifunctionalized with Carboxy and Triazole Groups, Chem. – Eur. J., 2013, 19, 11590–11597 CrossRef CAS PubMed.
- K.-J. Chen, H. S. Scott, D. G. Madden, T. Pham, A. Kumar, A. Bajpai, M. Lusi, K. A. Forrest, B. Space, J. J. Perry and M. J. Zaworotko, Benchmark C2H2/CO2 and CO2/C2H2 Separation by Two Closely Related Hybrid Ultramicroporous Materials, Chem, 2016, 1, 753–765 CAS.
- H. G. Hao, Y. F. Zhao, D. M. Chen, J. M. Yu, K. Tan, S. Ma, Y. Chabal, Z. M. Zhang, J. M. Dou, Z. H. Xiao, G. Day, H. C. Zhou and T. B. Lu, Simultaneous Trapping of C2H2 and C2H6 from a Ternary Mixture of C2H2 /C2H4 /C2H6 in a Robust Metal-Organic Framework for the Purification of C2H4, Angew. Chem., Int. Ed., 2018, 57, 16067–16071 CrossRef CAS PubMed.
- K. Li, D. H. Olson, J. Seidel, T. J. Emge, H. Gong, H. Zeng and J. Li, Zeolitic imidazolate frameworks for kinetic separation of propane and propene, J. Am. Chem. Soc., 2009, 131, 10368–10369 CrossRef CAS PubMed.
- Z. Zhang, B. Tan, P. Wang, X. Cui and H. Xing, Highly efficient separation of linear and branched C4 isomers with a tailor-made metal–organic framework, AIChE J., 2020, 66, e16236 CAS.
- P. Samaddar, Y.-S. Son, D. C. W. Tsang, K.-H. Kim and S. Kumar, Progress in graphene-based materials as superior media for sensing, sorption, and separation of gaseous pollutants, Coord. Chem. Rev., 2018, 368, 93–114 CrossRef CAS.
- H.-C. J. Zhou and S. Kitagawa, Metal-Organic Frameworks (MOFs), Chem. Soc. Rev., 2014, 43, 5415 RSC.
- Y. Liu, H. Dong, F. Hu and Y. S. Zhao, Metal-organic framework microlasers, Sci. Bull., 2017, 62, 3–4 CrossRef CAS.
- W. G. Cui, T. L. Hu and X. H. Bu, Metal-Organic Framework Materials for the Separation and Purification of Light Hydrocarbons, Adv. Mater., 2020, 32, e1806445 CrossRef PubMed.
- W. Fan, X. Zhang, Z. Kang, X. Liu and D. Sun, Isoreticular chemistry within metal–organic frameworks for gas storage and separation, Coord. Chem. Rev., 2021, 443, 213968–214024 CrossRef CAS.
- R.-B. Lin, S. Xiang, W. Zhou and B. Chen, Microporous Metal-Organic Framework Materials for Gas Separation, Chem, 2020, 6, 337–363 CAS.
- M. R. Tchalala, P. M. Bhatt, K. N. Chappanda, S. R. Tavares, K. Adil, Y. Belmabkhout, A. Shkurenko, A. Cadiau, N. Heymans, G. De Weireld, G. Maurin, K. N. Salama and M. Eddaoudi, Fluorinated MOF platform for selective removal and sensing of SO2 from flue gas and air, Nat. Commun., 2019, 10, 1328–1338 CrossRef CAS PubMed.
- P. Nugent, Y. Belmabkhout, S. D. Burd, A. J. Cairns, R. Luebke, K. Forrest, T. Pham, S. Ma, B. Space, L. Wojtas, M. Eddaoudi and M. J. Zaworotko, Porous materials with optimal adsorption thermodynamics and kinetics for CO2 separation, Nature, 2013, 495, 80–84 CrossRef CAS PubMed.
- V. F. Martins, A. M. Ribeiro, M. G. Plaza, J. C. Santos, J. M. Loureiro, A. F. Ferreira and A. E. Rodrigues, Gas-phase simulated moving bed: Propane/propylene separation on 13X zeolite, J. Chromatogr. A, 2015, 1423, 136–148 CrossRef CAS PubMed.
