DOI:
10.1039/C7CS00479F
(Review Article)
Chem. Soc. Rev., 2018,
47, 1969-1995
Engineering functional inorganic–organic hybrid systems: advances in siRNA therapeutics
Received
28th June 2017
First published on 8th February 2018
Abstract
Cancer treatment still faces a lot of obstacles such as tumor heterogeneity, drug resistance and systemic toxicities. Beyond the traditional treatment modalities, exploitation of RNA interference (RNAi) as an emerging approach has immense potential for the treatment of various gene-caused diseases including cancer. The last decade has witnessed enormous research and achievements focused on RNAi biotechnology. However, delivery of small interference RNA (siRNA) remains a key challenge in the development of clinical RNAi therapeutics. Indeed, functional nanomaterials play an important role in siRNA delivery, which could overcome a wide range of sequential physiological and biological obstacles. Nanomaterial-formulated siRNA systems have potential applications in protection of siRNA from degradation, improving the accumulation in the target tissues, enhancing the siRNA therapy and reducing the side effects. In this review, we explore and summarize the role of functional inorganic–organic hybrid systems involved in the siRNA therapeutic advancements. Additionally, we gather the surface engineering strategies of hybrid systems to optimize for siRNA delivery. Major progress in the field of inorganic–organic hybrid platforms including metallic/non-metallic cores modified with organic shells or further fabrication as the vectors for siRNA delivery is discussed to give credit to the interdisciplinary cooperation between chemistry, pharmacy, biology and medicine.

Jianliang Shen
| Jianliang Shen received his BS in Applied Chemistry from Hunan University of Science and Technology in 2007, MS in Inorganic Chemistry from Xiangtan University in 2010, and PhD degree in Inorganic Chemistry under the supervision of Prof. Zong-Wan Mao from Sun Yat-Sen University in 2014, then started as a postdoctoral fellow under the supervision of Prof. Haifa Shen in Houston Methodist Research Institute. He was appointed as a full professor at Wenzhou Medical University and Wenzhou Institute of Biomaterials and Engineering in 2017. His research interests lie in novel hybrid nano/micro-particles for biomedical applications. |
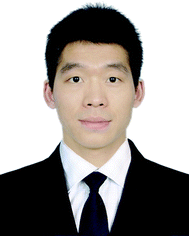
Wei Zhang
| Wei Zhang obtained his BS degree in Applied Chemistry from Sun Yat-Sen University in 2009, and PhD degree in Inorganic Chemistry under the supervision of Prof. Zong-Wan Mao from Sun Yat-Sen University in 2015. After completing a two-year postdoctoral training under the supervision of Prof. Xing-Jie Liang at the National Center for Nanoscience and Technology of China, he now works at the National University of Singapore as a research fellow. His research interests mainly focus on supramolecular self-assembly nanosystems and functional inorganic nanoparticles for biomedical applications. |
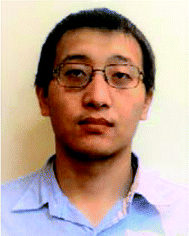
Ruogu Qi
| Ruogu Qi is currently a postdoctoral fellow at the Nanomedicine Department, Houston Methodist Research Institute. He received his BSc from Jilin University in 2007 and PhD from the Chinese Academy of Sciences in 2012. He then joined Baylor College of Medicine as a postdoc (2013–2014) and then moved to Massachusetts Institute of Technology as a postdoctoral Associate (2014–2016). His research interests are in engineering nanoparticles for drug/gene delivery, biosensors and bioimaging. |
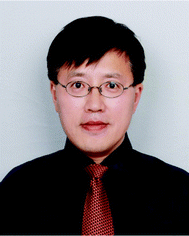
Zong-Wan Mao
| Zong-Wan Mao earned his Bachelor's degree at the Sichuan University in 1982, and received his MS and PhD degrees from Nanjing University in 1991 and 1994, respectively. Then he joined Prof. Liang-Nian Ji's group as a postdoctoral fellow at Sun Yat-Sen University. Two years later he received an Alexander von Humboldt Fellowship, and studied with Prof. Rudi van Eldik at the University of Erlangen-Nuremberg, Germany. He was appointed as an associate professor at Sun Yat-Sen University in 1999, and was promoted as a full professor in 2004. His research interests include metalloenzyme mimics and inhibitors, structure, recognition, and interaction of nucleic acids with small molecules, chemical biology of anticancer metal complexes, hybrid nanoparticles for probes, images and therapeutic applications. |
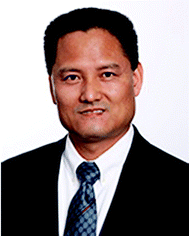
Haifa Shen
| Haifa Shen earned his MD from Zhejiang University Medical School of Hangzhou, China, in 1985, then received his PhD from the University of Texas at Houston in 1997. After completing a four-year postdoctoral fellowship at the National Cancer Institute, he worked on drug development at Lexicon Pharmaceuticals. He returned to the University of Texas at Houston Medical School as an assistant professor, then became an associate member of the Department of Nanomedicine in Houston Methodist Research Institute, and also became an associate professor of Weill Cornell Medical College of Cornell University. His research interests are in the fields of cancer therapeutics, drug delivery, nanomaterials and biomedicine. His research approach combines drug chemistry and formulation, nanotechnology, and cancer biology. |
1. Introduction
In spite of the huge advancement achieved in research, drug and technology development for cancer treatment during the past decades, cancer is still a major public health problem and is a main leading cause of death worldwide. Human cancer is a group of diseases characterized by the uncontrolled growth and spread of abnormal cells. Additionally, human cancers consist of a mixed population of malignant cells, which carry multiple genetic mutations caused by external and internal factors, such as tobacco smoke, infectious organisms, unhealthy diet, inherited genetic burden, hormones, and immune conditions.1 These factors may act together or in sequence to cause new cancer cases and deaths. As shown in Fig. 1A, around 1.6 million new cases of cancer have been diagnosed and about 35% of these have resulted in death in the US per year in the last five years. Additionally, more than 50% of cancer patients die annually according to statistics from 2000 to 2011 in China (Fig. 1B).2 In particular, there have been 4.292 million new cases and 2.814 million cancer deaths in the last year (Fig. 1C).3,4 Moreover, the number of new cases and deaths were 14.1 and 8.2 million in the world in 2012 (Fig. 1D).5 Thus, cancer is a major health care challenge. Currently, treatments include surgery, radiation, chemotherapy, hormone therapy, immune therapy, gene therapy and targeted therapy, each with their own limitations.6 However, many of the cancers are driver mutations causing tumor initiation, progression, metastasis and drug resistance that results in the urgent need for new technologies and strategies to fight cancer. Among the emerging technologies and anti-cancer therapeutics, small interfering RNA (siRNA) and RNA interference (RNAi) have been widely recognized to be capable of silencing many or perhaps all genes that result in modulation or targeted blockage of the biological processes, which are the defining hallmarks of cancer. Therefore, RNAi would pave the way for cancer treatment as a promising biotechnology.7,8
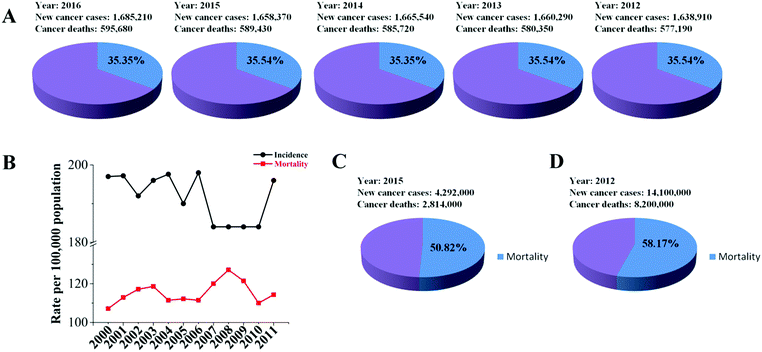 |
| Fig. 1 Estimated new cancer cases and deaths in the United States and China. (A) The new cases and deaths in the United States in recent five years. (B) Cancer death statistics in China from 2000 to 2011. (C) The new cases and cancer deaths in China in 2015. (D) The number of new cases and deaths in the world in 2012. | |
Since its discovery by Andrew Fire and Craig Mello, it was demonstrated in 1998 that long double-stranded RNA (dsRNA) mixtures were 10–100-fold more efficient at causing gene silencing than the single stranded RNAs in Caenorhabditis elegans (Fig. 2A).9 Four years later, Science named RNAi “technology of the year”. Fire and Mello were awarded jointly the Nobel Prize in Physiology or Medicine for their discovery of RNAi-gene silencing by siRNA in 2006 (Fig. 2A).10 Then, the therapeutics based on siRNA as a potent drug candidate for gene regulation came when Elbashir and co-workers proved that the synthetic siRNA enabled sequence-specific gene down-regulation in a mammalian cell line.11 Meanwhile, nanotechnology plays an important role in drug delivery including siRNA therapeutics, e.g. the first targeted nanoparticle-based siRNA therapeutics delivery in human cancer patients in 2010 (Fig. 2A).12–14 Indeed, a tremendous amount of effort has been put into development of siRNA cancer therapeutics over the last 20 years, and great progress has been achieved in research and development, both in academia and the pharmaceutical industry. However, during one period the big pharmaceutical factories closed the siRNA-related projects due to the disappointing results.15 Over the last 5 years, siRNA therapeutics has achieved a rapid development in fighting various diseases including cancer, which is indicated by over 30% of cases that have already achieved Phase 2 or Phase 3 clinical trials (Table 1). Moreover, the leading regional markets of RNAi are not only set up in developed countries, but also in developing countries (Fig. 2B). However, most of the leading companies of RNAi are in the USA or EU5 taking the statistics in the years ranging from 2015 to 2025 (Fig. 2C) (http://https://www.researchandmarkets.com/research/td7m2r/rnai). This trend indicates that RNAi would be a strong promising therapy for treatment of various human diseases in the future. Such big progress has led to a new avenue for siRNA therapeutics in clinical trials. Additionally, these achievements laid the foundations for employing RNAi as a key tool for next generation cancer drugs.
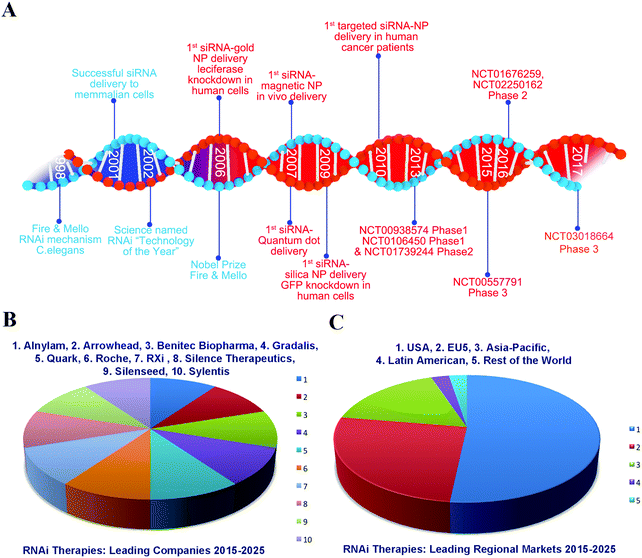 |
| Fig. 2 Summarization of the greatest events and markets in RNAi therapeutics. (A) Milestone timeline of the last 20 years in RNAi progress. (B) Leading companies of RNAi therapies in 2015–2025. (C) Leading regional markets of RNAi therapies in 2015–2025. | |
Table 1 Examples of RNAi-based therapeutics in clinical trials for treatment of various human diseases
Clinical subjects |
Disease |
Name |
Gene |
Delivery system |
http://ClinicalTrials.gov identifier |
Phase |
Ref. |
Cancer |
Advanced, recurrent cancer |
siRNA-EphA2-DOPC |
EphA2 |
LNP |
NCT01591356 |
Phase 1 |
16
|
Primary or secondary liver cancer |
TKM-080301 |
PLK1 |
LNP |
NCT01437007 |
Phase 1 |
17
|
Hepatocellular carcinoma |
DCR-MYC |
MYC |
LNP |
NCT02314052 |
Phase 1/2 |
18
|
Solid tumors, multiple myeloma, or lymphoma |
DCR-MYC |
MYC |
LNP |
NCT02110563 |
Phase 1 |
19
|
Solid tumor cancers |
CALAA-01 |
M2 subunit of ribonucleotide reductase (RRM2) |
LNP |
NCT00689065 |
Phase 1 |
20
|
Pancreatic ductal adenocarcinoma; pancreatic cancer |
siG12D LODER |
KRAS |
Polymer |
NCT01188785 |
Phase 1 |
21
|
Pancreatic ductal adenocarcinoma; pancreatic cancer |
siG12D-LODER |
KRAS |
Polymer |
NCT01676259 |
Phase 2 |
22
|
Advanced solid cancer |
Atu027 |
PKN3 |
LNP |
NCT00938574 |
Phase 1 |
23
|
Carcinoma, pancreatic ductal |
Atu027 |
Protein kinase N3 (PKN3) |
LNP |
NCT01808638 |
Phase 1/2 |
24
|
|
Ocular and retinal disorders |
Subfoveal choroidal neovascularization (CNV) secondary to age-related macular degeneration (AMD) |
AGN-745 (siRNA-027) |
VEGFR-1 |
Naked siRNA |
NCT00363714 |
Phase 1/2 |
24
|
Choroid neovascularization; age-related macular degeneration |
AGN211745 (siRNA-027) |
VEGFR-1 |
Naked siRNA |
NCT00395057 |
Phase 2 |
25
|
Optic nerve atrophy (stratum I) and acute non-arteritic anterior ischemic optic neuropathy (NAION) (stratum II) |
QPI-1007 |
Caspase 2 |
Naked siRNA |
NCT01064505 |
Phase 1 |
22
|
Moderate to severe dry eye disease |
SYL1001 |
Transient receptor potential cation channel subfamily V member 1 (TRPV1) |
Naked siRNA |
NCT03108664 |
Phase 3 |
26
|
Ocular pain; dry eye |
SYL1001 |
Transient receptor potential Vanilloid-1 (TRPV1) |
Naked siRNA |
NCT01438281 |
Phase 1 |
27
|
Ocular pain; dry eye syndrome |
SYL1001 |
Transient receptor potential Vanilloid-1 (TRPV1) |
Naked siRNA |
NCT01776658 |
Phase 1/2 |
22
|
Diabetic macular edema |
Bevasiranib (Cand5) |
VEGF |
Naked siRNA |
NCT00306904 |
Phase 2 |
28
|
Macular degeneration |
Bevasiranib (Cand5) |
VEGF |
Naked siRNA |
NCT00259753 |
Phase 2 |
22
|
Age related macular degeneration |
Bevasiranib |
VEGF |
Naked siRNA |
NCT00557791 |
Phase 3 |
29
|
Choroidal neovascularization; diabetic retinopathy; diabetic macular edema |
PF-04523655 |
RTP801 |
Chemically modified |
NCT01445899 |
Phase 2 |
30
|
Glaucoma; ocular hypertension |
SYL040012 |
β-Adrenergic receptor 2 |
Naked siRNA |
NCT01227291 |
Phase 1/2 |
31
|
Open angle glaucoma; ocular hypertension |
SYL040012 |
β-Adrenergic receptor 2 |
Naked siRNA |
NCT02250612 |
Phase 2 |
32
|
Ocular hypertension; open angle glaucoma |
SYL040012 |
β-Adrenergic receptor 2 |
Naked siRNA |
NCT01739244 |
Phase 2 |
33
|
Homozygous familial hypercholesterolemia |
ALN-PCSSC |
PCSK9 |
LNP |
NCT02963311 |
Phase 2 |
34
|
Atherosclerotic cardiovascular disease; familial hypercholesterolemia; diabetes |
ALN-PCSSC |
PCSK9 |
LNP |
NCT02597127 |
Phase 2 |
34
|
Hypercholesterolemia |
PRO-040201 |
Apolipoprotein B |
LNP |
NCT00927459 |
Phase 1 |
22
|
|
Urinary system disease |
Delayed graft function in kidney transplantation |
QPI-1002 |
Pro-apoptotic gene p53 |
Naked siRNA |
NCT00802347 |
Phase 1/2 |
22
|
Primary hyperoxaluria type 1 (PH1) |
ALN-GO1 |
Glycolate oxidase (GO) |
Naked siRNA |
NCT02706886 |
Phase 1/2 |
32
|
Primary hyperoxaluria type 1 |
DCR-PH1 |
HAO1 |
LNP |
NCT02795325 |
Phase 1 |
NCT02795325 |
|
Others |
Hypertrophic scar |
STP705 |
TGF-β1 and Cox-2 |
Polypeptide nanoparticle (PNP) |
NCT02956317 |
Phase 1/2 |
NCT02956317 |
Pachyonychia congenita |
TD101 |
K6a |
Naked siRNA |
NCT00716014 |
Phase 1 |
22
|
Moderate to extensive fibrosis (METAVIR F3-4) |
ND-L02-s0201 |
HSP47 |
LNP |
NCT02227459 |
Phase 1 |
18
|
Normal healthy subjects |
ND-L02-s0201 |
HSP47 |
LNP |
NCT01858935 |
Phase 1 |
18
|
2. Biochemical properties of siRNA and RNAi
siRNAs are typical short/small double-stranded RNA molecules, approximately 21 nucleotides in length, and having about 19 bp in their core region (Fig. 3A) with the ability to mobilize the RNAi pathway.35 The interference effect has revealed immense potential for regulating the diseases caused by the overexpression or mutation of genes including cancer, genetic disorder and other diseases because of sequence-specific gene silencing. The structure is also well defined; siRNAs have phosphorylated 5′ ends and hydroxylated 3′ ends with two overhanging nucleotides. Additionally, naked siRNA has a large molecular weight (14 kDa) and negative charges (polyanionic nature of the phosphate charges). Once the long siRNA or siRNA is introduced inside the cytoplasm, the enzyme endoribonuclease Dicer would cleave the long siRNA into fragment siRNA (21 bp), then siRNA is incorporated into a RNA inducing silencing complex (RISC), which consists of the Argonaute (Ago) protein as one of its main components, that results in splitting of each double-stranded siRNA into the passenger (sense) strand and the guide (antisense) strand. The sense strand is degraded, whereas the antisense strand is incorporated into RISC as activated RISC complexes form that further direct the specificity of the target mRNA recognition via complementary base pairing. Later, Ago-2 catalyzes cleavage of the target mRNA thereby triggering protein down-regulation and gene silencing (Fig. 3B).35 Compared to anticancer chemotherapeutic drugs, siRNA possesses a number of advantages due to its specific mechanism. The benefits of siRNA as a potential anticancer drug are strongly supported by its effective inhibition of the target mRNA or mutated gene of interest in any type of cancer cells. Moreover, the synthesis of siRNA is relatively easy with low production cost.
