Synergy between active sites and electric conductivity of molybdenum sulfide for efficient electrochemical hydrogen production†
Received
29th September 2017
, Accepted 22nd November 2017
First published on 6th December 2017
Abstract
Molybdenum sulfide is a promising non-precious material for electrochemical hydrogen production from water. The number of active sites, the intrinsic activity and the electric conductivity of molybdenum sulfide have a significant influence on hydrogen evolution activity. Poor performance of any of these three factors may hamper the hydrogen evolution activity, so synergy between active sites and electric conductivity is of great importance. Here, we report a scalable wet chemistry method coupled with controllable calcination and the incorporation of carbon nanotubes. In this way, molybdenum sulfides showing optimum synergy between tailored and abundant active sites and high electric conductivity become accessible. Major factors governing the intrinsic catalytic activity could be identified. The optimized molybdenum sulfide based catalyst obtained by this method shows higher activity than sole molybdenum sulfide or molybdenum sulfide modified by either calcination or CNT addition. A low overpotential of 154 mV at a current density of 10 mA cm−2, a low Tafel slope of 31 mV per decade and very good stability were achieved. This versatile approach paves the way for the systematic optimization of various 2D materials utilizing the synergy between active site design and enhanced electric conductivity.
Introduction
Hydrogen (H2) has been considered as an important energy carrier due to its high energy density and environmentally friendly character.1,2 Renewable energies like solar energy and wind energy could be stored in hydrogen through electrochemical water splitting.3,4 As a half reaction of electrochemical water splitting, the hydrogen evolution reaction (HER) plays an important role in transforming renewable energies into hydrogen fuel.5,6 One of the challenges in realizing HER is to develop efficient catalysts to reduce the overpotential.6–8 Although platinum-based catalysts work efficiently,9 an earth-abundant and inexpensive substitute is indispensable to make this process economically feasible.6,7 Since the successful use of molybdenum sulfide (MoSx) as an active HER catalyst in 2005,10 increasing studies have aimed at improved activity and demonstrated MoSx as a promising substitute for platinum.7,11,12 Active sites and the electric conductivity of MoSx are crucial for HER activity.7,13 Sulfur monomers on the edge of the molybdenum disulfide layer are identified as active sites for HER,10,14,15 and the catalytically inert basal plane could be activated by creating sulfur vacancies.16 Sulfur dimers on edge-like sites of amorphous molybdenum sulfide are also active after activation by in situ elimination of sulfur and cleavage of disulfide bonds.17,18 Thus, lots of investigations have focused on enhanced exposure of active sites, including sulfur monomers on edge sites, sulfur dimers on edge-like sites and defects on the basal plane, to achieve higher activity.8,12,16,19–25 Additionally, the intrinsic activity is also of great importance for high HER activity.16,24 Doping MoSx with vanadium, oxygen and nitrogen13,26,27 or coupling MoSx with conductive materials like carbon nanotubes and graphene28–37 increases the electric conductivity and therewith the observed HER activity. Since the number of active sites, the intrinsic activity and the electric conductivity are crucial for HER, poor performance of any of these three factors may hamper the HER performance. Accordingly, the synergy between active sites and electric conductivity plays a major role in optimizing the HER performance of MoSx. Disorder engineering13 and carbon-based materials28,36 have been used to achieve such synergy; however, an improved facile method for exploring the optimum synergy among abundant active sites, high intrinsic activity and high electric conductivity for high HER activity is still required.
Herein, we report a wet chemistry method coupled with calcination and the incorporation of carbon nanotubes (CNT) to synthesize MoSx and to unravel the optimum synergy between active sites and electric conductivity. Synthesis of MoSx by precipitation with excessive sulfur at low temperature creates high exposure of active sites with high intrinsic activity. Proper calcination enables high exposure of active sites and introduces a certain layer structure, which facilitates intra-domain charge transfer. The addition of conductive CNT by simply mixing them in with the catalyst ink accelerates the inter-domain charge transfer and increases the intrinsic activity. Overall, with material design, tailored calcination and the addition of CNT, the obtained synergy between active sites and electric conductivity taps more active sites working with higher intrinsic activity. The optimized catalyst shows a low overpotential of 154 mV at a current density of 10 mA cm−2, a Tafel slope of 31 mV dec−1 and good stability. The optimized catalyst obtained by this facile approach outperforms simple CNT incorporation without integrated material optimization and provides comparable activity to molybdenum sulfide supported on CNT.36,37 In line with the above, this study provides a scalable and economical synthesis method for efficient HER catalysts which is potentially broadly applicable to other 2D materials for HER.
Experimental
Material synthesis
Molybdenum sulfides, MoSx (x = 1.9–3.7), were prepared by the method reported in ref. 38 with only a little modification. Generally, 8 mL of 37% hydrochloric acid (Fluka) was diluted in 80 mL deionized water. A solution of 0.88 g (NH4)6Mo7O24·4H2O (Fluka) in 5 mL deionized water and a solution of 2.64 g Na2S·9H2O (Sigma-Aldrich) in 5 mL deionized water were added to the hydrochloric acid solution under stirring. 5 mL deionized water was used to wash the (NH4)6Mo7O24·4H2O and Na2S·9H2O residues and was added to the hydrochloric acid solution. A dark-brown slurry was formed after mixing. The slurry was kept in an oil bath at 80 °C under stirring and reflux. After 0.5 h, 0.7 g HONH2·HCl (Sigma-Aldrich) was added to the slurry. After one more hour, the slurry was filtered and washed with deionized water. The filtrate was dried under atmospheric conditions followed by calcination at varied temperatures. The obtained materials were labeled M80, M300, M500 and M700 for the as-precipitated material and materials calcined at 300 °C, 500 °C and 700 °C in a nitrogen atmosphere, respectively.
