DOI:
10.1039/C7CE01912B
(Paper)
CrystEngComm, 2018,
20, 75-87
Preparation, properties, formation mechanisms, and cytotoxicity of calcium oxalate monohydrate with various morphologies
Received
3rd November 2017
, Accepted 17th November 2017
First published on 17th November 2017
Abstract
Calcium oxalate monohydrate (COM) crystals with various morphologies, such as elliptical, hexagonal, peanut-like, spherical and flower-like structures with a size of about 10 μm, were prepared through varying the reactant concentration, stirring speed, reaction temperature, and additive. X-ray diffraction and infrared spectroscopy showed that the synthesized crystals were pure-phase COM. The specific surface area and pore structure analysis demonstrated that all these COM crystals contained pores. Moreover, the zeta potential of each COM morphology was negative, and the value positively corresponded to the negative charge density of the crystal surface. Cell viability, lactate dehydrogenase (LDH) release, and reactive oxygen species (ROS) level assays suggested that these COM crystals caused injury to African green monkey renal epithelial (Vero) cells. Such an ability of the crystals to cause injury was correlated with the Ca2+ ion-rich (
01) crystal plane area, crystal edge sharpness, zeta potential and crystal-specific surface area. Hence, the cytotoxicity of the COM crystals was closely related to their morphology and surface structure.
1. Introduction
The treatment of urinary tract calculi has gained considerable progress in recent years with the extensive application of endoscopic and extracorporeal lithotripsy and minimally invasive techniques; however, avoiding recurrence and preventing stone formation are difficult, and the recurrence rate has remained high at 75% over the last 20 years.1
Calcium oxalate, which mainly includes calcium oxalate monohydrate (COM) and calcium oxalate dehydrate (COD), is the main inorganic component of kidney stones, of which COM is the most common constituent of calcium oxalate stones.2 Recently, research on crystallization of COM has attracted interest because COM is most frequently observed in the majority of renal stones in the urinary system.3,4 The occurrence of COM calculi is higher in the urine of recurrent stone formers compared with that in healthy urine. Therefore, COM crystals produce a toxic effect on cells, injure the cell membrane, and easily adhere to the cell surface. Stone formation has focused largely on the role of modulators, which may act as promoters or inhibitors,5 and the lack of inhibitory activity or viability reduction in urine is one of the key factors. Several inhibitors in urine combine with calcium or oxalate ions, reduce the calcium oxalate crystal nucleation, decrease the supersaturation of calcium oxalate in urine, cause the blunt edges and corners of crystals, reduce the adhesion to renal tubular cells, decrease the calcium oxalate crystal size, which can be stably dispersed in urine and decrease the aggregation degree, and inhibit stone formation in urine.
Both healthy people and stone former patients contain calcium oxalate crystals in their urine.2 Nevertheless, the urine of normal people has more types of inhibitors with higher concentration and stronger activity compared with that of stone-forming patients,6,7 thus resulting in a large difference in the crystal morphology and size in the urine of normal people and stone formers. Furthermore, crystal particles in normal human urine are almost spherical or elliptical, whereas those in the urine of stone-formers have sharp corners and edges,8 which have higher affinity to adhere to renal tubular epithelial cells and cause physical damage and inflammation. Hence, urinary crystallites with different morphologies can influence the renal epithelial cell injury and crystal–cell adhesion, finally affecting stone formation.9 The differences in the morphology and surface structure of crystals affect their cytotoxicity. Huang et al.10 reported that mesoporous silica nanoparticles with large aspect ratio were taken up in large amounts and have fast internalization rates in A375 human melanoma cells. Similarly, particles with large aspect ratio have considerable impact on different aspects of cellular functions, including cell proliferation, apoptosis, cytoskeleton formation, adhesion, and migration.
Several factors can modify the morphology of calcium oxalate crystals.11 However, the relationship between various additives and morphological modification of CaOx crystals is not well understood. Accordingly, in the present study, the crystal morphology of COM was modified via varying the reactant concentration, reaction temperature, stirring speed, and additive. Moreover, the toxicity of COM with various morphologies to renal epithelial cells was studied to provide knowledge on the modulation of COM crystals and formation mechanism of renal stones.