- F. ZareKarizi, M. Joharian and A. Morsali, Pillar-layered MOFs: functionality, interpenetration, flexibility and applications, J. Mater. Chem. A, 2018, 6, 19288–19329 RSC.
- C. Yu, Q. Ding, J. Hu, Q. Wang, X. Cui and H. Xing, Selective capture of carbon dioxide from humid gases over a wide temperature range using a robust metal–organic framework, Chem. Eng. J., 2021, 405, 126937–126944 CrossRef CAS.
- T. Liu, H. Cui, X. Zhang, Z. Y. Zhang, R. B. Lin, B. Liang, J. Zhang, D. Li and B. Chen, Doubly Interpenetrated Metal-Organic Framework of pcu Topology for Selective Separation of Propylene from Propane, ACS Appl. Mater. Interfaces, 2020, 12, 48712–48717 CrossRef CAS PubMed.
- X. Wang, R. Krishna, L. Li, B. Wang, T. He, Y.-Z. Zhang, J.-R. Li and J. Li, Guest-dependent pressure induced gate-opening effect enables effective separation of propene and propane in a flexible MOF, Chem. Eng. J., 2018, 346, 489–496 CrossRef CAS.
- L. Yang, X. Cui, Q. Ding, Q. Wang, A. Jin, L. Ge and H. Xing, Polycatenated Molecular Cage-Based Propane Trap for Propylene Purification with Recorded Selectivity, ACS Appl. Mater. Interfaces, 2020, 12, 2525–2530 CrossRef CAS PubMed.
- L. Yu, X. Han, H. Wang, S. Ullah, Q. Xia, W. Li, J. Li, I. da Silva, P. Manuel, S. Rudic, Y. Cheng, S. Yang, T. Thonhauser and J. Li, Pore Distortion in a Metal-Organic Framework for Regulated Separation of Propane and Propylene, J. Am. Chem. Soc., 2021, 143, 19300–19305 CrossRef CAS PubMed.
- Z. Zhang, Q. Ding, S. B. Peh, D. Zhao, J. Cui, X. Cui and H. Xing, Mechano-assisted synthesis of an ultramicroporous metal-organic framework for trace CO2 capture, Chem. Commun., 2020, 56, 7726–7729 RSC.
- B. Liang, X. Zhang, Y. Xie, R. B. Lin, R. Krishna, H. Cui, Z. Li, Y. Shi, H. Wu, W. Zhou and B. Chen, An Ultramicroporous Metal-Organic Framework for High Sieving Separation of Propylene from Propane, J. Am. Chem. Soc., 2020, 142, 17795–17801 CrossRef CAS PubMed.
- R. B. Lin, L. Li, H. Wu, H. Arman, B. Li, R. G. Lin, W. Zhou and B. Chen, Optimized Separation of Acetylene from Carbon Dioxide and Ethylene in a Microporous Material, J. Am. Chem. Soc., 2017, 139, 8022–8028 CrossRef CAS PubMed.
- H. Wang, X. Dong, V. Colombo, Q. Wang, Y. Liu, W. Liu, X. L. Wang, X. Y. Huang, D. M. Proserpio, A. Sironi, Y. Han and J. Li, Tailor-Made Microporous Metal-Organic Frameworks for the Full Separation of Propane from Propylene Through Selective Size Exclusion, Adv. Mater., 2018, 30, e1805088 CrossRef PubMed.
- Z. Jiang, L. Fan, P. Zhou, T. Xu, S. Hu, J. Chen, D.-L. Chen and Y. He, An aromatic-rich cage-based MOF with inorganic chloride ions decorating the pore surface displaying the preferential adsorption of C2H2 and C2H6 over C2H4, Inorg. Chem. Front., 2021, 8, 1243–1252 RSC.