Material characterization
X-ray diffraction (XRD) was performed using a Bruker D8 Advance diffractometer with Cu Kα radiation (λ = 0.15406 nm) at 40 kV and 20 mA. The elemental composition of MoSx was analyzed with a SPECTROBLUE ICP-OES (inductively coupled plasma optical emission spectrometer). Scanning electron microscopy (SEM) images were taken using a Hitachi SU8020 microscope at 2 kV. Transmission electron microscopy (TEM) images were taken using an FEI Tecnai F20 microscope with an accelerating voltage of 200 kV. High resolution TEM (HRTEM) and selected-area electron diffraction (SAED) measurements were performed on the same machine with an accelerating voltage of 200 kV. Raman spectroscopy was performed using a WITec Alpha 300R microscope with a 532 nm excitation laser. X-ray photoelectron spectroscopy (XPS) was carried out using a Thermo Fisher-VG ESCALAB 250 spectrometer with Mg Kα as the excitation source at a power of 150 W. The binding energies were referenced to C 1s at 284.6 eV.
Electrochemical measurements
Electrochemical measurements were performed using an Autolab PGSTAT 302 N electrochemical workstation with a three-electrode cell. A glassy carbon rod and Ag/AgCl (in 3 M KCl solution) were used as the counter electrode and the reference electrode, respectively. A rotating disk electrode (glassy carbon electrode, 4 mm diameter, 0.126 cm2) modified with a catalyst was used as the working electrode. Typically, 3 mg MoSx was mixed with 500 μL Nafion solution (49 v% H2O, 49 v% ethanol and 2 v% Nafion solution (5%, Aldrich)) followed by a treatment in an ultrasonic bath to obtain a well-dispersed suspension. 5 μL catalyst ink was dropped onto the glassy carbon electrode to obtain a catalyst loading of 0.24 mg cm−2 and was dried under atmospheric conditions. For catalysts tested with CNT, 0.5 mg CNT were also added into the catalyst ink. Multiwall carbon nanotubes (Aldrich) were treated in 69% nitric acid (Sigma-Aldrich) at room temperature before use. In this way, MoSx and CNT were mixed well, and no significant change in the morphology of MoSx and CNT was found (SEM images are presented in Fig. S1†). Mixtures of MoSx and CNT were labeled MoSx-C. A platinum rotating disk electrode was also tested as a reference. The measurements were carried out at room temperature in a 0.5 M H2SO4 solution (pH ≈ 0.35). Cyclic voltammetry (CV) scans ranging from 71 mV versus RHE (reversible hydrogen electrode) to −280 mV versus RHE with a rotating speed of 2500 rpm were conducted to evaluate the activity and the stability of the catalyst. 100 CV scans with a scan rate of 100 mV s−1, a CV scan with a scan rate of 5 mV s−1, 1000 CV scans with a scan rate of 100 mV s−1 and a CV scan with a scan rate of 5 mV s−1 were conducted in sequence to stabilize the CV curves, to collect the polarization curves (negative potential sweep), to evaluate the catalyst stability and to collect the polarization curves after the stability test, respectively. We also carried out chronoamperometry and chronopotentiometry measurements at a potential of −179 mV versus RHE and a current density of −10 mA cm−2, respectively, to study the electrochemical stability. Double layer capacitances were measured by CV scans at various scan rates in the potential range of 120 mV versus RHE to 320 mV versus RHE. Electrochemical impedance spectroscopy (EIS) was performed at an overpotential of 179 mV with an amplitude of the sinusoidal voltage perturbation of 10 mV over a frequency range of 100 kHz to 50 mHz. The potentials were referenced to RHE calculated using the formula VRHE (V) = VAg/AgCl + 0.21 + 0.059 × pH. The polarization curves were corrected with IR- and background-correction.