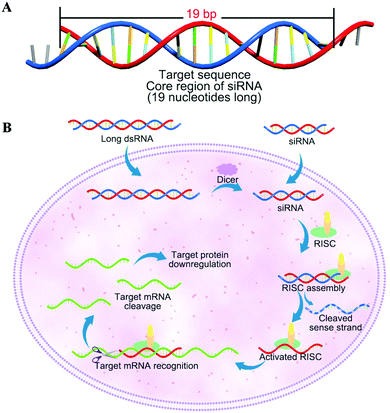 |
| Fig. 3 Structure and mechanism of siRNA. (A) Structure of siRNA. (B) Action of RNAi. | |
3. Bio-barriers of siRNA therapeutics
In spite of the advancement in preclinical and clinical studies, no product has been launched into the market, because there are still many challenges to be overcome for siRNA clinical therapeutics. As well known, siRNA molecules have unfavorable physicochemical properties, such as negative charges, large molecular weight and size, and instability that impede the access through the cell membrane. Serum endonuclease easily degrades naked siRNA which is then easily eliminated through glomerular filtration. This implies that the double-stranded siRNA molecules have a very short half-life (10 min) and very low stability. This issue can be overcome by chemical modifications of the RNA backbone and by embedding the siRNA into nano-carriers.35 In order to exhibit its effect, siRNA needs to arrive in the cytoplasm of the cancer cell. From the region of injection (if systemic delivery is used) to the site of action, the siRNA molecule faces many biological obstacles. Challenges that need to be overcome are safety, stability and effective delivery.
From the blood circulation to the tumor cell, the RNAi and/or its transport mechanism needs to additionally overcome biobarriers. Biobarriers are biological surfaces (epithelia, endothelia, cellular, nuclear, endosomal membranes, etc.), which can be multi-cellular and/or multi-compartmental. These are elements of separation within defined compartments (e.g. vascular, cytoplasmic, stromal, etc.) and between different compartments. In the delivery of nanoparticles to tumor sites, biobarriers comprise all “barriers” that a nanoparticle needs to cross in order to reach the target cell. Using systemic administration (e.g. intravenous injection of nanoparticles), the biobarriers that need to be addressed are: (1) enzymatic degradation; (2) reticulo-endothelial (RE) organ sequestration and phagocytes; (3) tumor interstitium; (4) crossing the cellular membrane; (5) endosomal escape in order to prevent degradation in lysosomes and/or excretion from the cell (Fig. 4).36 An adequate negotiation of these barriers is therefore necessary in order to successfully deliver the siRNA into the cell obtaining a sufficient therapeutic index.
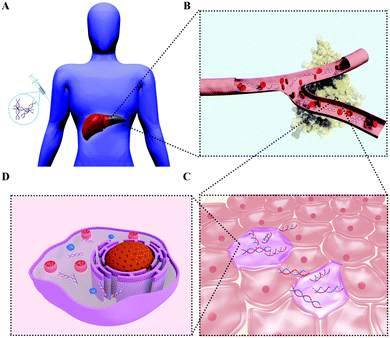 |
| Fig. 4 Physiological and biological obstacles of siRNA therapeutics. (A) Injection of siRNA therapeutics. (B) Circulation of siRNA in blood vessels. (C) Extracellular behavior of siRNA therapeutics. (D) Intracellular action of siRNA therapeutics. | |
So far, the major barriers that prevent siRNA therapeutics from reaching the market are poor resistance to enzymes, such as RNase, short biological half-life, lack of cell/tissue targeting, ineffective cellular internalization, complicated escape from endosomes/lysosomes, toxicity and other related side effects and difficulties (Fig. 4).37 Therefore, the successful delivery of siRNA to the targeted cell/organs is the most important issue for treatment of cancer in clinical application.
Therefore, to address these issues, a special system for siRNA delivery is needed. Delivery systems used nowadays can be categorized into physical methods, conjugation methods, methods with natural carriers, such as viruses and bacteria, and non-viral carrier methods.38 The use of viral vectors for the delivery of siRNA tends to be avoided because of insertional mutagenesis and immunogenicity problems that may arise due to the nature of the carrier. A new, non-viral way to overcome biobarriers, with added capability with respect to traditional cancer treatment methods of multi-functionality and specific cell targeting, has been proposed by nanomedicine research – nanoparticles.
Notably, the rational design of siRNA carriers is an important consideration because the use of high quantities of carriers can result in toxicity as a consequence of poor metabolism and elimination of the carriers. A great number of systems have been reported for siRNA delivery based on biomaterial platforms, such as lipids, polymers, and nanoparticles.39–41 Here we put the concept of inorganic–organic hybrid nanoparticle-based siRNA therapeutics. Additionally, we review the engineering strategies of inorganic–organic hybrid systems and examine the details of metallic and non-metallic core hybrids with organic moieties for siRNA delivery. Finally, we summarize the advantages of such hybrid systems for paving the way for next generation siRNA therapy.
4. Surface engineering strategies of inorganic–organic hybrid nanoparticles
The application of nanomaterials in biomedical areas shows great promise and inorganic nanomaterials attract particular interest in bioimaging, diagnosis, drug delivery and therapy applications. As regards to the above-mentioned siRNA delivery, inorganic nanomaterials also exhibit great potential to reveal the mechanism of the siRNA transportation process and subsequently to construct well-designed vectors to circumvent the biological barriers of siRNA delivery. To endow inorganic nanostructures with siRNA transportation capacity, surface engineering with organic ligands should firstly be carried out. Many strategies have been developed for the surface engineering of inorganic nanomaterials, and they can be divided into the following categories: covalent ligand conjugation, amphiphilic polymer assembly, electrostatic layer-by-layer assembly, in situ ligand coating during synthesis, host–guest supramolecular ligand self-assembly, and lipid shell coating (Fig. 5).
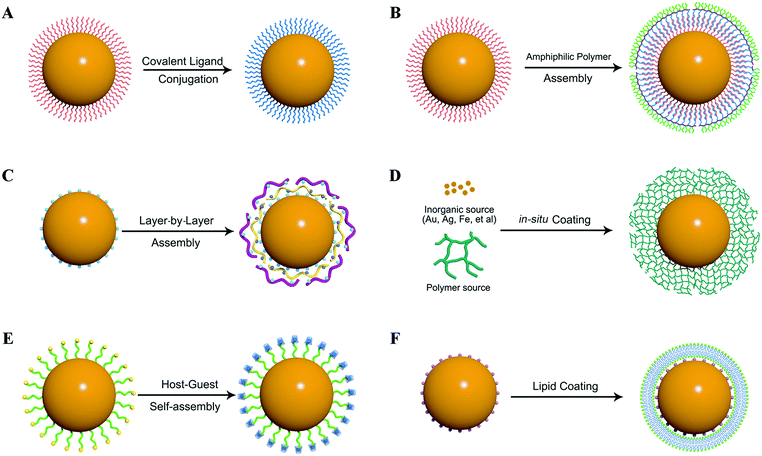 |
| Fig. 5 The main strategies for inorganic nanoparticle surface engineering. (A) Covalent ligand conjugation. (B) Amphiphilic polymer assembly. (C) Electrostatic layer-by-layer assembly. (D) In situ ligand coating during synthesis. (E) Host–guest supramolecular ligand self-assembly. (F) Lipid shell coating. | |
4.1. Covalent ligand conjugation
The surface chemistry of different kinds of inorganic nanoparticles has been extensively explored for surface engineering via covalent ligand conjugation. Several kinds of anchoring groups have been developed for ligand conjugation, such as thiol, carboxyl, and phosphate terminal ligands (Table 2). Thiols have been widely used as an anchoring unit to fabricate inorganic nanoparticles, mainly metallic nanoparticles. Thiols have very high affinity towards many metal ions, such as Au, Ag, Cu, Zn, etc. via coordination interactions. Thereby, thiol-containing cationic ligands can be used for binding to the nanoparticle surface to generate hybrid nanoparticles for siRNA delivery. Alternatively, nanoparticles can bond with a heterobifunctional ligand containing thiol and another functional group, and can then be further modified with other positively charged ligands. Rotello et al. synthesized three thiol-containing dendron ligands with triethylenetetramine (TETA) terminal groups of different generations.42 These ligands were used to modify gold nanoparticles (AuNPs) through Au–SH coordinate binding. The as-prepared hybrid nanoparticles were cationic and resisted aggregation, which were shown to be effective nanocarriers for siRNA delivery. Similar to thiol ligands, disulfide terminal group containing ligands can also bind with nanoparticles through S–metal bonds. In addition to AuNPs, a lot of other inorganic nanoparticles can be modified with thiol or disulfide ligands, such as silver nanoparticles (AgNPs), quantum dots (QDs), CuS nanoparticles, and so on. Hydrophobic iron oxide nanoparticles (IONPs) could also be functionalized through a covalent ligand exchange strategy. Xu et al. reported that the bidentate ligand, dopamine, can coordinate to the surface of IONPs owing to the improved orbital overlap of the five-membered ring and a reduced steric effect on the iron complex.43 The resulting IONPs showed excellent stability under physiological conditions. For non-metal inorganic nanomaterials, such as silica nanoparticles and carbon nanomaterials, they usually have abundant functional groups on the surface, which can be easily conjugated with other ligands.
Table 2 Surface engineering of representative inorganic nanoparticles through the covalent ligand conjugation strategy
Nanoparticle |
Anchoring groups |
Ligands |
Ref. |
Au nanoparticles (AuNPs) |
Thiol or disulfide |
α-Acetal-ω-mercapto-poly(ethylene glycol), cysteine-terminated nuclear targeting peptides, thiol-containing dendron ligands, poly(N-isopropylacrylamide), thioctic acid-modified DNA oligonucleotide |
42 and 44–47
|
Quantum dots (QDs) |
Thiol or disulfide |
Thioctic acid, dithiocarbamate ligands, dihydrolipoic acid-zwitterionic ligands |
48–50
|
Carboxyl |
Carboxymethyl chitosan, bicarboxyl dendron ligand, L-arginine-modified β-cyclodextrin |
51–53
|
Imidazole |
Multidentate-imidazole polymer ligands |
54
|
Phosphine |
Oligomeric phosphine ligand |
55
|
Iron oxide nanoparticles (IONPs) |
Carboxyl |
2,3-Dimercaptosuccinic acid, carboxymethylated polyvinyl alcohol |
56 and 57
|
Dopamine |
Dopamine-nitrilotriacetic acid ligand, dopamine-poly(ethylene glycol), dopamine-biotin |
43, 58 and 59
|
Phosphonate |
Poly(ethylene glycol)-phosphonate |
60
|
Upconversion nanoparticles (UCNPs) |
Carboxyl |
Polyacrylic acid, poly(ethylene glycol)-diacid |
61 and 62
|
Phosphonate |
Poly(ethylene glycol)-phosphonate |
63
|
Amino |
Poly(amidoamine), polyethylenimine |
64 and 65
|
Silicon/silica nanoparticle |
Various functional groups derived from silane ligands (carboxyl, amino, aldehyde, thiol, isocyanato, etc.) |
Poly(ethylene glycol), polyethylenimine, cyclodextrin, nuclear targeting peptide, etc. |
66–70
|
Carbon nanostructure |
Surface carboxyl and hydroxyl groups |
Poly(ethylene glycol), polyethylenimine, chloroacetic acid, hyaluronic acid, chitosan, etc. |
71–73
|
4.2. Amphiphilic polymer assembly
Inorganic nanoparticles with high quality are usually prepared in the organic phase with hydrophobic surface protection ligands because it allows a higher reaction temperature. In addition to the above-mentioned ligand exchange strategy, amphiphilic polymer coating is another widely used method for hydrophobic nanoparticle surface engineering. The polymers encapsulate nanoparticles via hydrophobic interactions between the hydrophobic portion of the polymer chains and the initial hydrophobic surface coating of nanoparticles. The hydrophilic portion of the polymer chains faces the aqueous media, and renders the nanoparticles soluble in aqueous media. This approach keeps the initial surface coating of nanoparticles and can be more effective in preserving the physicochemical properties of as-synthesized nanoparticles. The representative amphiphilic polymers used for hydrophobic inorganic nanoparticle surface engineering are summarized in Table 3. Nie et al. used a high-molecular-weight (100 kDa) copolymer with an elaborate ABC triblock structure and a grafted 8-carbon (C-8) alkyl side chain to encapsulate the hydrophobic ligand, tri-n-octylphosphine oxide (TOPO) protected CdSe/ZnS QDs.74 A key finding is that this polymer can disperse and encapsulate single TOPO-capped QDs via a spontaneous self-assembly process. As a result, after linking to PEG molecules, the polymer-coated QDs are perfectly protected, so their optical properties did not change in a broad range of pH (1 to 14) and salt conditions (0.01 to 1 M). Dai et al. reported the synthesis of several poly(ethylene glycol) grafted branched polymers based on poly(γ-glutamic acid) and poly(maleic anhydride-alt-1-octadecene) for functionalization of various nanomaterials including single-walled carbon nanotubes (SWNTs), gold nanoparticles, and gold nanorods, affording high aqueous solubility and stability for these materials.75 Moreover, the polymer-coated SWNTs exhibited remarkably long blood circulation upon intravenous injection into mice.
Table 3 Representative amphiphilic polymers used for hydrophobic inorganic nanoparticle surface engineering by the non-covalent assembly method
Hydrophilic backbone |
Hydrophobic chain |
Nanoparticles |
Ref. |
Poly(acrylic acid) or PEGylated poly(acrylic acid) |
Polybutylacrylate, polyethylacrylate, alkylamine (octyl, dodecyl, hexadecyl, octadecyl) |
QDs, UCNPs |
74, 76 and 77
|
PEGylated poly(maleic anhydride) |
1-Octadecene, 1-decene, styrene |
QDs, IONPs, carbon nanotube, reduced graphene oxide |
75 and 78–80
|
PEGylated poly(γ-glutamic acid) |
Pyrene, phospholipid 1,2-distearoyl-sn-glycero-3-phosphoethanolamine |
Carbon nanotube |
75
|
4.3. Electrostatic layer-by-layer assembly
Electrostatic layer-by-layer assembly is another easy to handle strategy for inorganic nanoparticle surface engineering because of the strong electrostatic attraction between oppositely charged species. Li et al. have developed a layer-by-layer (LBL) assembly strategy that uses oppositely charged linear polyions to generate water-soluble UCNPs.81 Upon sequential adsorption of positively charged poly(allylamine hydrochloride) (PAH) and negatively charged poly(sodium 4-styrenesulfonate) (PSS) onto the surface of nanoparticles, they successfully modified NaYF4:Yb/Er nanoparticles with stable amino-rich shells. The LBL assembly technique offers many advantages, albeit requiring repeated wash steps after each adsorption step, and these include simplicity, universality, and thickness control on the nanoscale. More importantly, the high stability and biocompatibility of these polyions make them attractive as coating materials for a wide range of fundamental and technological applications.
4.4.
In situ ligand coating during synthesis
Taking advantage of the easy-preparation nature of some kinds of inorganic nanoparticles, such as AuNPs, AgNPs, IONPs, the organic–inorganic hybrid nanoparticles could also be prepared in a one-pot reaction containing the inorganic precursors and organic ligands. He et al. reported the synthesis of hyperbranched polyethyleneimine-protected silver nanoclusters (hPEI–AgNCs) using a facile, one-pot reaction under mild conditions.82 The hPEI–AgNCs were very stable against extreme pH, ionic strength, temperature, and photoillumination. IONPs also have been reported to be prepared in the presence of a variety of surface coating ligands, such as dextran, starch, glycosaminoglycan, and polyvinyl alcohol (PVA), to obtain biocompatible and thermodynamically stable dispersions of IONPs in aqueous media. Pierre et al. reported the detailed magnetic and structural properties of IONPs formed in the presence of dextran. The results suggested that the presence of dextran limits the particle size compared to particles prepared without the polymer.83
4.5. Host–guest supramolecular ligand self-assembly
Host–guest interaction mediated self-assembly of hydrophilic ligands on the surface of hydrophobic nanoparticles can also be used as an efficient method to make the hydrophobic nanoparticles soluble in water. Li et al. successfully developed a simple and efficient approach to draw adamantane (Ad)-coated NaYF4:Yb,Er nanoparticles into water by the interaction between the host molecule β-cyclodextrin (β-CD) and the guest Ad molecules.84 This method provided a simple post-treatment through only stirring or shaking, rapid response time (<20 s), high conversion yield (>95%) and exhibited good capability for bioimaging. High quality inorganic nanoparticles are usually synthesized in the organic phase with hydrophobic surface coating ligands, such as oleylamine, oleic acid, etc. To transform the hydrophobic ligand coated nanoparticles into water-soluble forms, a host–guest strategy based on the interaction of α-cyclodextrin (α-CD) and the hydrophobic carbon chain was developed. Hydrophobic IONPs, AgNPs and UCNPs were all reported to be transferred to aqueous solution through hydrophilic α-CD self-assembly on the nanoparticle surface to afford aqueous suspensions of nanoparticles with very good stability.85,86 This approach is considered to be a generic method for the stabilization of hydrophobic ligand capped inorganic nanoparticles in aqueous solutions, which is an important aspect for the exploration of biological applications of inorganic nanoparticles.
4.6. Lipid shell coating
Lipids are widely used as delivery vectors for small molecular drugs and nucleic acid gene drugs of various molecular weights. Lipids are considered to be highly biocompatible and non-immunogenic. Lipid shell coating is a very well-established method for inorganic nanoparticle surface engineering to produce hybrid nanosystems with high biocompatibility. Huang et al. reported a lipid-coated calcium phosphate (LCP) nanoparticle formulation for efficient delivery of siRNA to a xenograft tumor model by intravenous administration.87 After entering the cells, LCP would decompose at low pH in the endosome, which would cause endosome swelling and bursting to release the entrapped siRNA. Inorganic nanoparticles with hydrophobic surface ligands (such as QDs, IONPs) can also be hydrophilized by coating with lipid shells for biomedical applications.88,89 Anderson et al. developed a simple and efficient method to coat IONPs with lipid molecules with low polydispersity.89 Cationic lipid-coated hybrid IONPs showed potent capability to deliver DNA and siRNA into cells. Moreover, the application of an external magnetic field further enhanced the efficiency of nucleic acid delivery.
5. Metallic core hybrids with organic shell delivery systems
There has been a long history of metals used in disease treatments since ancient times. Copper and iron were recorded for reducing inflammation and treating anemia, respectively, in ancient compendiums.90 More modern written accounts published at the beginning of the 20th century reported the use of sodium vanadate to lower blood sugar levels in diabetic patients and a simple gold complex to treat rheumatoid arthritis.91 The greatly successful development of platinum-based anticancer drugs attracted tremendous attention for medicinal inorganic chemistry.90 Along with the advent of the nanotechnology era, many kinds of metal-based nanoparticles have been prepared and great efforts have been made to explore their biomedical applications. These metal-based nanoparticles, including gold nanoparticles (AuNPs), semiconductor quantum dots (QDs), iron oxide nanoparticles (IONPs), up-conversion nanoparticles (UCNPs), and so on, have been extensively developed for detection, imaging, delivery and therapy applications. Owing to their unique optical, magnetic, electronic and thermal properties, versatile functions can be endowed to the engineered delivery systems. For example, the real-time delivery process can be tracked by optical imaging or magnetic resonance imaging (MRI), smart cargo release can be realized through pH or thermal responsive design, and much more potent therapy efficiency can be achieved by combining the intrinsic therapeutic effects of the metal nanoparticles. Moreover, the composition, size, shape and surface properties of these nanoparticles can be flexibly and precisely tailored, which afford great superiority for different biomedical applications.92 In this section, we will introduce representative classes of metal-based nanoparticles engineered as inorganic–organic hybrid delivery systems of siRNA therapeutics.