- L. Li, H.-M. Wen, C. He, R.-B. Lin, R. Krishna, H. Wu, W. Zhou, J. Li, B. Li and B. Chen, A Metal-Organic Framework with Suitable Pore Size and Specific Functional Sites for the Removal of Trace Propyne from Propylene, Angew. Chem., Int. Ed., 2018, 57, 15183–15188 CrossRef CAS PubMed.
- X.-Q. Wu, J.-H. Liu, T. He, P.-D. Zhang, J. Yu and J.-R. Li, Understanding how pore surface fluorination influences light hydrocarbon separation in metal–organic frameworks, Chem. Eng. J., 2021, 407, 127183–127192 CrossRef CAS.
- L. Yang, X. Cui, Q. Yang, S. Qian, H. Wu, Z. Bao, Z. Zhang, Q. Ren, W. Zhou, B. Chen and H. Xing, Highly Efficient Removal of Propyne from Propylene with Anion-Pillared Ultramicroporous Materials, Adv. Mater., 2018, 30, 1705374–1705382 CrossRef PubMed.
- Z. Zhang, Q. Ding, X. Cui, X. M. Jiang and H. Xing, Fine-Tuning and Selective-Binding within an Anion-Functionalized Ultramicroporous Metal-Organic Framework for Efficient Olefin/Paraffin Separation, ACS Appl. Mater. Interfaces, 2020, 12, 40229–40235 CrossRef CAS PubMed.
- B. Yu, S. Geng, H. Wang, W. Zhou, Z. Zhang, B. Chen and J. Jiang, A Solid Transformation into Carboxyl Dimers Based on a Robust Hydrogen–Bonded Organic Framework for Propyne/Propylene Separation, Angew. Chem., 2021, 133, 26146–26152 CrossRef.
- R. Das, S. S. Dhankhar and C. M. Nagaraja, Construction of a bifunctional Zn(II)–organic framework containing a basic amine functionality for selective capture and room temperature fixation of CO2, Inorg. Chem. Front., 2020, 7, 72–81 RSC.
- S. R. Caskey, A. G. Wong-Foy and A. J. Matzger, Dramatic Tuning of Carbon Dioxide Uptake via Metal Substitution in a Coordination Polymer with Cylindrical Pores, J. Am. Chem. Soc., 2008, 130, 10870–10871 CrossRef CAS PubMed.
- A. Kumar, D. G. Madden, M. Lusi, K. J. Chen, E. A. Daniels, T. Curtin, J. J. T. Perry and M. J. Zaworotko, Direct Air Capture of CO2 by Physisorbent Materials, Angew. Chem., Int. Ed., 2015, 54, 14372–14377 CrossRef CAS PubMed.
- S. D. Burd, S. Ma, J. A. Perman, B. J. Sikora, R. Q. Snurr, P. K. Thallapally, J. Tian, L. Wojtas and M. J. Zaworotko, Highly selective carbon dioxide uptake by [Cu(bpy-n)2(SiF6)] (bpy-1=4,4′-bipyridine; bpy-2=1,2-bis(4-pyridyl)ethene), J. Am. Chem. Soc., 2012, 134, 3663–3666 CrossRef CAS PubMed.
- L. Yang, X. Cui, Y. Zhang, Q. Yang and H. Xing, A highly sensitive flexible metal–organic framework sets a new benchmark for separating propyne from propylene, J. Mater. Chem. A, 2018, 6, 24452–24458 RSC.
- L. Li, L. Guo, F. Zheng, Z. Zhang, Q. Yang, Y. Yang, Q. Ren and Z. Bao, Calcium-Based Metal-Organic Framework for Simultaneous Capture of Trace Propyne and Propadiene from Propylene, ACS Appl. Mater. Interfaces, 2020, 12, 17147–17154 CrossRef CAS PubMed.
- H.-M. Wen, L. Li, R.-B. Lin, B. Li, B. Hu, W. Zhou, J. Hu and B. Chen, Fine-tuning of nano-traps in a stable metal–organic framework for highly efficient removal of propyne from propylene, J. Mater. Chem. A, 2018, 6, 6931–6937 RSC.