Results and discussion
Synthesis of MoSx
Molybdenum sulfides, MoSx (x = 1.9–3.7, elemental analysis results are shown in Table S1†), were synthesized by precipitation of (NH4)6Mo7O24·4H2O with excessive Na2S·9H2O followed by calcination at elevated temperature.38 By precipitation at 80 °C (M80) and calcination at 300 °C (M300), 500 °C (M500) and 700 °C (M700), MoSx with tailored exposure, different nature of active sites, and varying electric conductivity were obtained for HER studies. Fig. 1a presents the XRD patterns of MoSx. M80 and M300 have broad overlapping reflexes, while M500 and M700 show sharp reflexes in agreement with 2H-MoS2 (JCPDS 01-073-1508). This indicates a structure evolution from amorphous MoS3.7 to crystallized MoS1.9 with increasing calcination temperature. The SEM images (Fig. 1b and Fig. S2†) and TEM images (Fig. 1c and Fig. S3†) also prove this structural evolution. The as-precipitated M80 exhibits deformed nano-spheres (Fig. 1b1) consisting of disordered short-range chains (Fig. 1c1). The formation of a layer structure starts at 300 °C (Fig. 1c2). At higher calcination temperatures, nano-plates (Fig. 1b3 and b4) with an ordered layer structure (Fig. 1c3 and c4) are formed. The SAED images (Fig. S4†) further confirm the transformation from an amorphous structure into a well-crystallized structure. Fig. 1d summarizes the Raman spectra of the MoSx series. M80 shows Raman signatures typical of amorphous MoSx.39–41 The bands are assigned to ν(S–S) vibrations of terminal S22− ligands and bridging S22− ligands, ν(Mo3–μS) vibrations and ν(Mo–S) vibrations, respectively. After calcination at 300 °C, the in-plane Mo–S phonon mode (E2g1) and out-of-plane Mo–S mode (A1g) occur consistent with the formation of a MoS2 layer structure visible in the HRTEM image (Fig. 1c2).20,21 The sharp peaks of E2g1 and A1g modes in M500 and M700 indicate a well-crystallized layer structure consistent with the XRD and HRTEM results. Fig. 1e shows the S 2p XPS spectra and peak fittings of MoSx (Mo 3d XPS spectra are shown in Fig. S5†). We fit the S 2p XPS spectra to three sets of peaks. The first set is S 2p of SO42− (yellow area); the second includes S 2p3/2 and S 2p1/2 of terminal S22− and S2− (low-binding-energy peaks, red area); the third includes S 2p3/2 and S 2p1/2 of bridging S22− and apical S2− (high-binding-energy peaks, blue area).40,42–44 Combining the Raman spectra with the XPS quantification results (Table S1†), we conclude that terminal S22− and bridging S22− transform into S2− with increasing calcination temperature. The conversion of sulfur dimers into sulfur monomers is further emphasized by the activation process during electrochemical measurements (Fig. S6†). M80 and M300 (either without or with CNT) show increasing activity during the first scans because of the elimination of sulfur and cleavage of disulfide bonds,17,18 while M500 and M700 (either without or with CNT) show slightly decreasing activity.
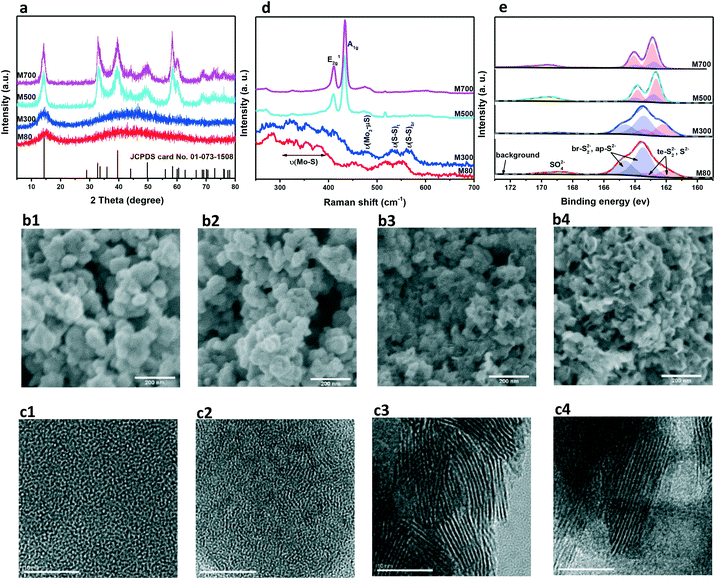 |
| Fig. 1 Characterization of MoSx. (a) XRD patterns of MoSx and 2H-MoS2 (JCPDS 01-073-1508). (b) SEM images of M80 (b1), M300 (b2), M500 (b3) and M700 (b4). Scale bars, 200 nm. (c) HR-TEM images of M80 (c1), M300 (c2), M500 (c3) and M700 (c4). Scale bars, 10 nm. (d) Raman spectra of MoSx. (e) S 2p XPS spectra and peak fittings of MoSx. Solid lines are experimental results and dashed lines are fitting results in (e). | |
Summarizing the characterization results, we propose a possible process for the evolution of M80 to M700 (Fig. 2). The as-precipitated amorphous MoS3.7 (M80) exhibits deformed nano-spheres composed of short-range disordered chains. The small domains expose a lot of sulfur dimers (terminal S22− and bridging S22−) on edge-like sites. With increasing calcination temperature, the deformed nano-spheres with short-range disordered chains evolve into nano-plates with a long-range ordered layer structure, accompanied by the elimination of sulfur, reduced exposure of the edge-like sites and/or edge sites due to the bigger domain size and the conversion of sulfur dimers into sulfur monomers. At the same time, the electric conductivity increases, because the long-range ordered structure enhances the electron transport. Thus, our preparation strategy provided a series of MoSx with tailored exposure, different nature of active sites, and varying electric conductivity.