5.1. Gold nanoparticles
Gold is known to be one of the least reactive metals, exhibiting incredible chemical resistance against both oxidation and corrosion. The biocompatibility of Au nanomaterials has been widely demonstrated by a number of in vitro and in vivo studies.93–95 Meanwhile, Au nanostructures can be readily synthesized with great diversity in size and shape, which is considered to be a crucial factor to determine the fate of nanocarriers, such as the blood circulation time, organ specific accumulation and cellular uptake mechanism. The size of synthetic AuNPs usually ranges from several nanometers to several hundred nanometers. Most commonly investigated morphologies include gold nanospheres, gold nanorods, gold nanoshells, gold nanocages, gold nanoclusters, and so on (Fig. 6A). Moreover, gold nanostructures possess some other unique properties for biomedical applications, such as strong localized surface plasmon resonance (LSPR), X-radiation absorption ability, photo-thermal conversion properties, and so on.96 Therefore, when the nanocarrier strategy emerged as a promising method to address the barriers of naked siRNA administration, gold nanoparticles immediately caught the attention of researchers as an excellent candidate to achieve this goal.97,98 In the past decade, various kinds of AuNP-based delivery systems have been developed through well-defined organic surface engineering with biocompatible polymers or biomolecules for siRNA delivery.
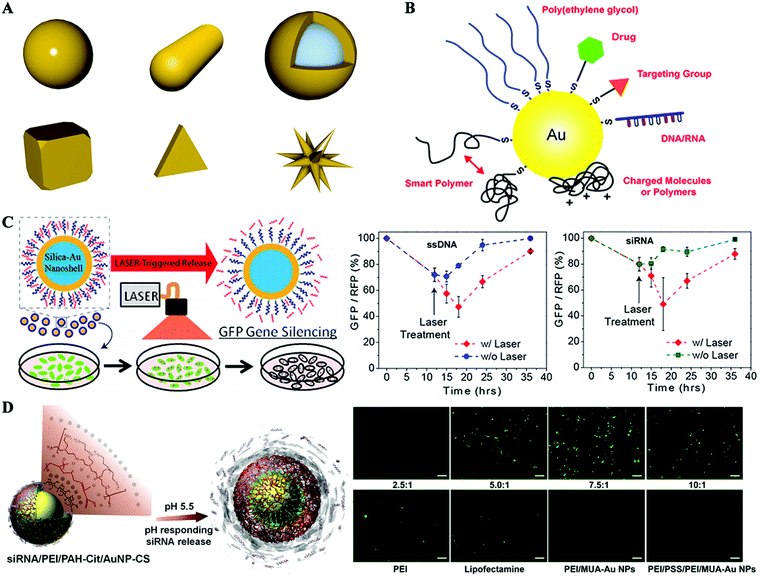 |
| Fig. 6 Hybrid gold nanoformulated siRNA therapeutics. (A) Illustration of gold nanostructures of different morphologies. (B) Gold nanoparticle surface engineering with a wide variety of functional moieties. (C) Gold nanoshells for siRNA delivery and laser-triggered release. (D) Gold nanoparticles coated with charged reversible polymers via the layer-by-layer method for siRNA delivery and pH-responsive release. Figures reproduced with permission from (B) ref. 93, (C) ref. 102, and (D) ref. 106 and 107. Copyright (B) 2011 the Royal Society of Chemistry, (C and D) 2009, 2010 and 2012 American Chemical Society. | |
As introduced above, a well-characterized approach for surface engineering of gold nanostructures utilizes their ability to form very strong and stable gold–thiolate bonds (Au–S) with molecules containing thiol (–SH) or disulfide groups (S–S) (Fig. 6B).93 Park et al.99 synthesized AuNPs in the presence of cysteamine hydrochloride to afford amine-functionalized AuNPs with a positively charged surface. Then siRNA conjugated with PEG5000 with a disulfide linker was added to adhere to the surface of AuNPs through electrostatic interactions. The nanosized polyelectrolyte complexes could be efficiently internalized into human prostate carcinoma cells and release siRNA in a reductive cytosol environment. Thus, enhanced intracellular uptake of siRNA and significant inhibition of the target gene expression were achieved. Anderson et al.100 employed a different strategy for siRNA–PEG co-loading with AuNPs by first decorating 15 nm AuNPs with SH-PEG1000-NH2 and then conjugating siRNA by means of a disulfide crosslinker to the terminal of the PEG. A comparable loading of ∼30 strands of siRNA per nanoparticle has been reported. To enhance the cellular uptake and facilitate endosomal escape, these particles were further coated with different kinds of poly(β-amino ester)s (PBAEs), a new class of cationic biodegradable polymers. These nanoparticulate formulations finally realized high levels of in vitro siRNA delivery, achieving gene silencing as good as or better than the commercially available lipid reagent, Lipofectamine 2000. Other authors have taken another way to address the endosomal escape issue and realize laser-triggered controlled release of siRNA by introducing gold nanoshells as the core structure of the delivery system. Gold nanoshells with peak plasmon resonance in the near-infrared (NIR) range are susceptible to local heating under irradiation of NIR light (Fig. 6C).101,102 A poly-L-lysine peptide (cysteine–tyrosine–serine–lysine50) was used to modify the gold nanoshell surface, affording a positive charge of 14.27 mV to capture siRNA electrostatically. Controlled release of siRNA and enhanced endosome rupture have been achieved by irradiating with lower power NIR irradiation without causing obvious cytotoxicity.102
Gold nanoparticles have also been developed for co-delivery of siRNA and small molecular chemotherapeutic drugs through well-defined surface organic ligand engineering.103,104 Gong et al. reported the surface engineering of AuNRs for co-delivery of achaete-scute complex-like 1 (ASCL1) siRNA and doxorubicin (Dox).103 AuNRs were covalently modified with different functional components: a pH-labile hydrazone linkage tethering Dox to enable pH-controlled drug release, a polyarginine cationic polymer for complexing siRNA and a tumor-targeting ligand octreotide (OCT), to specifically target neuroendocrine cancer cells. Finally, the delivery system Au–DOX–OCT–siRNA resulted in significantly higher cellular uptake and gene silencing effect. OCT targeting nanocarrier-mediated combination chemotherapy and RNA silencing exhibited the strongest anti-proliferative effect.
In addition to covalent modification of gold nanostructures with thiol-containing ligands, layer-by-layer assembly is an alternative way to engineer multifunctional surface for siRNA delivery.105–111 The commonly used AuNPs are synthesized in aqueous solution by a citrate reduction method, resulting in a negatively charged surface. Poly(ethylene imine) (PEI), a widely used positively charged polymer in gene delivery studies, has been employed to conduct the layer-by-layer assembling process.105 In this work, two kinds of final delivery systems with different terminal surfaces were fabricated, one with a terminal siRNA layer while another one with a terminal PEI layer. The final size of these particles is reported to be within the 20–25 nm range, with a loading density of about 780 siRNA per particle. The cellular particle uptake of siRNA/PEI–AuNPs was significantly more than that of PEI/siRNA/PEI–AuNPs; however, transmission electron microscopy (TEM) images showed siRNA/PEI–AuNPs primarily trapped within the endosome. Finally, GFP silencing studies showed that PEI/siRNA/PEI–AuNPs achieved 70% GFP knockdown 48 h post-transfection while no knockdown was observed for particles with siRNA/PEI–AuNPs, suggesting the need for an endosome escape component of siRNA delivery vectors. Liang et al. reported the incorporation of an anionic charge-reversal polyelectrolyte (PAH-Cit, cis-aconitic anhydride-functionalized poly(allylamine)) to assemble with PEI and siRNA on AuNP surfaces through a layer-by-layer method (Fig. 6D).106,107 The PAH-Cit can undergo a charge reversal from negative to positive inside the lysosome during a pH change from 7.4 to 5.0, which will disassemble the PEI/siRNA/PAH-Cit/PEI–AuNP layer-by-layer complex to accelerate siRNA release. The silencing efficiency of targeted gene was reported to be 80%, compared to only 20% for complexes formed with noncharge-reversal polymers. Confocal images revealed that the enhanced gene silencing effect benefited from the increased endosomal escape ability of this charge-reversal complex. This layer-by-layer strategy was also used to fabricate PEI modified gold nanorods (AuNRs) for siRNA delivery in combination with photothermal therapy.109–111 Cancer is a complicated disease that usually requires combination therapy of several treatment modalities. Photothermal therapy is an effective and noninvasive cancer treatment procedure, which generally utilizes a NIR light source to activate tumor localized photothermal agents to trigger local hyperthermia. AuNRs exhibit a strong photo-thermal conversion effect under NIR light irradiation because of the surface plasmon field enhancement of the absorption. We demonstrated that PEI–Au NRs not only protect siRNA from degradation, but also facilitate endosomal escape, both of which are prerequisites for successful gene silencing.109 As a result, the combined anticancer activity of PEI–Au NR/siRNA, PKM2 gene inhibition and photothermal effect showed very potent anticancer efficiency against breast cancer cells.
Moreover, taking advantage of their ease of synthesis, surface engineering of gold nanoparticles with positive organic ligands for siRNA delivery could be readily realized in situ during the process of particle synthesis.112–114 Wang et al. reported the manufacturing of PEI-capped AuNPs by directly using PEI25k as the reductant and stabilizer, in place of citrate.112 This resulted in AuNPs with a positive surface charge which loaded siRNA molecules via electrostatic interactions. It was reported that PEI–AuNPs induce more significant and enhanced reduction in targeted green fluorescent protein expression in MDA-MB-435s cells, because of more siRNA internalization, as evidenced by confocal laser scanning microscopy observation and fluorescence-activated cell sorting analyses. Furthermore, lower cytotoxicity of PEI–AuNPs than pure PEI was observed at a siRNA concentration of 120 nM. Another study adopted a similar in situ strategy and synthesized AuNPs in the presence of a block polymer p(HPMA70-b-DMAPMA24).113 This resulted in AuNPs with diameters of about 7 nm surrounded by a HPMA block, which serves as a hydrophilic, sterically stabilizing shell (similar to the role of PEG). The DMAPMA block functioned as the cationic part for binding siRNA. The gene silencing efficiency on luciferase-expressing KB cells was evaluated to up to 50% under the condition of 100 nM siRNA, 6 h transfection and 24 h incubation. In recent years, fluorescent gold nanoclusters have attracted a lot of attention due to their unique features, such as ultrasmall sub-nanometer size, great biocompatibility and excellent photostability, making them ideal fluorescent labels for biological applications.115,116 In a recent study, positively charged gold nanoclusters (GNC) were synthesized through one-step reduction of Au3+ in the presence of thiolate-containing GSH and oligoarginine CRRRRRRRRR.114 The prepared GNCs had a well-defined core structure with diameters of around 2.6
nm. It was reported that the GNCs had a loading capacity of 226
μmol siRNA per gram GNCs. Nerve growth factor (NGF) siRNA was loaded for pancreatic cancer treatment. The GNC–siRNA complex exhibited several attractive properties, increasing the serum stability of siRNAs, prolonging the blood circulation lifetime of siRNA and enhancing the cellular uptake and tumor accumulation of siRNA. Subsequently, GNC–siRNA complexes effectively down-regulated the NGF expression in both Panc-1 cells and the pancreatic tumor model. Finally, effective inhibition of the tumor progression was achieved in three pancreatic tumor models (subcutaneous model, orthotopic model and patient-derived xenograft model) without adverse effects.
5.2. Quantum dots
In the past two decades, quantum dots (QDs), also known as semiconductor nanoparticles, have become one of the dominant classes of fluorescent dyes in various biomedical areas since the pioneering works in 1998.117–121 QDs are generally made from hundreds to thousands of atoms of group II and VI elements (e.g. CdSe and CdTe) or group III and V elements (e.g. InP and InAs) with diameters on the order of 2–10 nm.122 QDs exhibit unique optical and electronic properties, such as size-tunable emission, resistance to photobleaching, superior signal brightness, and broad absorption spectra of excitation (Fig. 7A).121 QDs also have versatile surface chemistry, thereby allowing further surface engineering of functional groups to facilitate drug loading and cellular uptake. Therefore, QDs have been considered to be promising nanoscale scaffolds for designing multifunctional nanosystems with both imaging and therapeutic functions. Though the direct use of QDs as drug delivery vectors remains questionable due to their potential long-term toxicity, QDs offer great potential as a proof-of-concept model to investigate the fate of nanoparticle-based drug delivery systems (NDDS) in biological systems, especially in vivo.121,123 Owing to these unique features of QDs, they have also been considered to be a promising candidate for siRNA delivery systems to evaluate the cellular uptake mechanism,124 siRNA release process tracking,125–128 cancer cell targeting,129,130 endosome escape,131in vivo distribution,132,133 and so on.
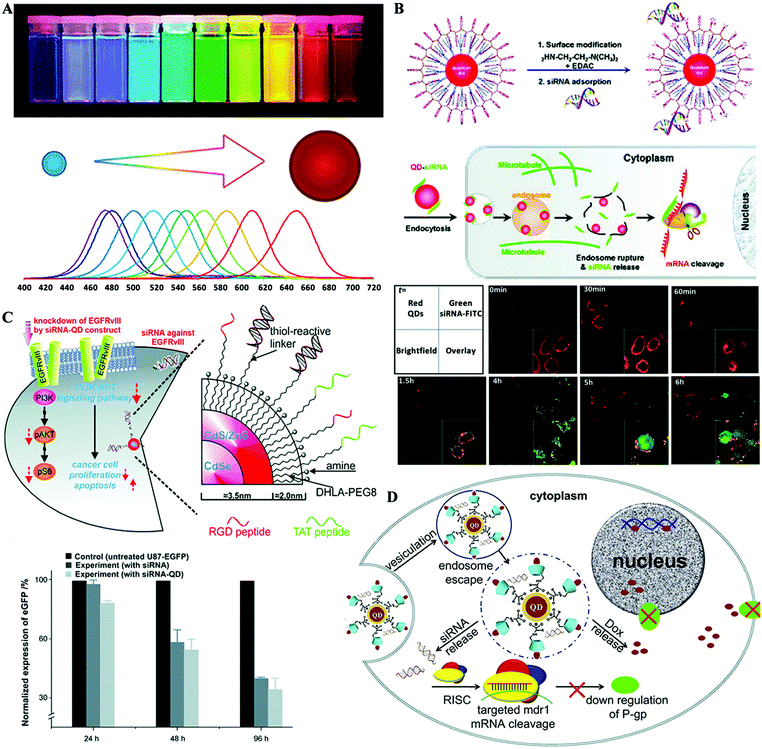 |
| Fig. 7 Hybrid QD nanoformulated siRNA therapeutics. (A) The optical property of QDs; size dependent emission wavelength. (B) Proton-sponge coated QDs for siRNA and real-time intracellular siRNA delivery tracking. (C) Multifunctional QD system for targeted siRNA delivery. (D) L-Arginine-β-cyclodextrin modified QDs for siRNA and Dox co-delivery to combat multidrug resistance. Figures reproduced with permission from (A) ref. 121, (B) ref. 124 and 131, (C) ref. 129, and (D) ref. 139. Copyright (A) 2010 the Royal Society of Chemistry, (B) 2008 American Chemical Society, (C) 2010 John Wiley & Sons, and (D) 2012 Elsevier B.V. | |
QDs were for the first time introduced into a siRNA delivery system by Bhatia et al. for monitoring the delivery process and improving the gene silencing effect.134 In this study, QDs were first modified with mercaptoacetic acid and thiol–PEG5000, and then incorporated into cationic liposomes along with siRNA. Taking advantage of the superior photostability and tunable optical properties of QDs, they used a flow cytometer to track delivery of nucleic acids, sort cells by the degree of transfection and purify homogenously-silenced subpopulations. Moreover, a multiplexed gene knockdown study was conducted using two kinds of QDs with different colors for different targeting siRNA labeling. For cellular siRNA delivery, endosome escape is a critical issue, because delivery complexes are usually internalized by cells through the endocytosis pathway, easily resulting in enzymatic degradation of the payload siRNA.124,131 Gao et al. reported engineering of QD surfaces by coating with proton-absorbing polymers, with a balanced composition of tertiary amine and carboxylic acid groups (Fig. 7B).124,131 The intracellular behavior of QD–siRNA complexes, including uptake, transport, and localization in live cells, was investigated by fluorescence tracking. It was demonstrated that the proton-sponge coating on the QD surface led to efficient siRNA release from intracellular vesicles, which achieved 10–20-fold improvement in gene silencing efficiency.
Cancer cell targeted siRNA vectors further improve the cancer specificity and therapeutic efficiency, and active tumor targeting moieties are usually conjugated to the carriers for molecular recognition of unique cancer-specific markers. With excellent fluorescence properties, siRNA nanocarriers engineered from QDs offer great advantages for targeted siRNA delivery tracking, mechanistic studies and therapeutic efficiency evaluation.129,130,135,136 Lee et al. developed a multifunctional delivery platform based on QDs for targeted EGFRvIII gene down-regulation (Fig. 7C).129 RGD and HIV-TAT peptides were conjugated to the QD surface to target the integrin receptor protein αvβ3 overexpressed on U87 cells and to enhance the cellular transfection efficiency of the QDs–siRNA complex. Target-specific delivery of siRNA was demonstrated by tracking the QD fluorescence in a novel co-culture system containing the U87-EGFP cell line with other less-tumorigenic cell lines, such as PC-12 cells. In another study, chimeras composed of the aptamer targeting prostate-specific membrane antigen (PSMA) and siRNA were delivered by positively charged QDs which were engineered by coating hydrophobic QDs with an amphiphilic copolymer poly(maleic anhydride-alt-1-tetradecene) and then conjugating with PEI.130 The central concern of this work is to evaluate the cellular uptake efficiency of the chimeras-QDs complex established through two different approaches: one-step direct adsorption of chimeras on QDs and a two-step method by sequential adsorption of siRNA and the conjugation aptamer onto siRNA. Finally, fluorescence tracking of QDs demonstrated that the two-step approach could greatly retain the conformation and high accessibility of the targeting aptamer moieties to achieve significantly higher transfection efficiency and targeting gene down-regulation ability.
With well-defined surface organic ligand engineering, QDs could also be used as siRNA and small molecule co-delivery vectors. A series of biocompatible QDs modified with amino acid grafted β-cyclodextrin (β-CD) derivatives have been reported.137–139 The arginine–β-CD modified QDs (Arg–CD-QDs) not only have a positively charged surface to absorb siRNA, but also a hydrophobic cavity of β-CD, which serves as a molecular capsule to encapsulate doxorubicin (Dox) (Fig. 7D).139 The siRNA was designed to target and silence the multidrug resistance gene (MDR-1), which is responsible for multidrug resistance in cancer cells. By simultaneous transportation of siRNA and Dox across the cell membrane to down-regulate the expression of P-glycoprotein (P-gp), the multidrug resistance could be reversed and as a result the efficiency of Dox can be improved in multidrug resistant cancer cells.