- M. Khraisheh, F. AlMomani and G. Walker, High Purity/Recovery Separation of Propylene from Propyne Using Anion Pillared Metal-Organic Framework: Application of Vacuum Swing Adsorption (VSA), Energies, 2021, 14, 609 CrossRef CAS.
- Z. T. Lin, Q. Y. Liu, L. Yang, C. T. He, L. Li and Y. L. Wang, Fluorinated Biphenyldicarboxylate-Based Metal-Organic Framework Exhibiting Efficient Propyne/Propylene Separation, Inorg. Chem., 2020, 59, 4030–4036 CrossRef CAS PubMed.
- Z. Zhang, Q. Ding, J. Cui, X. Cui and H. Xing, Fine-Tuning Pore Dimension in Hybrid Ultramicroporous Materials Boosting Simultaneous Trapping of Trace Alkynes from Alkenes, Small, 2020, 16, e2005360 CrossRef PubMed.
- X. Suo, H. Pan, L. Chen, X. Cui and H. Xing, Control of Functionalized Pore Environment in Robust Ionic Ultramicroporous Polymers for Efficient Removal of Trace Propyne from Propylene, ACS Appl. Mater. Interfaces, 2021, 13, 42706–42714 CrossRef CAS PubMed.
- K.-J. Chen, R.-B. Lin, P.-Q. Liao, C.-T. He, J.-B. Lin, W. Xue, Y.-B. Zhang, J.-P. Zhang and X.-M. Chen, New Zn-Aminotriazolate-Dicarboxylate Frameworks: Synthesis, Structures, and Adsorption Properties, Cryst. Growth Des., 2013, 13, 2118–2123 CrossRef CAS.
- Materials Studio v5.5, Accelrys Inc., San Diego, CA, 2010.
- A. L. Spek, Single-crystal structure validation with the program PLATON, J. Appl. Crystallogr., 2003, 36, 7–13 CrossRef CAS.
- Z. Li, L. Li, L. Guo, J. Wang, Q. Yang, Z. Zhang, Y. Yang, Z. Bao and Q. Ren, Gallate-Based Metal–Organic Frameworks for Highly Efficient Removal of Trace Propyne from Propylene, Ind. Eng. Chem. Res., 2020, 59, 13716–13723 CrossRef CAS.
- Y.-L. Peng, T. Wang, C. Jin, P. Li, S. Suepaul, G. Beemer, Y. Chen, R. Krishna, P. Cheng, T. Pham, B. Space, M. J. Zaworotko and Z. Zhang, A robust heterometallic ultramicroporous MOF with ultrahigh selectivity for propyne/propylene separation, J. Mater. Chem. A, 2021, 9, 2850–2856 RSC.
- J.-P. Zhang and X.-M. Chen, Exceptional Framework Flexibility and Sorption Behavior of a Multifunctional Porous Cuprous Triazolate Framework, J. Am. Chem. Soc., 2008, 130, 6010–6017 CrossRef CAS PubMed.
- S. Ma, D. Sun, D. Yuan, X. Wang and H.-C. Zhou, Preparation and Gas Adsorption Studies of Three Mesh-Adjustable Molecular Sieves with a Common Structure, J. Am. Chem. Soc., 2009, 131, 6445–6451 CrossRef CAS PubMed.
- G. S. Pawley, Unit-Cell REFINEMENT FROM Powder Diffraction Scans, J. Appl. Crystallogr., 1981, 14, 357–361 CrossRef CAS.
- C. F. Macrae, I. Sovago, S. J. Cottrell, P. T. A. Galek, P. McCabe, E. Pidcock, M. Platings, G. P. Shields, J. S. Stevens, M. Towler and P. A. Wood, Mercury 4.0: from visualization to analysis, design and prediction, J. Appl. Crystallogr., 2020, 53, 226–235 CrossRef CAS PubMed.
- A. L. Myers and J. M. Prausnitz, Thermodynamics of mixed-gas adsorption, AIChE J., 1965, 11, 121–127 CrossRef CAS.
|
This journal is © the Partner Organisations 2022 |