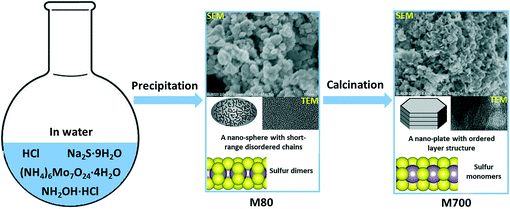 |
| Fig. 2 The formation of M80 and a possible evolution process of M80 to M700. | |
Electrocatalytic HER performances
To investigate the individual contribution to HER activity of the number and nature of active sites as well as electric conductivity and their synergy, we tested the HER activity of the MoSx series. All the measurements were conducted in a typical three-electrode cell in 0.5 M H2SO4 electrolyte. The polarization curves were treated with IR- and background-correction (Fig. S7†).45Fig. 3a shows the polarization curves of MoSx. The HER activity of MoSx increases with increasing calcination temperature from 80 °C to 500 °C. As the calcination temperature increases to 700 °C, the activity decreases. The reason is that increasing calcination temperature reduces the exposure of edge-like sites and/or edge sites and increases the electric conductivity. At low calcination temperature, the HER activity is hampered by the slow charge transfer due to the poor electric conductivity. At high calcination temperature, the HER activity is hampered by the sparse exposure of active sites. M500 achieves a compromise concerning the number of active sites and electric conductivity resulting in the highest activity of this series. To explore the optimum synergy between abundant active sites and high electric conductivity, carbon nanotubes (CNT) were incorporated during catalyst ink preparation followed by an ultrasonic treatment (labelled MoSx-C). The polarization curves of CNT and MoSx-C are presented in Fig. 3b. Sole CNT possess almost no HER activity; however, the incorporation of CNT increases the activity of M80-C and M300-C significantly but shows a slight influence on the activity of M500-C and M700-C. The current densities at an overpotential of 200 mV increase about 137 times and 80 times for M80-C and M300-C, respectively. CNT raise the electric conductivity of M80-C and M300-C, enabling the fast charge transfer to tap more active sites working. M300-C exhibits the lowest η10 (overpotential at a current density of 10 mA cm−2) of 167 mV. We assign this finding to the optimum synergy between abundant active sites available due to the small domain size of the material and the high electric conductivity enabled by the formation of some layer structures and the incorporation of CNT. The slope of the log
j–η plot (Tafel plot) provides information on the overpotential needed to increase the current density 10 fold and to elucidate the reaction mechanism (Note S1†).8 The Tafel slopes of 96 mV dec−1, 64 mV dec−1, 63 mV dec−1 and 77 mV dec−1 for M80, M300, M500 and M700 (Fig. 3c) suggest that the rate-determining steps correspond to the Volmer or Heyrovsky reaction. After the incorporation of CNT, the decrease of the Tafel slopes of M80-C and M300-C to 43 mV dec−1 and 35 mV dec−1 indicates changes of the rate determining steps to the Heyrovsky or Tafel reaction. Interestingly, CNT do not significantly affect the Tafel slopes and the rate-determining steps of M500-C and M700-C. We optimized the loading of M300-C (Fig. S8†) and obtained an η10 of 154 mV and a Tafel slope of 31 mV dec−1, which are in line with literature benchmarks of molybdenum sulfide-based catalysts reported in the literature (Table S2†). However, its activity is lower than that of platinum. A possible explanation is a lower atom utilization of only 25% compared to Pt with 100%.14 Future studies will focus on this point. It is important to mention that M300-C exhibits high stability. η10 increases by less than 1 mV after 1000 CV scans, and only a slight activity decrease was observed after 19 h electrochemical measurement (Fig. S8†). This is further evidenced by SEM as no significant morphology change was found after 19 h electrochemical measurement (Fig. S1h†).
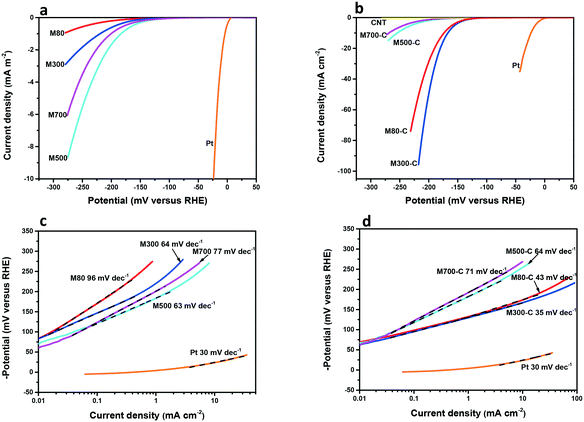 |
| Fig. 3 Electrocatalytic HER performances of MoSx and MoSx-C. (a) HER polarization curves of MoSx in comparison with Pt. (b) HER polarization curves of MoSx-C in comparison with CNT and Pt. (c) Tafel plots of MoSx derived from a. (d) Tafel plots of MoSx-C derived from b. Solid lines are experimental results, and dashed lines are fitting results in (c) and (d). All the measurements were performed in 0.5 M H2SO4 electrolyte. The polarization curves and the Tafel plots were IR- and background-corrected. Catalyst loading, 0.24 mg MoSx cm−2. Sweep rate, 5 mV s−1. | |
HER activation mechanism