5.3. Iron oxide nanoparticles
Magnetic iron oxide nanoparticles (mostly maghemite, γ-Fe2O3 or magnetite, Fe3O4) are well-established nanomaterials that possess unique magnetic properties. IONPs are being actively investigated as new generation of magnetic resonance imaging (MRI) contrast agents (Fig. 8A and B).56,140–143 Owing to their unique characteristics, including efficient contrast effects, excellent biocompatibility and versatile surface functionalization capability, IONPs are extensively explored for various biomedical applications, such as medical diagnosis, detoxification of biological fluids, protein purification, cell separation, chronic iron deficiency anemia treatment, hyperthermia, drug/gene delivery, and so on.144–146 The excellent biocompatibility of IONPs has been widely demonstrated in a number of studies in vitro and in vivo, because iron is one of the most abundant endogenous metallic elements in living organisms and is essential for various biological processes. IONPs can be degraded in the body and subsequently incorporated into iron pools or used in metabolic processes.147 Several IONP-based nanoformulations have been approved or are in the clinical trial stage as MRI contrast agents or therapeutic agents.148–150
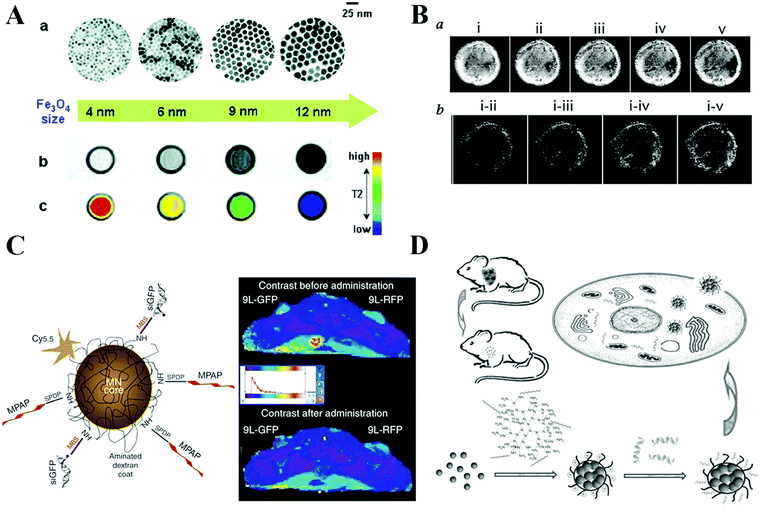 |
| Fig. 8 Hybrid magnetic nanoformulated siRNA therapeutics. (A) The magnetic resonance imaging property of representative IONPs. Nanoscale size effect on magnetism and induced MR signals. (B) IONPs for targeted in vivo tumor imaging. (C) Multifunctional IONPs for in vivo siRNA delivery and imaging. (D) Amphiphilic alkyl-PEI functionalized clustered IONPs for siRNA delivery and imaging. Figures reproduced with permission from (A) ref. 56, (B) ref. 142, (C) ref. 38, and (D) ref. 159. Copyright (A) 2005 American Chemical Society, (B) 2001 and (C) 2007 Springer Nature, and (D) 2011 John Wiley & Sons. | |
MRI has a number of unique advantages including deep tissue penetration, high spatial resolution, and excellent soft tissue contrast.140 Therefore, IONP-based delivery systems possess great promise for simultaneous in vivo transportation tracking, biodistribution imaging, and drug accumulation evaluation. Moore et al. developed a dual-purpose probe by surface engineering of IONPs for in vivo transfer of siRNA and the simultaneous imaging of its accumulation in tumors by high-resolution MRI and near-infrared in vivo optical imaging (Fig. 8C).38 The multifunctional delivery vector is synthesized through a step-by-step method: IONPs were first coated with dextran and then conjugated with Cy5.5 dyes and covalently linked to siRNA molecules specific for model (GFP) or therapeutic (survivin) targets, and this nanocarrier is also modified with myristoylated polyarginine peptides (MPAP) serving as a membrane translocation module. The in vivo transportation of siRNA mediated by the IONPs could be monitored by MRI and optical imaging. In addition, the imaging results of the gene silencing process could be correlated with histological data. In another study, a similar all-in-one strategy is used for siRNA delivery and simultaneous in vivo transportation monitoring. Bovine serum albumin (BSA)-coated manganese-doped magnetism-engineered iron oxide (MnMEIO) nanoparticles were used as the core material.151 The MnMEIO NPs were further modified with Cy5 dyes for subcellular imaging and cyclic RGD peptides for cancer cell targeted delivery. The multifunctional theranostic nanovector was designed to enable highly accurate imaging to be carried out simultaneously with the delivery of therapeutic siRNA, which can minimize invasiveness and deleterious side effects.
To engineer IONPs for specific biomedical applications, surface functionalization with proper organic ligands should be first carried out to make them water-soluble, biocompatible, or to introduce functional groups for further conjugation. The strategies for IONP surface engineering for siRNA delivery could be divided into several categories: amphiphilic polymer coating,152–154 ligand exchange155–157 and in situ coating during the synthesis process.158 Chen et al. used amphiphilic alkyl-PEI2k as a surface engineering ligand to assemble with hydrophobic IONPs to form cationic clusters for binding siRNA (Fig. 8D).159 The cluster nanocarrier could protect siRNA from enzymatic degradation in serum, and release complexed siRNA efficiently in the presence of polyanionic heparin. The excellent gene silencing efficiency of the siRNA-loaded system is assessed with 4T1 cells stably expressing luciferase (fluc-4T1) and with a fluc-4T1 xenograft model. Moreover, unlike high-molecular-weight analogues, the alkyl-PEI2k-coated IONPs show good biocompatibility. Pierre et al. synthesized two types of polymers, i.e. homopolymers poly(oligoethylene glycol)methyl ether acrylate (p(OEG-A)), poly(dimethylaminoethyl acrylate) (p(DMAEA)) and block poly(DMAEA-b-OEG-A) polymers, as the surface exchange ligands.160 IONPs with a diameter of about 8 nm were obtained. These polymers were grafted separately onto IONPs using the strong affinity of the phosphonic acid terminal group to the IONP surface yielding IONPs@p(OEG-A), IONPs@p(DMAEA) and IONPs@p[(DMAEA)-b-(OEG-A)]. These polymers confer good stability to IONPs in aqueous solution and 50% fetal bovine serum (FBS) medium because P(OEG-A) chains effectively mask the positive charge originating from p(DMAEA), thereby limiting protein adsorption on these particles. Hybrid nanoparticles were exploited for siRNA complexation, thereby generating IONPs/siRNA nano-carriers with anti-fouling p(OEG-A) shells. Excellent gene silencing efficiency was achieved on human neuroblastoma SHEP cells both in the presence and in the absence of a magnetic field in FBS containing medium. Taking advantage of the well-established silica coating chemistry, a layer of silica shell could be first deposited on the IONP surface as a platform for further modifications to generate a multifunctional delivery system. Magnetic mesoporous silica nanoparticles (M-MSNs) were constructed to load siRNAs into the mesopores of M-MSNs, followed by polyethylenimine (PEI) capping, PEGylation and fusogenic peptide KALA modification.161 The resultant delivery system exhibited prolonged half-life in bloodstream, enhanced cell membrane translocation and endosomal escapability, and favorable tissue biocompatibility and biosafety. The therapeutic effect of this delivery system carrying vascular endothelial growth factor (VEGF) siRNA was proved on both subdermal and orthotopic lung cancer models with remarkable tumor suppression, while tumor metastasis was also significantly reduced, overall leading to improved survival.
5.4. Upconversion nanoparticles
Lanthanide-doped upconversion nanoparticles (UCNPs) exhibit unique luminescence properties to utilize sequential absorption of multiple photons through the use of long lifetime and real ladder-like energy levels of trivalent lanthanide ions to produce higher energy anti-Stokes luminescence. They thereby convert two or more long-wavelength excitation photons, generally NIR light, into shorter wavelength emissions (e.g., NIR, visible, and UV) through a process known as photon upconversion (Fig. 9A).162 UCNPs possess a lot of advantages for bioimaging, such as high tissue penetration depth, low autofluorescence background, sharp emission bandwidths, large anti-Stokes shifts, high resistance to photobleaching, and high temporal resolution.163–166 In recent years, UCNPs have been extensively developed as a new class of luminescent agents that have become promising candidates for use in various biomedical applications including imaging, drug delivery, and therapy.167–169
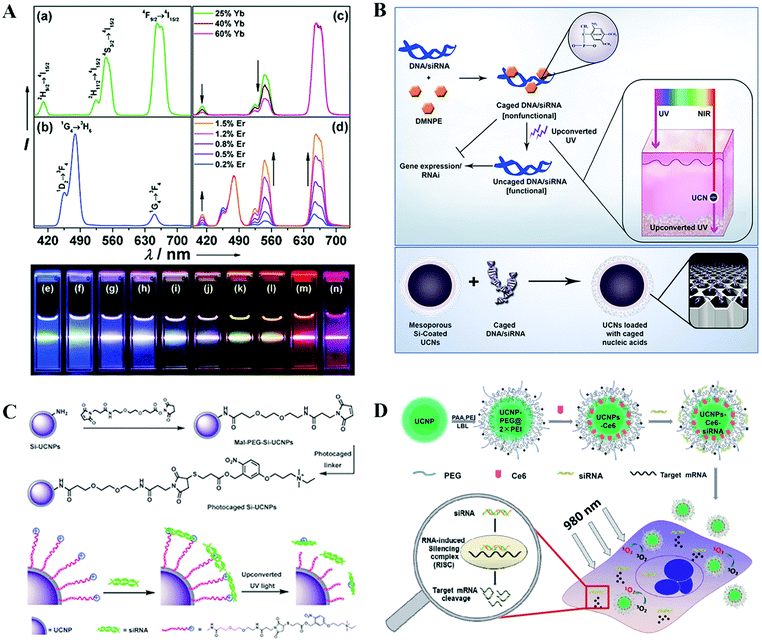 |
| Fig. 9 Hybrid UCNP nanoformulated siRNA therapeutics. (A) The unique optical properties of UCNPs. (B) UCNPs for siRNA delivery and NIR light triggered siRNA activation. (C) UCNPs for siRNA delivery and NIR light activated siRNA release. (D) UCNPs for siRNA and photosensitizer Ce6 co-delivery for NIR-triggered photodynamic therapy combined with gene therapy for a synergistically enhanced cancer cell killing effect. Figures reproduced with permission from (A) ref. 162, (B) ref. 175, (C) ref. 176, and (D) ref. 182. Copyright (A) 2008 American Chemical Society, (B) 2012 National Academy of Sciences, (C) 2013 and (D) 2014 the Royal Society of Chemistry. | |
Owing to the unique and amazing luminescence properties of UCNPs, they have been considered to be promising core structures for light-triggered delivery systems. Light-triggered drug delivery platforms have emerged as an elegant and non-invasive system for drug payload release in a remote spatiotemporal controllable manner at the desired site and time. This control is considered crucial to boost controlled local effective drug accumulation while minimizing side effects, therefore resulting in improved therapeutic efficacy.170–173 UCNPs offer an excellent choice for this task due to their utilization of NIR-excitation wavelength exhibiting the ability to penetrate deeply into living tissues without causing phototoxic effects, which shows great superiority over high energy UV/visible light and expensive high intensity pulsed lasers to activate the photosensitive component. In recent years, great breakthroughs have been successfully achieved by utilizing UCNP systems to realize NIR light-triggered drug release for chemotherapy. This concept has also been successfully applied for siRNA delivery and NIR light-triggered activation.174–178 Zhang et al. reported the exploitation of the potential of NIR-to-UV UCNPs to achieve photoactivation of caged siRNA for photo-controlled gene regulation (Fig. 9B).175 In this study, siRNAs were caged with light-sensitive 4,5-dimethoxy-2-nitroacetophenone (DMNPE) and loaded into the pores of mesoporous silica coated UCNPs by physical adsorption. DMNPE-caged siRNA molecules were shown to be uncaged and turned to functional genes as designed to silence the targeted gene after being activated with NIR light irradiation. This concept was fully proved by the significant decrease in GFP fluorescence for caged GFP siRNA delivery in GFP expressing B16 cells and furthermore in vivo in animal models with deep tissue penetration. In another study, Xing et al. developed UCNPs with surface functionalization by cationic photocaged linkers through covalent bonding, which could effectively adsorb anionic siRNA through electrostatic attraction (Fig. 9C).176 The UCNPs–siRNA complexes could be easily internalized by living cells. Upon NIR light irradiation, the photocaged linker on the UCNPs surface could be cleaved by the upconverted UV light and thus initiated the intracellular release of the siRNA. The in vitro agarose gel electrophoresis and intracellular imaging results indicated that the UCNP-based gene carrier system allowed effective siRNA delivery. The applications of NIR light instead of direct high energy UV irradiation may greatly guarantee less cell damage.
UCNPs are also shown to be an alternatively new choice for photodynamic therapy (PDT) with NIR light excitation.179,180 This promising approach was introduced for efficient siRNA delivery and therapy by combination with photochemical internalization, PDT or photothermal therapy (PTT) to achieve a synergistic tumor therapy effect.181–183 Positively charged UCNPs were engineered via a layer-by-layer strategy and further loaded simultaneously with chlorin e6 (Ce6), a photosensitizing molecule, and siRNA, which targets the Plk1 oncogene (Fig. 9D).182 Under excitation by NIR light at 980 nm, cytotoxic singlet oxygen can be generated via resonance energy transfer from UCNPs to photosensitizer Ce6, while the residual upconversion luminescence is utilized for imaging. The silencing of Plk1 induced by siRNA delivered with UCNPs could induce significant cancer cell apoptosis. As a result of such combined photodynamics with gene therapy, a remarkably enhanced cancer cell killing effect is realized. Gd-Doped UCNPs were synthesized and modified with cationic polymers, which were further conjugated with cypate and loaded with HSP70–siRNA against heat shock protein 70 (HSP70).183 Cypate, a type of organic carbocyanine fluorophore, displays a high molar extinction coefficient for NIR light, thereby exhibiting high photothermal conversion efficiency for cancer photothermal therapy. HSP70 are usually upregulated under heat stress, thus protecting cells from hyperthermic damage. Therefore, this UCNPs–cypate–siRNA system could simultaneously generate a photothermal effect to destroy cancer cells and inhibit the expression of HSP70 to achieve a synergistically enhanced antitumor therapy effect. Moreover, this multifunctional platform provided a multimodal imaging-guided system for precisely controllable antitumor theranostics.
6. Non-metallic core hybrids with organic shell delivery systems
The intrinsic physical and chemical properties of non-metallic materials also play a critically important role in the development progress in medicine and other industries, e.g., the well-known arsenic trioxide, also named pi-shuang in traditional Chinese medicine.184 It has long been of biomedical interest and it is currently being used to treat cancer like acute promyelocytic leukemia (APL). Another case, selenium (Se) is an essential dietary component for humans, and recent increasing reports and evidence show it to be a promising cancer chemopreventive element.185 With the rapid development of nanotechnology, it has brought great opportunities to use those non-metallic materials as nanocarriers for gene therapies, chemotherapies and other small molecule drugs. Se-based nanoparticles were successfully used to deliver siRNA to inhibit epidermal growth factor receptor signaling, or a tumor microenvironment responsive delivery system to enhance the nanotherapeutics.186,187 Specifically, both silicon and carbon elements in group IV are the most abundant and significant non-metallic substances in human tissues. These two kinds of typical materials have been designed, synthesized and applied in various research fields including nanomedicine. The advantages of these systems include large surface area, stability, biocompatibility, facile synthesis, easy surface functionalization, high penetration capacity to biological barriers, ultra-high loading capacity and so on.188 Silicon based delivery systems, such as mesoporous silica nanoparticles (MSNs), nanosized silicon particles and nanoporous silicon microparticles (also named multistage vector, MSV) have attracted increasing attention. Additionally, carbon based platforms including carbon dots, carbon tubes, graphene, fullerene, nanodiamond and so on have also been studied.189–191 They have emerged as a booming area for the development of delivery vehicles and for diagnostic agents and anticancer drugs. In the following, we expand the siRNA therapeutics based on silica nanoparticles, silicon nano/microparticles and carbon vectors with further fabrication of organic shells for treatment of human diseases.
6.1. Silicon nano/microparticles
Silicon nanoparticles have been investigated since the 1990s. Silicon offers specific physical and chemical properties, size-dependent multicolor reflection of light, photobleaching stability and favorable non-toxicity.192 Silicon is an “indirect band-gap material” unlike other semiconductors and shows particular changes when its size approximates the bulk Bohr radius (4 nm). Porous silicon with the advantages of biocompatibility, biodegradability and unique physical properties has great potential as a drug delivery platform for various therapeutic agents including chemotherapeutics, genes, and small molecular diagnostics.193,194 The in vivo biosafety and efficacy are highly dependent on the surface functionalized properties (size, fluorescent dye, peptides, targeting moieties and geometries), administration dosage and routes (Fig. 10A).195 A typical example of silicon carriers for siRNA delivery is the multistage vector system (MSV); this system encompasses three components and each optimized stage to address a different set of biological barriers. (1) Porous silicon microparticles as a first stage component that can be loaded with different nanoparticles. (2) The second stage includes micelles, liposomes, polymeric nanoparticles or metallic nanoparticles, etc. (3) The third stage is the payload drugs including siRNA, chemotherapeutics, miRNA, and antibodies (Fig. 10A).196
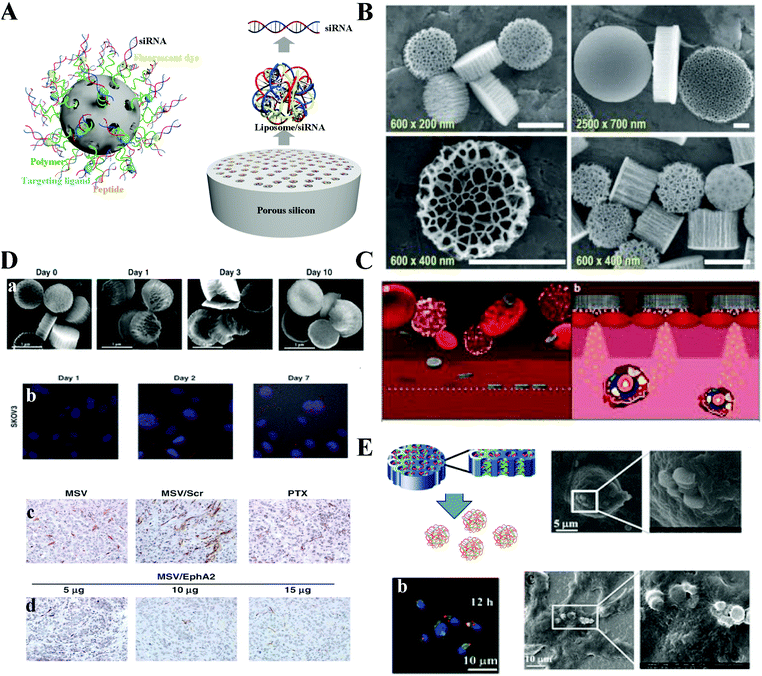 |
| Fig. 10 Hybrid silicon formulated siRNA therapeutics. (A) Schematic of silicon based nano/microparticles with different size and modification. (B) Scanning electron microscopy (SEM) images of unloaded MSVs with various dimensions and pore sizes. (C) The circulation routine and mechanism of MSVs. (D) Combination of MSV/EphA2 siRNA and paclitaxel for treatment of metastatic ovarian cancer. (E) Polycation nanoporous silicon (PCPS) releases self-assembled secondary nanoparticles for siRNA delivery. Figures reproduced with permission from (B) ref. 197, (C) ref. 195, (D) ref. 198, and (E) ref. 182. Copyright (B) 2015 Elsevier B.V. (C) 2012 Springer Nature, (D) 2013 the American Association for Cancer Research, and (E) 2013 American Chemical Society. | |
Up to now, the protocol for mass production of MSV microparticles has been well established according to the United States Food and Drug Administration's (FDA's) Current Good Manufacturing Practices (cGMPs). Discoidal MSVs with different diameters (500–2600 nm), various heights (200–700 nm), pore sizes (5–150 nm), and high porosity (40–90%) can be adjusted by modulating the electrochemical etching and photolithography processes (Fig. 10B).197 Consequently, such physical properties of MSVs enable generation of particles with various performance attributes, nanoparticle-loading capacity, drug release profile, biodistribution and degradation kinetics. Additionally, MSVs could successfully stain in the tumor blood vessels because of their geometrical properties and the significant difference in structure between the tumor and normal vessels. When the silicon microparticles gradually degrade, second stage nanoparticles are released into tumor tissues. These secondary nanoparticles could further protect the therapeutic drugs from degradation and promote intracellular uptake in cancer cells. Finally, the third stage anticancer drug is typically released from the second stage nanoparticles into targeted cells (Fig. 10C).195 The MSVs with the novelty of multistage design could sequentially overcome the biological barriers to enhance the accumulation in the disease tissues and improve the drug concentration in the targeted cells.