To study the origin of increased HER activity of certain MoSx upon CNT addition, we measured the charge transfer resistance (Rct), the electrochemical double layer capacitance (Cdl) and the turnover frequency (TOF). Nyquist plots of MoSx (Fig. 4a) and MoSx-C (Fig. 4b) were obtained by electrochemical impedance spectroscopy (EIS). A two time constant equivalent circuit (Fig. S9†) was used to extract Rct (Table 1).44 Conductive M500 and M700 exhibit smaller Rct than poorly conductive M80 and M300. The incorporation of CNT reduces the Rct of M80-C and M300-C about 170 times and 120 times but shows a minor influence on that of M500-C and M700-C. The lowest Rct of 4.7 Ω is obtained for M300-C. We measured Cdl to evaluate the electrochemically active surface area by performing CV at different scan rates (Fig. S10†).13Cdl values were extracted by linear regression (Fig. 4c and d), and the results are listed in Table 1. Without CNT, M500 and M700 show higher Cdl than M80 and M300. After incorporation of CNT, the Cdl of M80-C and M300-C increases about 43 and 13 times to 16.35 mF cm−2 and 15.29 mF cm−2, but the Cdl of M500-C and M700-C stays almost constant. We estimated the electrochemically active surface area and calculated the TOFs of the catalysts with Cdl results (Note S2†).46 The TOF curves of MoSx and MoSx-C are illustrated in Fig. 4e and f, and the TOFs at an overpotential of 175 mV are shown in Table 1. Without CNT, MoSx reaches similar TOFs at 175 mV ranging from 0.029 s−1 to 0.048 s−1. After the incorporation of CNT, the TOFs of M80-C and M300-C increase about 2.2 times and 4.0 times, while those of M500-C and M700-C only change slightly. The increase in TOF could additionally be derived from the weakened adsorption capacity of H atoms due to the CNT–MoSx interface. The weakened adsorption facilitates the H recombination and release in HER leading to higher intrinsic activity.47
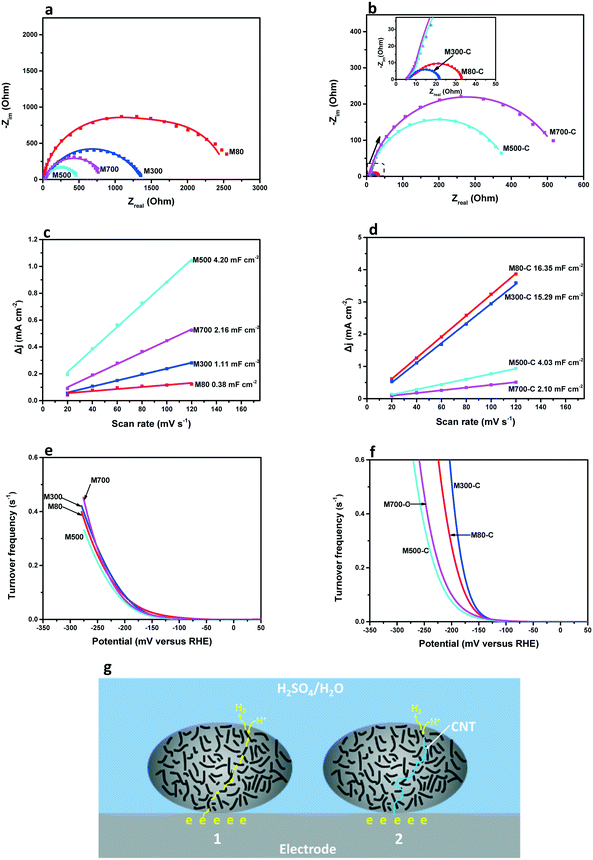 |
| Fig. 4 HER activation mechanism. (a) EIS Nyquist plots of MoSx. (b) EIS Nyquist plots of MoSx-C. The inset in (b) shows Nyquist plots at a high frequency range. Zreal is the real impedance, and Zim is the imaginary impedance. (c) Charging current density differences at different scan rates and Cdl fittings of MoSx. (d) Charging current density differences at different scan rates and Cdl fittings of MoSx-C. Square points are the experimental results, and solid lines are the fitting results in a, b, c and d. (e) TOFs at varied overpotentials of MoSx. (f) TOFs at varied overpotentials of MoSx-C. (g) Scheme of HER enhancement mechanism. Without (1) and with CNT (2). All the measurements were performed in 0.5 M H2SO4 electrolyte. The TOF curves, e and f, were IR- and background-corrected. | |
Table 1 Charge transfer resistances, double layer capacitances and turnover frequencies of MoSx and MoSx-C
|
R
ct
(Ω) |
C
dl
(mF cm−2) |
TOFc (s−1) |
Without CNT |
With CNT |
Without CNT |
With CNT |
Without CNT |
With CNT |
R
ct, charge transfer resistance.
C
dl, double layer capacitance.
TOF, turnover frequency at 175 mV.
|
M80 |
1882.0 |
10.7 |
0.38 |
16.35 |
0.048 |
0.104 |
M300 |
598.7 |
4.7 |
1.11 |
15.29 |
0.041 |
0.162 |
M500 |
60.5 |
12.8 |
4.20 |
4.03 |
0.029 |
0.032 |
M700 |
86.6 |
17.6 |
2.16 |
2.10 |
0.035 |
0.044 |
Consequently, the observed increased HER activity of certain materials upon combination with CNT can be assigned to synergetic effects between tailored active sites, facilitated desorption and enhanced electric conductivity. Synthesis of MoSx by precipitation with excessive sulfur at low temperature enables high exposure of active sites with high intrinsic activity. However, the slow charge transfer due to the poor electric conductivity hampers the efficient use of the active sites resulting in an overall low apparent activity. Calcination at low temperature on the one hand keeps a relatively high exposure of active sites, but on the other hand introduces some layer structures facilitating intra-domain charge transfer. The incorporation of conductive CNT accelerates the inter-domain charge transfer and increases the intrinsic activity. Through material design, proper calcination and the incorporation of CNT, synergy among abundant active sites, high intrinsic activity and high electric conductivity is obtained. The synergy taps more active sites working with high intrinsic activity. Considering the reduction of protons on amorphous M80 or M300 at a site far away from the electrode, electrons have to jump from domain to domain to combine with protons at the active site (Fig. 4g1). Due to the poor electric conductivity of M80 and M300, the electrons transfer very slowly (high Rct). Only sites near the electrode are electrochemically active (low Cdl). The slow electron transfer hampers the efficient use of active sites, leading to low HER activity. After the incorporation of CNT, electrons could go through the CNT to sites far away from the electrode quickly (Fig. 4g2). The promoted electron transfer (low Rct) enhances the accessibility of active sites (high Cdl). In line with this, CNT have a more positive influence on the intrinsic activity of active sites in the amorphous structure than in the crystalized structure. The HER activity of M500 and M700 is restricted by the spare exposure of active sites, so CNT have a slight influence on it. Instead, M300 calcined at low temperature has abundant active sites with some layer structures, which facilitates intra-domain charge transfer. The incorporation of CNT accelerates the inter-domain charge transfer and increases the intrinsic activity. The synergy between active sites and electric conductivity and the best HER performance are achieved by finely controlled calcination coupled with CNT addition.