Based on MSV systems, Ferrari's groups encapsulated the liposome or polymer siRNA formulated secondary nanoparticles inside the nanopores and successfully applied in the treatment of triple negative breast cancer, bone marrow metastatic breast cancer, metastatic ovarian cancer and inflammatory disease.198–203 Shen et al. used 1000 nm (diameter) and 400 nm (height) MSVs with further optimization through 3-aminopropyl triethoxysilane (APTES) to obtain a slight positive charge, which facilitated loading of slightly negative liposomes into the nanopores. Additionally, APTES incorporated MSVs reveal more stable ability in the PBS/FBS (10% FBS) mixture solutions. By day 10, most of the porous silicon particles maintain clear edges and a well-defined nanoporous structure. This system could successfully deliver siRNA inside SKOV3 (Fig. 10D).198 Meanwhile, the siRNA with a sustained release from the MSVs maintained the down-regulated protein expression from day 3 to day 9. The in vivo results also indicated that this platform could suppress the EphA2 protein expression and trigger the reduction of the total number of microvessels in tumor samples with a dose-dependent behavior. Importantly, tumor growth was completely inhibited when mice were treated with MSV/EphA2 siRNA in combination with paclitaxel.
Besides the above concept, Shen et al. further developed a system conceptually different from the multistage system. Therapeutic siRNA or microRNA are not pre-packaged, nor are they loaded in the form of nanoparticles in the polycation nanoporous silicon (PCPS) system.204,205 Instead, they bind directly to the polycation inside the nanopores, thus providing a very convenient approach to load a large quantity of therapeutic agents. The therapeutic agents and polymers will be slowly released and self-assembled into nanoparticles upon silicon particle degradation, thus avoiding a sudden burst of release; their study has clearly demonstrated effective cellular internalization and tumor enrichment (Fig. 10E).205 Release of therapeutics is achieved during porous silicon degradation, which is another difference from the multistage vector system. This platform has the following advantages: (1) high loading capacity, (2) low or no toxicity, (3) a friendly production protocol, and (4) stability during transportation and storage. Additionally, the self-assembled nanoparticles could successfully help siRNA polyplexes to escape from the endosome and lysosome to the cytoplasm with the benefits from the PEI moieties triggering the proton sponge effects. Furthermore, systemic delivery of PCPS/STAT3 siRNA in the murine model of MDA-MB-231 breast cancer enriched particles in tumor tissues and reduced STAT3 expression in cancer cells, causing significant reduction of cancer stem cells in the residual tumor tissue.205 Totally, their new multistage concept has a high loading capacity and no detectable toxicity. These inorganic (silicon) and organic (liposome or polymer) hybrid systems not only address the biological barriers for siRNA delivery to the targeted tissue and cells, but also could maintain a sustained gene silencing expression.
6.2. Mesoporous silica nanoparticles
Recently, mesoporous silica nanoparticles (MSNs) have drawn great attention for nucleic acid transportation due to their biocompatibility, definable morphology, large surface area for massive loading amount of cargos.206–208 For delivery application, MSNs exhibited unique features upon chemical modifications of outer and inner surfaces of the MSNs with various bioactive macromolecules including PEG, dendrimers, antibodies, aptamers, peptides and cationic polymers.208–213 Specifically, a surface coating of cationic polymers enables siRNAs to become tightly associated with the MSN and be released into the cytoplasm following cellular uptake, while targeting ligands induce rapid internalization (Fig. 11A). Therefore, MSNs have also been successfully administered as effective gene delivery devices in different cancers.214,215 For instance, Xia et al. studied the transfection efficiency of a MSNs/siRNA delivery system on GFP-HEPA cells by noncovalent attachment of different molecular weight polyethyleneimine (PEI) polymers to the surface of MSNs as a siRNA delivery platform (Fig. 11B).216 After a comparison of the cellular uptake, cytotoxicity and transfection efficiency of PEI-coated MSNPs, they demonstrated that 10 kDa PEI-coated particles not only bind siRNA with high affinity, but also enable achievement of efficient nontoxic cellular delivery of these payloads. Additionally, due to their unique mesoporous scaffolds, MSNs can be easily loaded with both chemotherapy drugs and siRNAs and effectively co-deliver the payloads into tumor cells with a synergistic effect.217–219 Huan et al. reported that administration of MSNs loaded concurrently with doxorubicin and siRNA targets the P-glycoprotein (P-gp) to overcome multiple drug resistance (MDR). They showed that this co-delivery strategy of DOX and siRNA by MSNs was capable of increasing the intracellular drug concentration to levels exceeding that of free DOX which resulted in an increased killing of DOX compared with the free form on KB-1V MDR cancerous cells, which demonstrate that it is possible to use the MSN platform to effectively deliver a siRNA targeting the drug exporter gene that can be used to improve the sensitivity of the chemotherapeutic agent (Fig. 11C).220
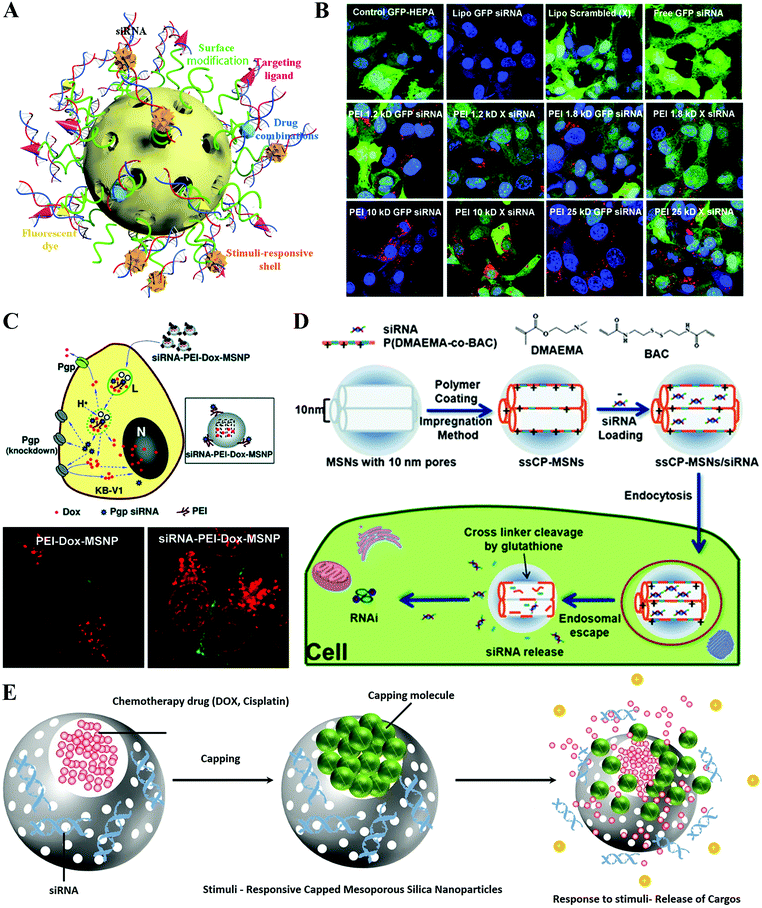 |
| Fig. 11 Hybrid silica nanoformulated siRNA therapeutics. (A) Schematic of multifunctional mesoporous silica nanoparticles (MSNs) for siRNA delivery. (B) MSN–PEI for siRNA delivery. (C) MSNs–PEI for co-delivering siRNA and doxorubicin (DOX) to treat the multiple drug resistance model (MDR). (D) Tumor microenvironment responsive MSN system for siRNA delivery. (E) Tumor microenvironment responsive MSN system for siRNA and DOX co-delivery. Figures reproduced with permission from (B) ref. 216, (C) ref. 220, (D) ref. 222, and (E) ref. 225. Copyright (B) 2009 and (C) 2010 American Chemical Society, (D) 2013 the Royal Society of Chemistry, and (E) 2017 Elsevier B.V. | |
During blood circulation of nanoparticles before reaching their site of action, undesirable release and degradation of loaded active siRNA in MSNs may occur due to the mesoporous structure, which is a major challenge that should be avoided as much as possible.221 To fulfill this purpose, the pores of MSNs could be capped with materials that are only sensitive to the tumor microenvironment, in which those capping molecules respond, followed by the opening of MSNP-locked valves to release the siRNA and other active reagents such as the chemo drug. Lin et al. reported a MSN based siRNA delivery system by capping the pores with poly(2-dimethylaminoethyl methacrylate) (PDMAEMA) cross-linked with a reductive sensitive linker (Fig. 11D).222 The results showed that the ssCP-MSNs exhibited an excellent siRNA binding capacity. After cellular uptake of ssCP-MSNs, high concentration of intracellular GSH triggers the release of loading siRNA by decapping the PDMAEMA. Moreover, this capping siRNA system exhibited obvious tumor suppression of the HeLa-Luc xenograft murine model after systemic administration. Overall, considering further evaluation of the anti-cancer activity of MSN based drugs and gene delivery platforms, tumor microenvironment-responsive capped MSNs loaded with hydrophobic or hydrophilic chemotherapy drugs in core siRNAs in the mesopores have been the most advanced and effective strategy so far.223,224 Such a responsive capping reagent is decapped because of the specific environment difference of tumor sites such as acidic and high redox nature. Administration of such MSNs with the least premature drug and siRNA release behavior significantly will improve the outcome of gene therapy (Fig. 11E).225,226
6.3. Carbon vectors
Up to now, carbon-based nanomaterials (CBNs) have also been actively investigated due to their advantageous chemical and physical properties (i.e., thermal effect, electrical conductivity, and high mechanical strength).227–229 This perspective highlights different types of carbon-based nanomaterials such as carbon nanotubes (CNTs), graphene, fullerene, carbon quantum dots and carbon fibers currently used in biomedical applications.230–233 Among the different applications, CBNs have attracted enormous interest as siRNA delivery platforms due to their extremely large surface area, with every atom exposed on their surface, which allows for ultra-high functionalization and loading capacities, high affinity towards biological molecules and facile surface functionalization (Fig. 12A).234–236
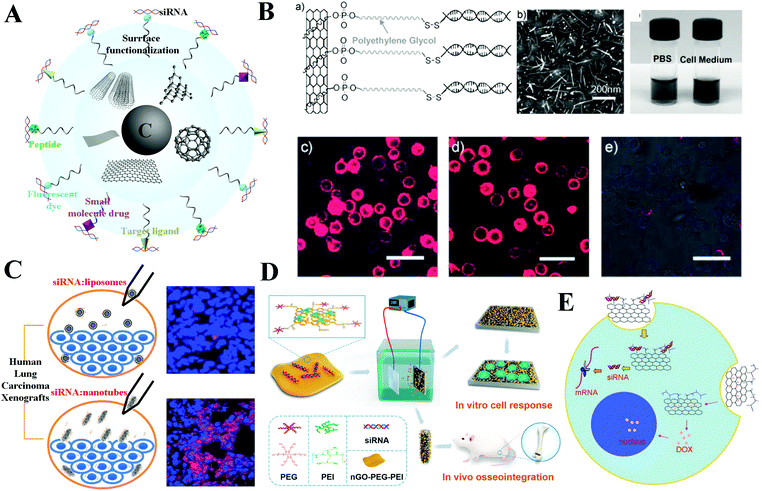 |
| Fig. 12 Hybrid carbon nanoformulated siRNA therapeutics. (A) Schematic of carbon based nanoparticles with different morphology and modification. (B) Single-walled carbon nanotubes (SWNTs) for siRNA delivery. (C) Multi-walled carbon nanotubes (MWNTs) for siRNA delivery. (D) Nanosized graphene oxide (nGO) system for siRNA delivery. (E) nGO system for co-delivery of siRNA and doxorubicin. Figures reproduced with permission from (B) ref. 221, (C) ref. 241, (D) ref. 249, and (E) ref. 251. Copyright (B) 2007 and (E) 2011 John Wiley & Sons, (C) 2015 and (D) 2017 American Chemical Society. | |
Carbon nanotubes are hollow 1-dimensional CBNs with a typical diameter of 1–2 nm and lengths ranging from 50 nm to 1000 nm. Their tubular morphology enables them to be easily and efficiently taken up by cells by acting as nano-shaped needles that make CNTs the most widely used CBNs in bio-application.237–239 Meanwhile, they are capable of encapsulating cargos and maintaining their function with controlled release of loaded molecules which is becoming increasingly important in gene delivery application. For siRNA delivery, both single-walled (SW) and multi-walled (MW) carbon nanotubes were modified or conjugated with siRNA for various disease treatments. Zhang et al. proposed an efficient vector based on SWNTs for siRNA to knockdown murine telomerase reverse transcriptase expression in murine tumor cells at both in vitro and in vivo levels for controlling key signaling regulators in cancer cells.240 Guo et al. established the capacity of cationic MWNT to deliver the apoptotic siRNA against PLK1 (siPLK1) in Calu6 tumor xenografts, which express high transfection efficiency compared with cationic liposomes. Moreover, the MWNTs/siRNA complexes significantly improve the xenograft animal survival rate. This study demonstrated the potential therapeutic efficacy of cationic MWNTs as a promising and safe siRNA delivery agent (Fig. 12C).241 Notably, novel “smart” single-walled carbon nanotubes (SWNTs), to achieve nanotube–siRNA conjugates with reductive cleavable disulfide bonds to enable intracellular controlled siRNA release from nanotube surfaces, were developed by Liu et al. as nonviral molecular transporters for the delivery of siRNA into human T cells and primary cells. This smart siRNA platform exhibited efficient RNA inference of CXCR4 and CD4 receptors on both human T cells and peripheral blood mononuclear cells (PBMCs). The cellular penetration ability and RNAi efficiency of nanotubes are far exceeding those of existing liposomes due to the underlying hydrophobic interactions upon nanotube-mediated molecular delivery (Fig. 12B).242
Graphene, a newly discovered two-dimensional carbon nanosheet structure,243–245 was demonstrated with additional remarkable colloidal stability, easily tunable surface functionalization, and good biocompatibility as a promising nano-carrier for safe and efficient gene transfection.246–248 Recently, its oxidized form, graphene oxide (GO), was used in a pioneering study by Zhang et al. for a PEI and PEG dual-modification step for complexing Ckip1-targeted siRNA, followed by deposition onto the pre-prepared Titania nanotube surface via cathodic electrodeposition to obtain a nGO–PEG–PEI/siRNA biofunctionalized implant for enhanced osteogenesis (Fig. 12D).249 The results demonstrated that together with Ckip-1 siRNA of osteogenic potential, both osteogenic differentiation on the NT-GPP/siCkip-1 implant surface in vitro and the new bone formation around the implant in vivo were significantly promoted which presents a promising implant biomodification siRNA delivery system based on functionalized GO.249 Ren et al. prepared a non-viral carrier (GO-PLL-SDGR) to deliver VEGF-siRNA for targeting cancer therapy. This GO-PLL-SDGR/siRNA complex could deliver VEGF-siRNA into tumor cells and down-regulate the expression of VEGF effectively to inhibit the tumor growth in a S180 xenograft tumor model.250 These results indicated that the targeting cationic GO/siRNA delivery system has great application potential in cancer therapy. Moreover, a GO based siRNA/drug co-delivery system was developed by Zhang et al., which led to a PEI-grafted GO nanocarrier for delivery of Bcl-2 siRNA and doxorubicin. The results reveal that the PEI–GO not only shows significantly lower cytotoxicity but also leads to significantly enhanced anticancer efficacy that may provide insight into the design and construction of GO based novel drug/gene co-delivery nanocarriers (Fig. 12E).251
Other CBNs such as carbon quantum dots (Cdots) are also widely applied in drug/gene delivery due to their photoluminescence nature.252,253 For instance, Wang et al. developed a novel targeting theranostic nanocarrier based on fc-rPEI–carbon quantum dots (rPEI–Cdots)/siRNA which can be used simultaneously in lung cancer diagnosis and gene therapeutics. The in vitro results showed that treating lung cancer cells with fc-rPEI–Cdots/pooled siRNA complex for 3 days reduced the cell vaibility to nearly 30%.236 Wang et al. also demonstrated Cdot-based and PEI-adsorbed complexes both as imaging agents and surviving siRNA nanocarriers, which indicate that Cdot-based nanocarriers are promising platforms for both siRNA delivery and imaging for cancer treatment.254
Overall, extensive research studies have elevated CBNs as one of the highlighted nanocarrier platforms for siRNA delivery owing to their unique combinations of structure, energetics, and electrical properties. The major disadvantage of CBNs as siRNA delivery agents is their non-biodegradability that may induce a range of adverse health effects. This point has been demonstrated in several studies and requires more future investigations.255,256
7. Conclusion and perspective
The recent announcement of Alnylam on the remarkably successful phase III results of Patisiran after two decades of the discovery of RNAi is encouraging.257 Meanwhile, resurgence in clinical trials using RNAi occurred in 2012; there are more than 30 RNAi-based therapeutics currently in clinical trials, and several of these are Phase III trials. However, considering the complicated biosystem and time consuming preclinical study, there are still a number of issues to address due to the instability of siRNA in body circulation and low bio-application. Luckily, it has been revealed that one of the major challenges indeed for RNAi-based therapy is the development of a delivery system. To solve this problem, a large number of carriers have been reported for siRNA delivery including virus and non-virus based platforms over the past two decades.