Conclusions
Herein, we report a scalable wet chemistry method combined with controllable calcination and the use of carbon nanotubes as additives, enabling synergy between the active sites and electric conductivity of a molybdenum sulfide based catalyst for efficient hydrogen evolution. Synthesis of MoSx by precipitation with excessive sulfur creates high exposure of active sites with high intrinsic activity. By proper calcination, the high exposure of the active sites is maintained, and the formation of some layer structure facilitates intra-domain charge transfer. The use of CNT by simply mixing them in with the catalyst ink accelerates the inter-domain charge transfer and increases the intrinsic activity. By calcination at 300 °C and the use of conductive CNT as additives, synergy between the number and nature of active sites and electric conductivity was obtained, enabling efficient accessibility of the active sites to provide high intrinsic activity. Following this approach, we obtained a highly active and stable HER catalyst with an overpotential of 154 mV (at 10 mA cm−2) and a Tafel slope of 31 mV dec−1. Our work provides a promising HER catalyst together with an easy and versatile method for improving the HER activity of various two-dimensional materials.
Author contributions
Regina Palkovits conceived the project, supervised the research and supported data interpretation. Feng Zeng performed material synthesis, material characterization and electrochemical measurements, and wrote the manuscript. Cornelia Broicher helped with the electrochemical measurements. Stefan Palkovits helped with building up the electrochemical measurement system and gave helpful suggestions about the experiment. Kalin Simeonov provided suggestions for material synthesis. All authors read and commented on the manuscript.
Conflicts of interest
There are no conflicts to declare.
Acknowledgements
We thank Fabian Joschka Holzhäuser for his help with the electrochemical experiments. We thank Marc Drögeler for his help with Raman spectroscopy. Feng Zeng thanks the China Scholarship Council for financial support. Regina Palkovits and Cornelia Broicher acknowledge the Federal Ministry of Education and Research (BMBF) for funding part of this work with the MANGAN research cluster (FKZ 03SF0508).
Notes and references
- K. T. Møller, T. R. Jensen, E. Akiba and H. Li, Hydrogen-A sustainable energy carrier, Prog. Nat. Sci.: Mater. Int., 2017, 27(1), 34–40 CrossRef.
- J. A. Turner, A realizable renewable energy future, Science, 1999, 285(5428), 687–689 CrossRef CAS PubMed.
- M. R. Shaner, H. A. Atwater, N. S. Lewis and E. W. McFarland, A comparative technoeconomic analysis of renewable hydrogen production using solar energy, Energy Environ. Sci., 2016, 9(7), 2354–2371 CAS.
- S. A. Sherif, F. Barbir and T. N. Veziroglu, Wind energy and the hydrogen economy-Review of the technology, Sol. Energy, 2005, 78(5), 647–660 CrossRef CAS.
- S. Anantharaj, S. R. Ede, K. Sakthikumar, K. Karthick, S. Mishra and S. Kundu, Recent trends and perspectives in electrochemical water splitting with an emphasis on sulfide, selenide, and phosphide catalysts of Fe, Co, and Ni: A review, ACS Catal., 2016, 6(12), 8069–8097 CrossRef CAS.
- I. Roger, M. A. Shipman and M. D. Symes, Earth-abundant catalysts for electrochemical and photoelectrochemical water splitting, Nat. Rev. Chem, 2017, 1, 0003 CrossRef CAS.
- X. Zou and Y. Zhang, Noble metal-free hydrogen evolution catalysts for water splitting, Chem. Soc. Rev., 2015, 44(15), 5148–5180 RSC.
- C. G. Morales-Guio, L.-A. Stern and X. Hu, Nanostructured hydrotreating catalysts for electrochemical hydrogen evolution, Chem. Soc. Rev., 2014, 43(18), 6555–6569 RSC.
- S. Trasatti, Work function, electronegativity, and electrochemical behaviour of metals: III. Electrolytic hydrogen evolution in acid solutions, J. Electroanal. Chem. Interfacial Electrochem., 1972, 39(1), 163–184 CrossRef CAS.
- B. Hinnemann, P. G. Moses, J. Bonde, K. P. Jørgensen, J. H. Nielsen and S. Horch,
et al., Biomimetic hydrogen evolution: MoS2 nanoparticles as catalyst for hydrogen evolution, J. Am. Chem. Soc., 2005, 127(15), 5308–5309 CrossRef CAS PubMed.
- A. B. Laursen, S. Kegnæs, S. Dahl and I. Chorkendorff, Molybdenum sulfides-efficient and viable materials for electro-and photoelectrocatalytic hydrogen evolution, Energy Environ. Sci., 2012, 5(2), 5577–5591 CAS.