Among all kinds of siRNA carriers, inorganic–organic hybrid nanoparticles emerge as a diverse set of versatile platforms for both fundamental studies and potential clinical translation applications (Fig. 13). Here, we summarized the various choices for a broad range of covalent or non-covalent approaches for inorganic nanoparticle surface engineering to provide the ability to further optimize siRNA delivery in vitro and in vivo. Through the results, it could be concluded that the size and morphology of the inorganic cores showed great flexibility of tailoring to achieve better cellular uptake, blood circulation and specific bio-distribution. Meanwhile, the unique optical, magnetic and thermal properties of inorganic nanoparticles also have been extensively used to explore the crucial mechanisms of siRNA transportation in vitro and in vivo, such as endosome escape, reticuloendothelial system (RES) capture, kidney clearance, and enhanced penetration and retention (EPR) effect, etc. Decades of studies demonstrated that the therapeutic capability of inorganic–organic hybrid systems for siRNA delivery in a broad range of disease models shows great promise for future therapeutic applications. However, the remaining obstacles such as undesired systemic toxicity of hybrid systems hinder the translation of these delivery systems into clinics. Therefore, a lot of efforts are still needed to fill the space between basic research and clinical translation for these delivery systems (Fig. 13), for example, engineering and manufacturing siRNA-loaded inorganic nanoparticles with homogeneous size, composition, and surface charge in a simple, fast, and inexpensive manner; revealing the safety effect of siRNA-loaded nanoparticles with regard to administration, clearance routes, and potential long-term immune responses. In particular, with the development of immunology, a more careful evaluation of the immune responses of a biological system to the siRNA–nanoparticle complex after administration should be developed. For instance, the potential potent immune system response induced by siRNA molecules and delivery vectors is considered to be a great challenge, due to the difference between therapeutic effects from target-specific, RNAi-mediated gene silencing and those caused by nonspecific stimulation (i.e. inflammation/toxicity) of the innate immune system. A better understanding of the fundamental aspects of nanomaterial interactions with biological systems such as at the organismic, tissue, and cellular levels will further promote the development of this area.
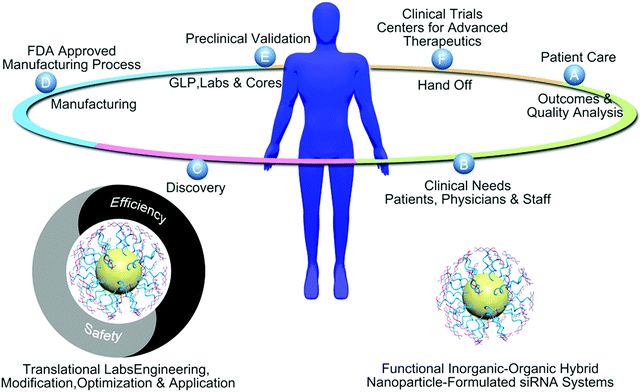 |
| Fig. 13 Inorganic–organic hybrid system formulated siRNA therapeutics for clinical needs. | |
Owing to the great properties of some inorganic nanoparticles, such as the excellent biostability of AuNPs and IONPs, the biodegradability and biocompatibility of silicon materials, their great potential for clinical translation applications has enlightened the pathway to the future. Taken together, RNAi therapy based on siRNA has the potential to revolutionize treatment of various kinds of cancers and other diseases. Innovative delivery systems based on inorganic–organic hybrid nanoparticles are highly promising to further enrich the fundamental theory of siRNA therapy, and develop safe and efficient delivery platforms for personalized cancer therapy in the future.
Conflicts of interest
The authors have declared that no conflicts of interest exist.
Acknowledgements
This work was funded by the National Natural Science Foundation of China (No. 21231007 and 21572282), the 973 program (No. 2014CB845604 and 2015CB856301), the Ministry of Education of China (No. IRT17R111), the Guangdong government (207999 and 2015A030306023) and the Fundamental Research Funds for the Central Universities; the Wenzhou Medical University and Wenzhou Institute of Biomaterials & Engineering (WIBEZD2017001-03); the Houston Methodist Research Institute, and the National Institute of Health (1R01CA193880-01A1).
References
- A. Jemal, R. Siegel, J. Xu and E. Ward, Ca-Cancer J. Clin., 2010, 60, 277–300 CrossRef PubMed.
- W. Chen, R. Zheng, P. D. Baade, S. Zhang, H. Zeng, F. Bray, A. Jemal, X. Q. Yu and J. He, Ca-Cancer J. Clin., 2016, 66, 115–132 CrossRef PubMed.
- C. E. DeSantis, R. L. Siegel, A. G. Sauer, K. D. Miller, S. A. Fedewa, K. I. Alcaraz and A. Jemal, Ca-Cancer J. Clin., 2016, 66, 290–308 CrossRef PubMed.
- R. L. Siegel, K. D. Miller and A. Jemal, Ca-Cancer J. Clin., 2016, 66, 7–30 CrossRef PubMed.
- R. Siegel, J. Ma, Z. Zou and A. Jemal, Ca-Cancer J. Clin., 2014, 64, 9–29 CrossRef PubMed.
- S. A. Kaliberov and D. J. Buchsbaum, Adv. Cancer Res., 2012, 115, 221–263 CrossRef CAS PubMed.
- Y. K. Oh and T. G. Park, Adv. Drug Delivery Rev., 2009, 61, 850–862 CrossRef CAS PubMed.
- K. A. Whitehead, R. Langer and D. G. Anderson, Nat. Rev. Drug Discovery, 2009, 8, 129–138 CrossRef CAS PubMed.
- A. Fire, S. Xu, M. K. Montgomery, S. A. Kostas, S. E. Driver and C. C. Mello, Nature, 1998, 391, 806–811 CrossRef CAS PubMed.
- R. Bernards, Ned. Tijdschr. Geneeskd., 2006, 150, 2849–2853 CAS.
- S. M. Elbashir, J. Harborth, W. Lendeckel, A. Yalcin, K. Weber and T. Tuschl, Nature, 2001, 411, 494–498 CrossRef CAS PubMed.
- Y. Singh, P. Murat and E. Defrancq, Chem. Soc. Rev., 2010, 39, 2054–2070 RSC.
- E. Miele, G. P. Spinelli, E. Miele, E. Di Fabrizio, E. Ferretti, S. Tomao and A. Gulino, Int. J. Nanomed., 2012, 7, 3637–3657 Search PubMed.
- A. Gallas, C. Alexander, M. C. Davies, S. Puri and S. Allen, Chem. Soc. Rev., 2013, 42, 7983–7997 RSC.
- A. Rolland, Adv. Drug Delivery Rev., 2005, 57, 669–673 CrossRef CAS PubMed.
- C. N. Landen, A. Chavez-Reyes, C. Bucana, R. Schmandt, M. T. Deavers, G. Lopez-Berestein and A. K. Sood, Cancer Res., 2005, 65, 6910–6918 CrossRef CAS PubMed.
- D. W. Northfelt, S. I. Hamburg, M. J. Borad, M. Seetharam, K. K. Curtis, P. Lee, B. Crowell, L. Vocila, P. Fredlund and M. J. Gilbert, J. Clin. Oncol., 2013, 31(15 suppl), TPS2621 Search PubMed.
- T. S. Zatsepin, Y. V. Kotelevtsev and V. Koteliansky, Int. J. Nanomed., 2016, 11, 3077–3086 CrossRef CAS PubMed.
- J. R. Whitfield, M. E. Beaulieu and L. Soucek, Front. Cell Dev. Biol., 2017, 5, 10 Search PubMed.
- J. E. Zuckerman, I. Gritli, A. Tolcher, J. D. Heidel, D. Lim, R. Morgan, B. Chmielowski, A. Ribas, M. E. Davis and Y. Yun, Proc. Natl. Acad. Sci. U. S. A., 2014, 111, 11449–11454 CrossRef CAS PubMed.
- G. Talia, K. E. Zorde, H. Ayala, G. R. Malka, H. Naama, S. Amiel, D. Abraham, H. Gil, D. E. Ben and R. Stephen, Oncotarget, 2015, 6, 24560–24570 Search PubMed.
- H. Yin, R. L. Kanasty, A. A. Eltoukhy, A. J. Vegas, J. R. Dorkin and D. G. Anderson, Nat. Rev. Genet., 2014, 15, 541–555 CrossRef CAS PubMed.
- B. Schultheis, D. Strumberg, A. Santel, C. Vank, F. Gebhardt, O. Keil, C. Lange, K. Giese, J. Kaufmann and M. Khan, J. Clin. Oncol., 2014, 32, 4141–4148 CrossRef CAS PubMed.
- J. E. Zuckerman and M. E. Davis, Nat. Rev. Drug Discovery, 2015, 14, 843–856 CrossRef CAS PubMed.
- R. Kanasty, J. R. Dorkin, A. Vegas and D. Anderson, Nat. Mater., 2013, 12, 967–977 CrossRef CAS PubMed.
- J. M. Benitez-Del-Castillo, J. Moreno-Montañés, I. Jiménez-Alfaro, F. J. Muñoz-Negrete, K. Turman, K. Palumaa, B. Sádaba, M. V. González, V. Ruz and B. Vargas, Invest. Ophthalmol. Visual Sci., 2016, 57, 6447 CAS.
- V. Gonzalez, J. Morenomontanes, B. Sadaba, V. Ruz and A. I. Jimenez, Invest. Ophthalmol. Visual Sci., 2012, 53, 575 Search PubMed.
- K. Vaziri, S. G. Schwartz, N. Relhan, K. S. Kishor and H. Flynn Jr, Rev. Diab. Studies, 2015, 12, 196–210 CrossRef PubMed.
- J. Callizo and H. T. Agostini, Ophthalmologe, 2010, 107, 1077–1080 CrossRef CAS PubMed.
- D. S. Boyer, J. J. Hopkins, J. Sorof and J. S. Ehrlich, Ther. Adv. Endocrinol. Metab., 2013, 4, 151–169 CrossRef CAS PubMed.
- C. F. Xu and J. Wang, Asian J. Pharm. Sci., 2015, 10, 1–12 CrossRef.
- J. C. Kaczmarek, P. S. Kowalski and D. G. Anderson, Genome Med., 2017, 9, 60 CrossRef PubMed.
- Y. Wen and W. S. Meng, J. Pharm. Innov., 2014, 9, 158–173 CrossRef PubMed.
- C. Tam, J. H. Wong, C. Rcf, T. Zuo and T. B. Ng, Appl. Microbiol. Biotechnol., 2017, 1–21 Search PubMed.
- A. Reynolds, D. Leake, Q. Boese, S. Scaringe, W. S. Marshall and A. Khvorova, Nat. Biotechnol., 2004, 22, 326–330 CrossRef CAS PubMed.
- B. Ozpolat, A. K. Sood and G. Lopez-Berestein, J. Intern. Med., 2010, 267, 44–53 CrossRef CAS PubMed.
- C. Wolfrum, S. Shi, K. N. Jayaprakash, M. Jayaraman, G. Wang, R. K. Pandey, K. G. Rajeev, T. Nakayama, K. Charrise and E. M. Ndungo, Nat. Biotechnol., 2007, 25, 1149–1157 CrossRef CAS PubMed.
- Z. Medarova, W. Pham, C. Farrar, V. Petkova and A. Moore, Nat. Med., 2007, 13, 372–377 CrossRef CAS PubMed.
- M. L. Hans and A. M. Lowman, Curr. Opin. Solid State Mater. Sci., 2002, 6, 319–327 CrossRef CAS.
- J. Panyam and V. Labhasetwar, Adv. Drug Delivery Rev., 2003, 55, 329–347 CrossRef CAS PubMed.
- A. Kumari, S. K. Yadav and S. C. Yadav, Colloids Surf., B, 2010, 75, 1–18 CrossRef CAS PubMed.
- S. T. Kim, A. Chompoosor, Y.-C. Yeh, S. S. Agasti, D. J. Solfiell and V. M. Rotello, Small, 2012, 8, 3253–3256 CrossRef CAS PubMed.
- C. Xu, K. Xu, H. Gu, R. Zheng, H. Liu, X. Zhang, Z. Guo and B. Xu, J. Am. Chem. Soc., 2004, 126, 9938–9939 CrossRef CAS PubMed.
- H. Otsuka, Y. Akiyama, Y. Nagasaki and K. Kataoka, J. Am. Chem. Soc., 2001, 123, 8226–8230 CrossRef CAS PubMed.
- A. G. Tkachenko, H. Xie, D. Coleman, W. Glomm, J. Ryan, M. F. Anderson, S. Franzen and D. L. Feldheim, J. Am. Chem. Soc., 2003, 125, 4700–4701 CrossRef CAS PubMed.
- J. Sharma, R. Chhabra, C. S. Andersen, K. V. Gothelf, H. Yan and Y. Liu, J. Am. Chem. Soc., 2008, 130, 7820–7821 CrossRef CAS PubMed.
- M. S. Yavuz, Y. Cheng, J. Chen, C. M. Cobley, Q. Zhang, M. Rycenga, J. Xie, C. Kim, K. H. Song, A. G. Schwartz, L. V. Wang and Y. Xia, Nat. Mater., 2009, 8, 935–939 CrossRef CAS PubMed.
- H. Mattoussi, J. M. Mauro, E. R. Goldman, G. P. Anderson, V. C. Sundar, F. V. Mikulec and M. G. Bawendi, J. Am. Chem. Soc., 2000, 122, 12142–12150 CrossRef CAS.
- F. Dubois, B. Mahler, B. Dubertret, E. Doris and C. Mioskowski, J. Am. Chem. Soc., 2007, 129, 482–483 CrossRef CAS PubMed.
- K. Susumu, E. Oh, J. B. Delehanty, J. B. Blanco-Canosa, B. J. Johnson, V. Jain, W. J. Hervey, W. R. Algar, K. Boeneman, P. E. Dawson and I. L. Medintz, J. Am. Chem. Soc., 2011, 133, 9480–9496 CrossRef CAS PubMed.
- M. Xie, H.-H. Liu, P. Chen, Z.-L. Zhang, X.-H. Wang, Z.-X. Xie, Y.-M. Du, B.-Q. Pan and D.-W. Pang, Chem. Commun., 2005, 5518–5520 RSC.
- Y. Liu, M. Kim, Y. Wang, Y. A. Wang and X. Peng, Langmuir, 2006, 22, 6341–6345 CrossRef CAS PubMed.
- M.-X. Zhao, Q. Xia, X.-D. Feng, X.-H. Zhu, Z.-W. Mao, L.-N. Ji and K. Wang, Biomaterials, 2010, 31, 4401–4408 CrossRef CAS PubMed.
- P. Zhang, S. Liu, D. Gao, D. Hu, P. Gong, Z. Sheng, J. Deng, Y. Ma and L. Cai, J. Am. Chem. Soc., 2012, 134, 8388–8391 CrossRef CAS PubMed.
- S. Kim and M. G. Bawendi, J. Am. Chem. Soc., 2003, 125, 14652–14653 CrossRef CAS PubMed.
- Y.-w. Jun, Y.-M. Huh, J.-s. Choi, J.-H. Lee, H.-T. Song, K. Kim, S. Yoon, K.-S. Kim, J.-S. Shin, J.-S. Suh and J. Cheon, J. Am. Chem. Soc., 2005, 127, 5732–5733 CrossRef CAS PubMed.
- M. Liong, H. Shao, J. B. Haun, H. Lee and R. Weissleder, Adv. Mater., 2010, 22, 5168–5172 CrossRef CAS PubMed.
- H. Gu, Z. Yang, J. Gao, C. K. Chang and B. Xu, J. Am. Chem. Soc., 2005, 127, 34–35 CrossRef CAS PubMed.
- S. Peng, C. Wang, J. Xie and S. Sun, J. Am. Chem. Soc., 2006, 128, 10676–10677 CrossRef CAS PubMed.
- U. I. Tromsdorf, O. T. Bruns, S. C. Salmen, U. Beisiegel and H. Weller, Nano Lett., 2009, 9, 4434–4440 CrossRef CAS PubMed.
- R. Naccache, F. Vetrone, V. Mahalingam, L. A. Cuccia and J. A. Capobianco, Chem. Mater., 2009, 21, 717–723 CrossRef CAS.
- G. S. Yi and G. M. Chow, Adv. Funct. Mater., 2006, 16, 2324–2329 CrossRef CAS.
- J.-C. Boyer, M.-P. Manseau, J. I. Murray and F. C. J. M. van Veggel, Langmuir, 2010, 26, 1157–1164 CrossRef CAS PubMed.
- N. Bogdan, F. Vetrone, R. Roy and J. A. Capobianco, J. Mater. Chem., 2010, 20, 7543–7550 RSC.
- G. Yi, Y. Peng and Z. Gao, Chem. Mater., 2011, 23, 2729–2734 CrossRef CAS.
- C.-Y. Lai, B. G. Trewyn, D. M. Jeftinija, K. Jeftinija, S. Xu, S. Jeftinija and V. S. Y. Lin, J. Am. Chem. Soc., 2003, 125, 4451–4459 CrossRef CAS PubMed.
- I. Slowing, B. G. Trewyn and V. S. Y. Lin, J. Am. Chem. Soc., 2006, 128, 14792–14793 CrossRef CAS PubMed.
- D. P. Ferris, Y.-L. Zhao, N. M. Khashab, H. A. Khatib, J. F. Stoddart and J. I. Zink, J. Am. Chem. Soc., 2009, 131, 1686–1688 CrossRef CAS PubMed.
- L. Pan, Q. He, J. Liu, Y. Chen, M. Ma, L. Zhang and J. Shi, J. Am. Chem. Soc., 2012, 134, 5722–5725 CrossRef CAS PubMed.
- W. Zhang, J. Shen, H. Su, G. Mu, J.-H. Sun, C.-P. Tan, X.-J. Liang, L.-N. Ji and Z.-W. Mao, ACS Appl. Mater. Interfaces, 2016, 8, 13332–13340 CAS.
- Z. Liu, J. T. Robinson, X. Sun and H. Dai, J. Am. Chem. Soc., 2008, 130, 10876–10877 CrossRef CAS PubMed.
- X. Sun, Z. Liu, K. Welsher, J. T. Robinson, A. Goodwin, S. Zaric and H. Dai, Nano Res., 2008, 1, 203–212 CrossRef CAS PubMed.
- C. F. Chin, S. Q. Yap, J. Li, G. Pastorin and W. H. Ang, Chem. Sci., 2014, 5, 2265–2270 RSC.
- X. Gao, Y. Cui, R. M. Levenson, L. W. K. Chung and S. Nie, Nat. Biotechnol., 2004, 22, 969–976 CrossRef CAS PubMed.
- G. Prencipe, S. M. Tabakman, K. Welsher, Z. Liu, A. P. Goodwin, L. Zhang, J. Henry and H. Dai, J. Am. Chem. Soc., 2009, 131, 4783–4787 CrossRef CAS PubMed.
- R. E. Anderson and W. C. W. Chan, ACS Nano, 2008, 2, 1341–1352 CrossRef CAS PubMed.
- L. Cheng, K. Yang, S. Zhang, M. Shao, S. Lee and Z. Liu, Nano Res., 2010, 3, 722–732 CrossRef CAS.
- T. Pellegrino, L. Manna, S. Kudera, T. Liedl, D. Koktysh, A. L. Rogach, S. Keller, J. Rädler, G. Natile and W. J. Parak, Nano Lett., 2004, 4, 703–707 CrossRef CAS.
- W. W. Yu, E. Chang, J. C. Falkner, J. Zhang, A. M. Al-Somali, C. M. Sayes, J. Johns, R. Drezek and V. L. Colvin, J. Am. Chem. Soc., 2007, 129, 2871–2879 CrossRef CAS PubMed.
- J. T. Robinson, S. M. Tabakman, Y. Liang, H. Wang, H. Sanchez Casalongue, D. Vinh and H. Dai, J. Am. Chem. Soc., 2011, 133, 6825–6831 CrossRef CAS PubMed.
- L. Wang, R. Yan, Z. Huo, L. Wang, J. Zeng, J. Bao, X. Wang, Q. Peng and Y. Li, Angew. Chem., Int. Ed., 2005, 44, 6054–6057 CrossRef CAS PubMed.
- Z. Yuan, N. Cai, Y. Du, Y. He and E. S. Yeung, Anal. Chem., 2014, 86, 419–426 CrossRef CAS PubMed.
- H. Pardoe, W. Chua-anusorn, T. G. St. Pierre and J. Dobson, J. Magn. Magn. Mater., 2001, 225, 41–46 CrossRef CAS.
- Q. Liu, C. Li, T. Yang, T. Yi and F. Li, Chem. Commun., 2010, 46, 5551–5553 RSC.
- Y. Wang, J. F. Wong, X. Teng, X. Z. Lin and H. Yang, Nano Lett., 2003, 3, 1555–1559 CrossRef CAS.
- Q. Liu, M. Chen, Y. Sun, G. Chen, T. Yang, Y. Gao, X. Zhang and F. Li, Biomaterials, 2011, 32, 8243–8253 CrossRef CAS PubMed.
- J. Li, Y.-C. Chen, Y.-C. Tseng, S. Mozumdar and L. Huang, J. Controlled Release, 2010, 142, 416–421 CrossRef CAS PubMed.
- W. T. Al-Jamal, K. T. Al-Jamal, P. H. Bomans, P. M. Frederik and K. Kostarelos, Small, 2008, 4, 1406–1415 CrossRef CAS PubMed.
- S. Jiang, A. A. Eltoukhy, K. T. Love, R. Langer and D. G. Anderson, Nano Lett., 2013, 13, 1059–1064 CrossRef CAS PubMed.
-
I. Bertini, H. B. Gray, S. J. Lippard and J. S. Valentine, Bioinorganic chemistry, University Science Books, 1994 Search PubMed.
- J. Forestier, Lancet, 1934, 224, 646–648 CrossRef.
- A. Albanese, P. S. Tang and W. C. W. Chan, Annu. Rev. Biomed. Eng., 2012, 14, 1–16 CrossRef CAS PubMed.
- C. M. Cobley, J. Chen, E. C. Cho, L. V. Wang and Y. Xia, Chem. Soc. Rev., 2011, 40, 44–56 RSC.
- E. C. Dreaden, A. M. Alkilany, X. Huang, C. J. Murphy and M. A. El-Sayed, Chem. Soc. Rev., 2012, 41, 2740–2779 RSC.
- L. Dykman and N. Khlebtsov, Chem. Soc. Rev., 2012, 41, 2256–2282 RSC.
- X. Yang, M. Yang, B. Pang, M. Vara and Y. Xia, Chem. Rev., 2015, 115, 10410–10488 CrossRef CAS PubMed.
- A. K. R. Lytton-Jean, R. Langer and D. G. Anderson, Small, 2011, 7, 1932–1937 CrossRef CAS PubMed.
- J. Guo, K. Rahme, K. A. Fitzgerald, J. D. Holmes and C. M. O’Driscoll, Nano Res., 2015, 8, 3111–3140 CrossRef CAS.
- S. H. Lee, K. H. Bae, S. H. Kim, K. R. Lee and T. G. Park, Int. J. Pharm., 2008, 364, 94–101 CrossRef CAS PubMed.
- J.-S. Lee, J. J. Green, K. T. Love, J. Sunshine, R. Langer and D. G. Anderson, Nano Lett., 2009, 9, 2402–2406 CrossRef CAS PubMed.
- J. Kim, S. Park, J. E. Lee, S. M. Jin, J. H. Lee, I. S. Lee, I. Yang, J.-S. Kim, S. K. Kim, M.-H. Cho and T. Hyeon, Angew. Chem., 2006, 118, 7918–7922 CrossRef.
- R. Huschka, A. Barhoumi, Q. Liu, J. A. Roth, L. Ji and N. J. Halas, ACS Nano, 2012, 6, 7681–7691 CrossRef CAS PubMed.
- Y. Xiao, R. Jaskula-Sztul, A. Javadi, W. Xu, J. Eide, A. Dammalapati, M. Kunnimalaiyaan, H. Chen and S. Gong, Nanoscale, 2012, 4, 7185–7193 RSC.
- F. Yin, C. Yang, Q. Wang, S. Zeng, R. Hu, G. Lin, J. Tian, S. Hu, R. F. Lan and H. S. Yoon, Theranostics, 2015, 5, 818–833 CrossRef CAS PubMed.
- A. Elbakry, A. Zaky, R. Liebl, R. Rachel, A. Goepferich and M. Breunig, Nano Lett., 2009, 9, 2059–2064 CrossRef CAS PubMed.
- S. Guo, Y. Huang, Q. Jiang, Y. Sun, L. Deng, Z. Liang, Q. Du, J. Xing, Y. Zhao, P. C. Wang, A. Dong and X.-J. Liang, ACS Nano, 2010, 4, 5505–5511 CrossRef CAS PubMed.
- L. Han, J. Zhao, X. Zhang, W. Cao, X. Hu, G. Zou, X. Duan and X.-J. Liang, ACS Nano, 2012, 6, 7340–7351 CrossRef CAS PubMed.
- A. C. Bonoiu, E. J. Bergey, H. Ding, R. Hu, R. Kumar, K.-T. Yong, P. N. Prasad, S. Mahajan, K. E. Picchione and A. Bhattacharjee, Nanomedicine, 2011, 6, 617–630 CrossRef CAS PubMed.
- J. Shen, H.-C. Kim, C. Mu, E. Gentile, J. Mai, J. Wolfram, L.-n. Ji, M. Ferrari, Z.-w. Mao and H. Shen, Adv. Healthcare Mater., 2014, 3, 1629–1637 CrossRef CAS PubMed.
- Z. Yang, T. Liu, Y. Xie, Z. Sun, H. Liu, J. Lin, C. Liu, Z.-W. Mao and S. Nie, Acta Biomater., 2015, 25, 194–204 CrossRef CAS PubMed.
- B.-K. Wang, X.-F. Yu, J.-H. Wang, Z.-B. Li, P.-H. Li, H. Wang, L. Song, P. K. Chu and C. Li, Biomaterials, 2016, 78, 27–39 CrossRef CAS PubMed.
- W.-J. Song, J.-Z. Du, T.-M. Sun, P.-Z. Zhang and J. Wang, Small, 2010, 6, 239–246 CrossRef CAS PubMed.
- S. Kirkland-York, Y. Zhang, A. E. Smith, A. W. York, F. Huang and C. L. McCormick, Biomacromolecules, 2010, 11, 1052–1059 CrossRef CAS PubMed.
- Y. Lei, L. Tang, Y. Xie, Y. Xianyu, L. Zhang, P. Wang, Y. Hamada, K. Jiang, W. Zheng and X. Jiang, Nat. Commun., 2017, 8, 15130 CrossRef PubMed.
- L. Shang, S. Dong and G. U. Nienhaus, Nano Today, 2011, 6, 401–418 CrossRef CAS.
- Y. Lu and W. Chen, Chem. Soc. Rev., 2012, 41, 3594–3623 RSC.
- M. Bruchez, M. Moronne, P. Gin, S. Weiss and A. P. Alivisatos, Science, 1998, 281, 2013–2016 CrossRef CAS PubMed.
- W. C. W. Chan and S. Nie, Science, 1998, 281, 2016–2018 CrossRef CAS PubMed.
- X. Michalet, F. F. Pinaud, L. A. Bentolila, J. M. Tsay, S. Doose, J. J. Li, G. Sundaresan, A. M. Wu, S. S. Gambhir and S. Weiss, Science, 2005, 307, 538–544 CrossRef CAS PubMed.
- A. M. Smith, H. Duan, A. M. Mohs and S. Nie, Adv. Drug Delivery Rev., 2008, 60, 1226–1240 CrossRef CAS PubMed.
- P. Zrazhevskiy, M. Sena and X. Gao, Chem. Soc. Rev., 2010, 39, 4326–4354 RSC.
- B. O. Dabbousi, J. Rodriguez-Viejo, F. V. Mikulec, J. R. Heine, H. Mattoussi, R. Ober, K. F. Jensen and M. G. Bawendi, J. Phys. Chem. B, 1997, 101, 9463–9475 CrossRef CAS.
- C. E. Probst, P. Zrazhevskiy, V. Bagalkot and X. Gao, Adv. Drug Delivery Rev., 2013, 65, 703–718 CrossRef CAS PubMed.
- L. Qi and X. Gao, ACS Nano, 2008, 2, 1403–1410 CrossRef CAS PubMed.
- A. Bonoiu, S. D. Mahajan, L. Ye, R. Kumar, H. Ding, K.-T. Yong, I. Roy, R. Aalinkeel, B. Nair, J. L. Reynolds, D. E. Sykes, M. A. Imperiale, E. J. Bergey, S. A. Schwartz and P. N. Prasad, Brain Res., 2009, 1282, 142–155 CrossRef CAS PubMed.
- N. Singh, A. Agrawal, A. K. L. Leung, P. A. Sharp and S. N. Bhatia, J. Am. Chem. Soc., 2010, 132, 8241–8243 CrossRef CAS PubMed.
- S. Li, Z. Liu, F. Ji, Z. Xiao, M. Wang, Y. Peng, Y. Zhang, L. Liu, Z. Liang and F. Li, Mol. Ther.–Nucleic Acids, 2012, 1, e20 CrossRef PubMed.
- P. Subramaniam, S. J. Lee, S. Shah, S. Patel, V. Starovoytov and K.-B. Lee, Adv. Mater., 2012, 24, 4014–4019 CrossRef CAS PubMed.
- J. Jung, A. Solanki, K. A. Memoli, K.-i. Kamei, H. Kim, M. A. Drahl, L. J. Williams, H.-R. Tseng and K. Lee, Angew. Chem., Int. Ed., 2010, 49, 103–107 CrossRef CAS PubMed.
- V. Bagalkot and X. Gao, ACS Nano, 2011, 5, 8131–8139 CrossRef CAS PubMed.
- M. V. Yezhelyev, L. Qi, R. M. O’Regan, S. Nie and X. Gao, J. Am. Chem. Soc., 2008, 130, 9006–9012 CrossRef CAS PubMed.
- T. Endres, M. Zheng, A. Kılıç, A. Turowska, M. Beck-Broichsitter, H. Renz, O. M. Merkel and T. Kissel, Mol. Pharmaceutics, 2014, 11, 1273–1281 CrossRef CAS PubMed.
- H. Zhu, S. Zhang, Y. Ling, G. Meng, Y. Yang and W. Zhang, J. Controlled Release, 2015, 220, 529–544 CrossRef CAS PubMed.
- A. A. Chen, A. M. Derfus, S. R. Khetani and S. N. Bhatia, Nucleic Acids Res., 2005, 33, e190–e190 CrossRef PubMed.
- W. B. Tan, S. Jiang and Y. Zhang, Biomaterials, 2007, 28, 1565–1571 CrossRef CAS PubMed.
- J. Park, J. Lee, J. Kwag, Y. Baek, B. Kim, C. J. Yoon, S. Bok, S.-H. Cho, K. H. Kim, G. O. Ahn and S. Kim, ACS Nano, 2015, 9, 6511–6521 CrossRef CAS PubMed.
- J.-M. Li, M.-X. Zhao, H. Su, Y.-Y. Wang, C.-P. Tan, L.-N. Ji and Z.-W. Mao, Biomaterials, 2011, 32, 7978–7987 CrossRef CAS PubMed.
- M.-X. Zhao, J.-M. Li, L. Du, C.-P. Tan, Q. Xia, Z.-W. Mao and L.-N. Ji, Chem. – Eur. J., 2011, 17, 5171–5179 CrossRef CAS PubMed.
- J.-M. Li, Y.-Y. Wang, M.-X. Zhao, C.-P. Tan, Y.-Q. Li, X.-Y. Le, L.-N. Ji and Z.-W. Mao, Biomaterials, 2012, 33, 2780–2790 CrossRef CAS PubMed.
- N. Lee and T. Hyeon, Chem. Soc. Rev., 2012, 41, 2575–2589 RSC.
- S. Laurent, D. Forge, M. Port, A. Roch, C. Robic, L. Vander Elst and R. N. Muller, Chem. Rev., 2008, 108, 2064–2110 CrossRef CAS PubMed.
- M. Zhao, D. A. Beauregard, L. Loizou, B. Davletov and K. M. Brindle, Nat. Med., 2001, 7, 1241–1244 CrossRef CAS PubMed.
- N. A. Frey, S. Peng, K. Cheng and S. Sun, Chem. Soc. Rev., 2009, 38, 2532–2542 RSC.
- J. Gao, H. Gu and B. Xu, Acc. Chem. Res., 2009, 42, 1097–1107 CrossRef CAS PubMed.
- R. Hao, R. Xing, Z. Xu, Y. Hou, S. Gao and S. Sun, Adv. Mater., 2010, 22, 2729–2742 CrossRef CAS PubMed.
- L. H. Reddy, J. L. Arias, J. Nicolas and P. Couvreur, Chem. Rev., 2012, 112, 5818–5878 CrossRef CAS PubMed.
- M. Levy, N. Luciani, D. Alloyeau, D. Elgrabli, V. Deveaux, C. Pechoux, S. Chat, G. Wang, N. Vats, F. Gendron, C. Factor, S. Lotersztajn, A. Luciani, C. Wilhelm and F. Gazeau, Biomaterials, 2011, 32, 3988–3999 CrossRef CAS PubMed.
- P. Reimer and T. Balzer, Eur. Radiol., 2003, 13, 1266–1276 Search PubMed.
- K. Maier-Hauff, F. Ulrich, D. Nestler, H. Niehoff, P. Wust, B. Thiesen, H. Orawa, V. Budach and A. Jordan, J. Neuro-Oncol., 2011, 103, 317–324 CrossRef PubMed.
- Y. Min, J. M. Caster, M. J. Eblan and A. Z. Wang, Chem. Rev., 2015, 115, 11147–11190 CrossRef CAS PubMed.
- J.-H. Lee, K. Lee, S. H. Moon, Y. Lee, T. G. Park and J. Cheon, Angew. Chem., Int. Ed., 2009, 48, 4174–4179 CrossRef CAS PubMed.
- X. Wang, Z. Zhou, Z. Wang, Y. Xue, Y. Zeng, J. Gao, L. Zhu, X. Zhang, G. Liu and X. Chen, Nanoscale, 2013, 5, 8098–8104 RSC.
- G. Lin, W. Zhu, L. Yang, J. Wu, B. Lin, Y. Xu, Z. Cheng, C. Xia, Q. Gong, B. Song and H. Ai, Biomaterials, 2014, 35, 9495–9507 CrossRef CAS PubMed.
- L. Qi, L. Wu, S. Zheng, Y. Wang, H. Fu and D. Cui, Biomacromolecules, 2012, 13, 2723–2730 CrossRef CAS PubMed.
- H. Mok, O. Veiseh, C. Fang, F. M. Kievit, F. Y. Wang, J. O. Park and M. Zhang, Mol. Pharmaceutics, 2010, 7, 1930–1939 CrossRef CAS PubMed.
- O. Veiseh, F. M. Kievit, H. Mok, J. Ayesh, C. Clark, C. Fang, M. Leung, H. Arami, J. O. Park and M. Zhang, Biomaterials, 2011, 32, 5717–5725 CrossRef CAS PubMed.
- M. Shen, F. Gong, P. Pang, K. Zhu, X. Meng, C. Wu, J. Wang, H. Shan and X. Shuai, Int. J. Nanomed., 2012, 7, 3319–3332 CrossRef CAS PubMed.
- O. Veiseh, F. M. Kievit, C. Fang, N. Mu, S. Jana, M. C. Leung, H. Mok, R. G. Ellenbogen, J. O. Park and M. Zhang, Biomaterials, 2010, 31, 8032–8042 CrossRef CAS PubMed.
- G. Liu, J. Xie, F. Zhang, Z. Wang, K. Luo, L. Zhu, Q. Quan, G. Niu, S. Lee, H. Ai and X. Chen, Small, 2011, 7, 2742–2749 CrossRef CAS PubMed.
- C. Boyer, P. Priyanto, T. P. Davis, D. Pissuwan, V. Bulmus, M. Kavallaris, W. Y. Teoh, R. Amal, M. Carroll, R. Woodward and T. St Pierre, J. Mater. Chem., 2010, 20, 255–265 RSC.
- Y. Chen, H. Gu, D. S.-Z. Zhang, F. Li, T. Liu and W. Xia, Biomaterials, 2014, 35, 10058–10069 CrossRef CAS PubMed.
- F. Wang and X. Liu, J. Am. Chem. Soc., 2008, 130, 5642–5643 CrossRef CAS PubMed.
- F. Wang and X. Liu, Chem. Soc. Rev., 2009, 38, 976–989 RSC.
- J. Zhou, Z. Liu and F. Li, Chem. Soc. Rev., 2012, 41, 1323–1349 RSC.
- J. Shen, L. Zhao and G. Han, Adv. Drug Delivery Rev., 2013, 65, 744–755 CrossRef CAS PubMed.
- G. Chen, H. Qiu, P. N. Prasad and X. Chen, Chem. Rev., 2014, 114, 5161–5214 CrossRef CAS PubMed.
- H. Dong, S.-R. Du, X.-Y. Zheng, G.-M. Lyu, L.-D. Sun, L.-D. Li, P.-Z. Zhang, C. Zhang and C.-H. Yan, Chem. Rev., 2015, 115, 10725–10815 CrossRef CAS PubMed.