- D. Merki and X. Hu, Recent developments of molybdenum and tungsten sulfides as hydrogen evolution catalysts, Energy Environ. Sci., 2011, 4(10), 3878–3888 CAS.
- J. Xie, J. Zhang, S. Li, F. Grote, X. Zhang and H. Zhang,
et al., Controllable disorder engineering in oxygen-incorporated MoS2 ultrathin nanosheets for efficient hydrogen evolution, J. Am. Chem. Soc., 2013, 135(47), 17881–17888 CrossRef CAS PubMed.
- T. F. Jaramillo, K. P. Jørgensen, J. Bonde, J. H. Nielsen, S. Horch and I. Chorkendorff, Identification of active edge sites for electrochemical H2 evolution from MoS2 nanocatalysts, Science, 2007, 317(5834), 100–102 CrossRef CAS PubMed.
- P. Lazar and M. Otyepka, Role of the edge properties in the hydrogen evolution reaction on MoS2, Chem. – Eur. J., 2017, 23(20), 4863–4869 CrossRef CAS PubMed.
- H. Li, C. Tsai, A. L. Koh, L. Cai, A. W. Contryman and A. H. Fragapane,
et al., Activating and optimizing MoS2 basal planes for hydrogen evolution through the formation of strained sulphur vacancies, Nat. Mater., 2016, 15(1), 48–53 CrossRef CAS PubMed.
- H. Vrubel, D. Merki and X. Hu, Hydrogen evolution catalyzed by MoS3 and MoS2 particles, Energy Environ. Sci., 2012, 5(3), 6136–6144 CAS.
- Y. Deng, L. R. L. Ting, P. H. L. Neo, Y.-J. Zhang, A. A. Peterson and B. S. Yeo, Operando Raman spectroscopy of amorphous molybdenum sulfide (MoSx) during the electrochemical hydrogen evolution reaction: Identification of sulfur atoms as catalytically active sites for H+ reduction, ACS Catal., 2016, 6(11), 7790–7798 CrossRef CAS.
- J. Kibsgaard, Z. Chen, B. N. Reinecke and T. F. Jaramillo, Engineering the surface structure of MoS2 to preferentially expose active edge sites for electrocatalysis, Nat. Mater., 2012, 11(11), 963–969 CrossRef CAS PubMed.
- M.-R. Gao, M. K. Chan and Y. Sun, Edge-terminated molybdenum disulfide with a 9.4-Å interlayer spacing for electrochemical hydrogen production, Nat. Commun., 2015, 6, 7493 CrossRef PubMed.
- D. Y. Chung, S.-K. Park, Y.-H. Chung, S.-H. Yu, D.-H. Lim and N. Jung,
et al., Edge-exposed MoS2 nano-assembled structures as efficient electrocatalysts for hydrogen evolution reaction, Nanoscale, 2014, 6(4), 2131–2136 RSC.
- D. Wang, Z. Wang, C. Wang, P. Zhou, Z. Wu and Z. Liu, Distorted MoS2 nanostructures: An efficient catalyst for the electrochemical hydrogen evolution reaction, Electrochem. Commun., 2013, 34, 219–222 CrossRef.
- D. Gopalakrishnan, D. Damien and M. M. Shaijumon, MoS2 quantum dot-interspersed exfoliated MoS2 nanosheets, ACS Nano, 2014, 8(5), 5297–5303 CrossRef CAS PubMed.
- J. Xie, H. Zhang, S. Li, R. Wang, X. Sun and M. Zhou,
et al., Defect-rich MoS2 ultrathin nanosheets with additional active edge sites for enhanced electrocatalytic hydrogen evolution, Adv. Mater., 2013, 25(40), 5807–5813 CrossRef CAS PubMed.
- C. Tsai, H. Li, S. Park, J. Park, H. S. Han, J. K. Nørskov, X. Zheng and F. Abild-Pedersen, Electrochemical generation of sulfur vacancies in the basal plane of MoS2 for hydrogen evolution, Nat. Commun., 2017, 8, 15113 CrossRef PubMed.
- X. Sun, J. Dai, Y. Guo, C. Wu, F. Hu and J. Zhao,
et al., Semimetallic molybdenum disulfide ultrathin nanosheets as an efficient electrocatalyst for hydrogen evolution, Nanoscale, 2014, 6(14), 8359–8367 RSC.
- W. Zhou, D. Hou, Y. Sang, S. Yao, J. Zhou and G. Li,
et al., MoO2 nanobelts@nitrogen self-doped MoS2 nanosheets as effective electrocatalysts for hydrogen evolution reaction, J. Mater. Chem. A, 2014, 2(29), 11358–11364 CAS.
- Y. Li, H. Wang, L. Xie, Y. Liang, G. Hong and H. Dai, MoS2 nanoparticles grown on graphene: An advanced catalyst for the hydrogen evolution reaction, J. Am. Chem. Soc., 2011, 133(19), 7296–7299 CrossRef CAS PubMed.
- X. Zheng, J. Xu, K. Yan, H. Wang, Z. Wang and S. Yang, Space-confined growth of MoS2 nanosheets within graphite: The layered hybrid of MoS2 and graphene as an active catalyst for hydrogen evolution reaction, Chem. Mater., 2014, 26(7), 2344–2353 CrossRef CAS.
- E. G. Firmiano, M. A. Cordeiro, A. C. Rabelo, C. J. Dalmaschio, A. N. Pinheiro and E. C. Pereira,
et al., Graphene oxide as a highly selective substrate to synthesize a layered MoS2 hybrid electrocatalyst, Chem. Commun., 2012, 48(62), 7687–7689 RSC.
- Y. Yan, X. Ge, Z. Liu, J.-Y. Wang, J.-M. Lee and X. Wang, Facile synthesis of low crystalline MoS2 nanosheet-coated CNTs for enhanced hydrogen evolution reaction, Nanoscale, 2013, 5(17), 7768–7771 RSC.
- Y. Yan, B. Xia, X. Qi, H. Wang, R. Xu and J.-Y. Wang,
et al., Nano-tungsten carbide decorated graphene as co-catalysts for enhanced hydrogen evolution on molybdenum disulfide, Chem. Commun., 2013, 49(43), 4884–4886 RSC.
- J. Deng, W. Yuan, P. Ren, Y. Wang, D. Deng and Z. Zhang,
et al., High-performance hydrogen evolution electrocatalysis by layer-controlled MoS2 nanosheets, RSC Adv., 2014, 4(66), 34733–34738 RSC.
- H. Zhu, M. Du, M. Zhang, M. Zou, T. Yang and Y. Fu,
et al., The design and construction of 3D rose-petal-shaped MoS2 hierarchical nanostructures with structure-sensitive properties, J. Mater. Chem. A, 2014, 2(21), 7680–7685 CAS.
- D. H. Youn, S. Han, J. Y. Kim, J. Y. Kim, H. Park and S. H. Choi,
et al., Highly active and stable hydrogen evolution electrocatalysts based on molybdenum compounds on carbon nanotube-graphene hybrid support, ACS Nano, 2014, 8(5), 5164–5173 CrossRef CAS PubMed.
- D. J. Li, U. N. Maiti, J. Lim, D. S. Choi and W. J. Lee, Oh Y, et al., Molybdenum sulfide/N-doped CNT forest hybrid catalysts for high-performance hydrogen evolution reaction, Nano Lett., 2014, 14(3), 1228–1233 CrossRef CAS PubMed.
- D. Voiry, M. Salehi, R. Silva, T. Fujita, M. Chen and T. Asefa,
et al., Conducting MoS2 nanosheets as catalysts for hydrogen evolution reaction, Nano Lett., 2013, 13(12), 6222–6227 CrossRef CAS PubMed.
- L. Chang, H. Yang, W. Fu, J. Zhang, Q. Yu and H. Zhu,
et al., Simple synthesis of MoS2 inorganic fullerene-like nanomaterials from MoS2 amorphous nanoparticles, Mater. Res. Bull., 2008, 43(8), 2427–2433 CrossRef CAS.
- T. Weber, J. C. Muijsers and J. W. Niemantsverdriet, Structure of amorphous MoS3, J. Phys. Chem., 1995, 99(22), 9194–9200 CrossRef CAS.
- Y. Li, Y. Yu, Y. Huang, R. A. Nielsen, W. A. Goddard III and Y. Li,
et al., Engineering the composition and crystallinity of molybdenum sulfide for high-performance electrocatalytic hydrogen evolution, ACS Catal., 2014, 5(1), 448–455 CrossRef.
- P. D. Tran, T. V. Tran, M. Orio, S. Torelli, Q. D. Truong and K. Nayuki,
et al., Coordination polymer structure and revisited hydrogen evolution catalytic mechanism for amorphous molybdenum sulfide, Nat. Mater., 2016, 15(6), 640–646 CrossRef CAS PubMed.
- L. Qiu and G. Xu, Peak overlaps and corresponding solutions in the X-ray photoelectron spectroscopic study of hydrodesulfurization catalysts, Appl. Surf. Sci., 2010, 256(11), 3413–3417 CrossRef CAS.
- H. W. Wang, P. Skeldon and G. E. Thompson, XPS studies of MoS2 formation from ammonium tetrathiomolybdate solutions, Surf. Coat. Technol., 1997, 91(3), 200–207 CrossRef CAS.
- J. Kibsgaard, T. F. Jaramillo and F. Besenbacher, Building an appropriate active-site motif into a hydrogen-evolution catalyst with thiomolybdate [Mo3S13]2− clusters, Nat. Chem., 2014, 6(3), 248–253 CrossRef CAS PubMed.
- M. S. Faber, R. Dziedzic, M. A. Lukowski, N. S. Kaiser, Q. Ding and S. Jin, High-performance electrocatalysis using metallic cobalt pyrite (CoS2) micro- and nanostructures, J. Am. Chem. Soc., 2014, 136(28), 10053–10061 CrossRef CAS PubMed.
- J. D. Benck, Z. Chen, L. Y. Kuritzky, A. J. Forman and T. F. Jaramillo, Amorphous molybdenum sulfide catalysts for electrochemical hydrogen production: Insights into the origin of their catalytic activity, ACS Catal., 2012, 2(9), 1916–1923 CrossRef CAS.
- Q. Liu, Q. Fang, W. Chu, Y. Wan, X. Li and W. Xu,
et al., Electron-doped 1T-MoS2 via Interface engineering for enhanced electrocatalytic hydrogen evolution, Chem. Mater., 2017, 29(11), 4738–4744 CrossRef CAS.
Footnote |
† Electronic supplementary information (ESI) available. See DOI: 10.1039/c7cy02001e |
|
This journal is © The Royal Society of Chemistry 2018 |