- D. Yang, P. a. Ma, Z. Hou, Z. Cheng, C. Li and J. Lin, Chem. Soc. Rev., 2015, 44, 1416–1448 RSC.
- J. Zhou, Q. Liu, W. Feng, Y. Sun and F. Li, Chem. Rev., 2015, 115, 395–465 CrossRef CAS PubMed.
- J. Liu, W. Bu, L. Pan and J. Shi, Angew. Chem., Int. Ed., 2013, 52, 4375–4379 CrossRef CAS PubMed.
- L. Zhao, J. Peng, Q. Huang, C. Li, M. Chen, Y. Sun, Q. Lin, L. Zhu and F. Li, Adv. Funct. Mater., 2014, 24, 363–371 CrossRef CAS.
- Y. Dai, H. Xiao, J. Liu, Q. Yuan, P. a. Ma, D. Yang, C. Li, Z. Cheng, Z. Hou, P. Yang and J. Lin, J. Am. Chem. Soc., 2013, 135, 18920–18929 CrossRef CAS PubMed.
- Y. Min, J. Li, F. Liu, E. K. L. Yeow and B. Xing, Angew. Chem., Int. Ed., 2014, 53, 1012–1016 CrossRef CAS PubMed.
- S. Jiang and Y. Zhang, Langmuir, 2010, 26, 6689–6694 CrossRef CAS PubMed.
- M. K. G. Jayakumar, N. M. Idris and Y. Zhang, Proc. Natl. Acad. Sci. U. S. A., 2012, 109, 8483–8488 CrossRef CAS PubMed.
- Y. Yang, F. Liu, X. Liu and B. Xing, Nanoscale, 2013, 5, 231–238 RSC.
- L. Wang, J. Liu, Y. Dai, Q. Yang, Y. Zhang, P. Yang, Z. Cheng, H. Lian, C. Li, Z. Hou, P. a. Ma and J. Lin, Langmuir, 2014, 30, 13042–13051 CrossRef CAS PubMed.
- J. Li, C. W. T. Leung, D. S. H. Wong, J. Xu, R. Li, Y. Zhao, C. Y. Y. Yung, E. Zhao, B. Z. Tang and L. Bian, ACS Appl. Mater. Interfaces, 2017 DOI:10.1021/acsami.7b00845.
- H. S. Qian, H. C. Guo, P. C.-L. Ho, R. Mahendran and Y. Zhang, Small, 2009, 5, 2285–2290 CrossRef CAS PubMed.
- N. M. Idris, M. K. Gnanasammandhan, J. Zhang, P. C. Ho, R. Mahendran and Y. Zhang, Nat. Med., 2012, 18, 1580–1585 CrossRef CAS PubMed.
- M. K. G. Jayakumar, A. Bansal, K. Huang, R. Yao, B. N. Li and Y. Zhang, ACS Nano, 2014, 8, 4848–4858 CrossRef CAS PubMed.
- X. Wang, K. Liu, G. Yang, L. Cheng, L. He, Y. Liu, Y. Li, L. Guo and Z. Liu, Nanoscale, 2014, 6, 9198–9205 RSC.
- L. Wang, C. Gao, K. Liu, Y. Liu, L. Ma, L. Liu, X. Du and J. Zhou, Adv. Funct. Mater., 2016, 26, 3480–3489 CrossRef CAS.
- X. Wu, Z. Hu, S. Nizzero, G. Zhang, M. R. Ramirez, C. Shi, J. Zhou, M. Ferrari and H. Shen, J. Controlled Release, 2017, 268, 92–101 CrossRef CAS PubMed.
- H. Zeng and G. F. Combs, J. Nutr. Biochem., 2008, 19, 1–7 CrossRef CAS PubMed.
- L. Kamrani Moghaddam, S. Ramezani Paschepari, M. A. Zaimy, A. Abdalaian and A. Jebali, Cancer Gene Ther., 2016, 23, 321–325 CrossRef CAS PubMed.
- Q. Yu, Y. Liu, C. Cao, F. Le, X. Qin, D. Sun and J. Liu, Nanoscale, 2014, 6, 9279–9292 RSC.
- M. S. Draz, B. A. Fang, P. Zhang, Z. Hu, S. Gu, K. C. Weng, J. W. Gray and F. F. Chen, Theranostics, 2014, 4, 872–892 CrossRef CAS PubMed.
- M. Prato, K. Kostarelos and A. Bianco, Acc. Chem. Res., 2008, 41, 60–68 CrossRef CAS PubMed.
- K. K. Jain, Expert Opin. Drug Discovery, 2012, 7, 1029–1037 CrossRef CAS PubMed.
- C. L. Lay, J. Liu and Y. Liu, Expert Rev. Med. Devices, 2011, 8, 561–566 CrossRef CAS PubMed.
- H. Chang and S.-Q. Sun, Chin. Phys. B, 2014, 23, 088102 CrossRef.
- A. Gizzatov, C. Stigliano, J. S. Ananta, R. Sethi, R. Xu, A. Guven, M. Ramirez, H. Shen, A. Sood, M. Ferrari, L. J. Wilson, X. Liu and P. Decuzzi, Cancer Lett., 2014, 352, 97–101 CrossRef CAS PubMed.
- E. Blanco, H. Shen and M. Ferrari, Nat. Biotechnol., 2015, 33, 941–951 CrossRef CAS PubMed.
- H. Shen, T. Sun and M. Ferrari, Cancer Gene Ther., 2012, 19, 367–373 CrossRef CAS PubMed.
- R. Xu, Y. Huang, J. Mai, G. Zhang, X. Guo, X. Xia, E. J. Koay, G. Qin, D. R. Erm, Q. Li, X. Liu, M. Ferrari and H. Shen, Small, 2013, 9, 1799–1808 CrossRef CAS PubMed.
- J. Wolfram, H. Shen and M. Ferrari, J. Controlled Release, 2015, 219, 406–415 CrossRef CAS PubMed.
- H. Shen, C. Rodriguez-Aguayo, R. Xu, V. Gonzalez-Villasana, J. Mai, Y. Huang, G. Zhang, X. Guo, L. Bai, G. Qin, X. Deng, Q. Li, D. R. Erm, B. Aslan, X. Liu, J. Sakamoto, A. Chavez-Reyes, H. D. Han, A. K. Sood, M. Ferrari and G. Lopez-Berestein, Clin. Cancer Res., 2013, 19, 1806–1815 CrossRef CAS PubMed.
- X. Chen, D. Iliopoulos, Q. Zhang, Q. Tang, M. B. Greenblatt, M. Hatziapostolou, E. Lim, W. L. Tam, M. Ni, Y. Chen, J. Mai, H. Shen, D. Z. Hu, S. Adoro, B. Hu, M. Song, C. Tan, M. D. Landis, M. Ferrari, S. J. Shin, M. Brown, J. C. Chang, X. S. Liu and L. H. Glimcher, Nature, 2014, 508, 103–107 CrossRef CAS PubMed.
- D. K. Kirui, E. J. Koay, X. Guo, V. Cristini, H. Shen and M. Ferrari, Nanomedicine, 2014, 10, 1487–1496 CrossRef CAS PubMed.
- J. Mai, Y. Huang, C. Mu, G. Zhang, R. Xu, X. Guo, X. Xia, D. E. Volk, G. L. Lokesh, V. Thiviyanathan, D. G. Gorenstein, X. Liu, M. Ferrari and H. Shen, J. Controlled Release, 2014, 187, 22–29 CrossRef CAS PubMed.
- S. Ma, X. Y. Tian, Y. Zhang, C. Mu, H. Shen, J. Bismuth, H. J. Pownall, Y. Huang and W. T. Wong, Sci. Rep., 2016, 6, 22910 CrossRef CAS PubMed.
- Y. Mi, C. Mu, J. Wolfram, Z. Deng, T. Y. Hu, X. Liu, E. Blanco, H. Shen and M. Ferrari, Adv. Healthcare Mater., 2016, 5, 936–946 CrossRef CAS PubMed.
- M. Zhang, R. Xu, X. Xia, Y. Yang, J. Gu, G. Qin, X. Liu, M. Ferrari and H. Shen, Biomaterials, 2014, 35, 423–431 CrossRef CAS PubMed.
- J. Shen, R. Xu, J. Mai, H. C. Kim, X. Guo, G. Qin, Y. Yang, J. Wolfram, C. Mu, X. Xia, J. Gu, X. Liu, Z. W. Mao, M. Ferrari and H. Shen, ACS Nano, 2013, 7, 9867–9880 CrossRef CAS PubMed.
- D. Tarn, C. E. Ashley, M. Xue, E. C. Carnes, J. I. Zink and C. J. Brinker, Acc. Chem. Res., 2013, 46, 792–801 CrossRef CAS PubMed.
- I. Slowing, J. C. Vivero-Escoto and V. Lin, Adv. Drug Delivery Rev., 2008, 60, 1278–1288 CrossRef CAS PubMed.
- X. Li, Q. R. Xie, J. Zhang, W. Xia and H. Gu, Biomaterials, 2011, 32, 9546–9556 CrossRef CAS PubMed.
- M. Oroval, E. Climent, C. Coll, R. Eritja, A. Aviñó, M. D. Marcos, F. Sancenón, R. Martínez-Máñez and P. Amorós, Chem. Commun., 2013, 49, 5480–5482 RSC.
- A. M. Chen, M. Zhang, D. Wei, D. Stueber, O. Taratula, T. Minko and H. He, Small, 2009, 5, 2673–2677 CrossRef CAS PubMed.
- E. Climent, A. Bernardos, R. Martínez-Máñez, A. Maquieira, M. D. Marcos, N. Pastor-Navarro, R. Puchades, F. Sancenón, J. Soto and P. Amorós, J. Am. Chem. Soc., 2009, 131, 14075–14080 CrossRef CAS PubMed.
- E. Aznar, M. D. Marcos, R. Martínezmáñez, F. Sancenón, J. Soto, P. Amorós and C. Guillem, J. Am. Chem. Soc., 2009, 131, 6833–6843 CrossRef CAS PubMed.
- C. De la Torre, A. Agostini, L. Mondragón, M. Orzáez, F. Sancenón, R. Martínez-Máñez, M. D. Marcos, P. Amorós and E. Pérez-Payá, Chem. Commun., 2014, 50, 3184–3186 RSC.
- V. Mamaeva, C. Sahlgren and M. Lindén, Adv. Drug Delivery Rev., 2013, 65, 689–702 CrossRef CAS PubMed.
- D. R. Radu, C. Y. Lai, K. Jeftinija, E. W. Rowe, S. Jeftinija and V. S. Lin, J. Am. Chem. Soc., 2004, 126, 13216–13217 CrossRef CAS PubMed.
- T. Xia, M. Kovochich, M. Liong, H. Meng, S. Kabehie, S. George, J. I. Zink and A. E. Nel, ACS Nano, 2009, 3, 3273–3286 CrossRef CAS PubMed.
- L. Sun, D. Wang, Y. Chen, L. Wang, P. Huang, Y. Li, Z. Liu, H. Yao and J. Shi, Biomaterials, 2017, 133, 219–228 CrossRef CAS PubMed.
- H. Lu, T. Cui and C. Yin, Biomaterials, 2015, 60, 42–52 CrossRef PubMed.
- J. Shen, H. Liu, C. Mu, J. Wolfram, W. Zhang, H.-C. Kim, G. Zhu, Z. Hu, L.-N. Ji, X. Liu, M. Ferrari, Z.-W. Mao and H. Shen, Nanoscale, 2017, 9, 5329–5341 RSC.
- H. Meng, M. Liong, T. Xia, Z. Li, Z. Ji, J. I. Zink and A. E. Nel, ACS Nano, 2010, 4, 4539–4550 CrossRef CAS PubMed.
- Z. Li, J. C. Barnes, A. Bosoy, J. F. Stoddart and J. I. Zink, Chem. Soc. Rev., 2012, 43, 2590–2605 RSC.
- D. Lin, Q. Cheng, Q. Jiang, Y. Huang, Z. Yang, S. Han, Y. Zhao, S. Guo, Z. Liang and A. Dong, Nanoscale, 2013, 5, 4291–4301 RSC.
- W. Cheng, C. Liang, X. Wang, H. Tsai, G. Liu, Y. Peng, J. Nie, L. Huang, L. Mei and X. Zeng, Nanoscale, 2017, 9, 17063–17073 RSC.
- X. Ma, C. Teh, Q. Zhang, P. Borah, C. Choong, V. Korzh and Y. Zhao, Antioxid. Redox Signaling, 2014, 21, 707–722 CrossRef CAS PubMed.
- B. Darvishi, L. Farahmand and K. Majidzadeh-A, Mol. Ther.–Nucleic Acids, 2017, 7, 164–180 CrossRef CAS PubMed.
- X. Ma, Y. Zhao, K. W. Ng and Y. Zhao, Chem. – Eur. J., 2013, 19, 15593–15603 CrossRef CAS PubMed.
- X. Shi, H. Chang, S. Chen, C. Lai, A. Khademhosseini and H. Wu, Adv. Funct. Mater., 2012, 22, 751–759 CrossRef CAS.
- X. Li, H. Liu, X. Niu, B. Yu, Y. Fan, Q. Feng, F.-z. Cui and F. Watari, Biomaterials, 2012, 33, 4818–4827 CrossRef CAS PubMed.
- K. S. Kim, Y. Zhao, H. Jang, S. Y. Lee, J. M. Kim, K. S. Kim, J.-H. Ahn, P. Kim, J.-Y. Choi and B. H. Hong, Nature, 2009, 457, 706–710 CrossRef CAS PubMed.
- J. M. Yoo, J. H. Kang and B. H. Hong, Chem. Soc. Rev., 2015, 44, 4835–4852 RSC.
- A. De La Zerda, C. Zavaleta, S. Keren, S. Vaithilingam, S. Bodapati, Z. Liu, J. Levi, B. R. Smith, T.-J. Ma, O. Oralkan, Z. Cheng, X. Chen, H. Dai, B. T. Khuri-Yakub and S. S. Gambhir, Nat. Nanotechnol., 2008, 3, 557–562 CrossRef CAS PubMed.
- J. Ge, M. Lan, B. Zhou, W. Liu, L. Guo, H. Wang, Q. Jia, G. Niu, X. Huang, H. Zhou, X. Meng, P. Wang, C.-S. Lee, W. Zhang and X. Han, Nat. Commun., 2014, 5, 4596 CAS.
- S. K. S. Kushwaha, S. Ghoshal, A. K. Rai and S. Singh, Braz. J. Pharm. Sci., 2013, 49, 629–643 CrossRef CAS.
- L. Wang, J. Shi, H. Zhang, H. Li, Y. Gao, Z. Wang, H. Wang, L. Li, C. Zhang, C. Chen, Z. Zhang and Y. Zhang, Biomaterials, 2013, 34, 262–274 CrossRef CAS PubMed.
- F. Zhou, S. Wu, S. Song, W. R. Chen, D. E. Resasco and D. Xing, Biomaterials, 2012, 33, 3235–3242 CrossRef CAS PubMed.
- Y.-F. Wu, H.-C. Wu, C.-H. Kuan, C.-J. Lin, L.-W. Wang, C.-W. Chang and T.-W. Wang, Sci. Rep., 2016, 6, 21170 CrossRef CAS PubMed.
- V. Raffa, G. Ciofani, O. Vittorio, C. Riggio and A. Cuschieri, Nanomedicine, 2010, 5, 89–97 CrossRef CAS PubMed.
-
K. T. Al-Jamal and K. Kostarelos, Carbon Nanotubes: Methods and Protocols, 2010, pp. 123–134 Search PubMed.
- C. Klumpp, K. Kostarelos, M. Prato and A. Bianco, Biochim. Biophys. Acta, Biomembr., 2006, 1758, 404–412 CrossRef CAS PubMed.
- Z. Zhang, X. Yang, Y. Zhang, B. Zeng, S. Wang, T. Zhu, R. B. S. Roden, Y. Chen and R. Yang, Clin. Cancer Res., 2006, 12, 4933 CrossRef CAS PubMed.
- C. Guo, W. T. Al-Jamal, F. M. Toma, A. Bianco, M. Prato, K. T. Al-Jamal and K. Kostarelos, Bioconjugate Chem., 2015, 26, 1370–1379 CrossRef CAS PubMed.
- Z. Liu, M. Winters, M. Holodniy and H. Dai, Angew. Chem., Int. Ed., 2007, 46, 2023–2027 CrossRef CAS PubMed.
- A. K. Geim and K. S. Novoselov, Nat. Mater., 2007, 6, 183–191 CrossRef CAS PubMed.
- X. Li, X. Wang, L. Zhang, S. Lee and H. Dai, Science, 2008, 319, 1229–1232 CrossRef CAS PubMed.
- K. S. Novoselov, A. K. Geim, S. V. Morozov, D. Jiang, Y. Zhang, S. V. Dubonos, I. V. Grigorieva and A. A. Firsov, Science, 2004, 306, 666–669 CrossRef CAS PubMed.
- B. Chen, M. Liu, L. Zhang, J. Huang, J. Yao and Z. Zhang, J. Mater. Chem., 2011, 21, 7736–7741 RSC.
- L. Feng, S. Zhang and Z. Liu, Nanoscale, 2011, 3, 1252–1257 RSC.
- B. J. Hong, O. C. Compton, Z. An, I. Eryazici and S. T. Nguyen, ACS Nano, 2012, 6, 63–73 CrossRef CAS PubMed.
- L. Zhang, Q. Zhou, W. Song, K. Wu, Y. Zhang and Y. Zhao, ACS Appl. Mater. Interfaces, 2017, 9, 34722–34735 CAS.
- L. Ren, Y. Zhang, C. Cui, Y. Bi and X. Ge, RSC Adv., 2017, 7, 20553–20566 RSC.
- L. Zhang, Z. Lu, Q. Zhao, J. Huang, H. Shen and Z. Zhang, Small, 2011, 7, 460–464 CrossRef CAS PubMed.
- S. Ghaderi, B. Ramesh and A. M. Seifalian, J. Drug Targeting, 2011, 19, 475–486 CrossRef CAS PubMed.
- L. Hu, Y. Sun, S. Li, X. Wang, K. Hu, L. Wang, X.-J. Liang and Y. Wu, Carbon, 2014, 67, 508–513 CrossRef CAS.
- Q. Wang, C. Zhang, G. Shen, H. Liu, H. Fu and D. Cui, J. Nanobiotechnol., 2014, 12, 58 CrossRef PubMed.
-
J. Kolosnjaj, H. Szwarc and F. Moussa, Bio-Applications of Nanoparticles, Springer, 2007, pp. 181–204 Search PubMed.
- A. B. Seabra, A. J. Paula, R. de Lima, O. L. Alves and N. Durán, Chem. Res. Toxicol., 2014, 27, 159–168 CrossRef CAS PubMed.
- D. Adams, O. B. Suhr, P. J. Dyck, W. J. Litchy, R. G. Leahy, J. Chen, J. Gollob and T. Coelho, BMC Neurol., 2017, 17, 181 CrossRef PubMed.
Footnote |
† These authors contributed equally to this work. |
|
This journal is © The Royal Society of Chemistry 2018 |