2. Materials and methods
2.1 Reagents and apparatus
(1) Materials.
Calcium chloride (CaCl2), sodium oxalate (Na2Ox), potassium oxalate (K2Ox), Na3Cit (sodium citrate), gelatin, ethylenediaminetetraacetic acid (Na2EDTA), sodium chloride (NaCl) and the other conventional reagents used were analytically pure and purchased from Guangzhou Chemical Reagent Factory of China (Guangzhou, China). African green monkey renal epithelial (Vero) cells were purchased from Shanghai Cell Bank, Chinese Academy of Sciences (Shanghai, China). Dulbecco's modified Eagle's medium (DMEM) was purchased from HyClone Biochemical Products Co., Ltd. (UT, USA). Fetal bovine serum was purchased from Hangzhou Sijiqing Biological Engineering Materials Co., Ltd. (Hangzhou, China). Penicillin and streptomycin were purchased from Beijing Pubo Biotechnology Co., Ltd. (Beijing, China). Cell Counting Kit-8 (CCK-8) was purchased from Dojindo Laboratories (Kumamoto, Japan). Lactate dehydrogenase (LDH) kit and 2′,7′-dichlorofluorescein diacetate dye (DCFH-DA) were purchased from Shanghai Beyotime Bio-Tech Co., Ltd. (Shanghai, China). Cell culture plates were purchased from Wuxi Nest Bio-Tech Co., Ltd. (Wuxi, China).
(2) Apparatus.
The apparatus included an XL type environmental scanning electron microscope (SEM, Philips, Eindhoven, Netherlands), an ULTRA 55 field emission scanning electron microscope (Zeiss company, Germany), a Nano-ZS nanoparticle sizer (Malvem, UK), a D/max2400X X-ray powder diffractometer (Rigaku, Japan), a Fourier transform infrared spectrometer (Nicolet, American), and a Tristar 3000 surface area and porosity analyzer (Micromeritics, American).
2.2 Preparation of COM crystals with various morphologies
1) COM-elliptical.
500 mL of Na2Ox (10 mM) solution and 1.176 g of Na3Cit were added into a 2000 mL beaker, evenly stirred, and heated to 75 °C. Afterward, 500 mL of CaCl2 (5 mM) solution preheated to 75 °C was added to the reaction mixture, and the reaction was maintained at 75 °C for 30 min under static conditions. The final solution was incubated overnight at room temperature and subsequently washed three times with distilled water and anhydrous ethanol under ultrasonication. Crystals were collected via suction filtration and dried in a drying oven for 24 h. Finally, elliptical COM crystals were obtained.
2) COM-hexagonal.
0.2 g of gelatin was added to 20 mL of distilled water and heated to dissolve completely. Impurities in the gelatin were removed via centrifugation using 400 mL of 0.05% (w/v) of the gelatin solution and heated to 75 °C. In addition, about 20 mL of K2Ox (20 mM) solution was added and stirred at 300 rpm for 10 min. Afterward, 20 mL of CaCl2 (20 mM) solution was added, and the stirring speed and reaction temperature were maintained for 10 min. The prepared crystals were collected with the same processing as above.
3) COM-peanut-like.
A total of 200 mL of CaCl2 (40 mM) and 100 mL of ethylenediaminetetraacetic acid (Na2EDTA, 10 mM) were mixed in a 500 mL beaker, stirred to form Ca-EDTA ions, and heated to 65 °C. Subsequently, 100 mL of Na2Ox (10 mM) solution preheated to 65 °C was added, and the final solution was incubated overnight at room temperature. The prepared crystals were collected with the same processing as the synthesis of COM-elliptical.
4) COM-spherical.
A total of 150 mL of Na2Ox (10 mM), 150 mL of NaCl (0.5 M) and 0.35 g Na3Cit were mixed in a 500 mL beaker, evenly stirred, and heated to 70 °C. Afterward, 150 mL of CaCl2 (5 mM) solution preheated to 75 °C was added to the reaction mixture, and the reaction was maintained at 70 °C under continuous stirring at 300 rpm for 2 min. The final solution was incubated overnight at room temperature. The prepared crystals were collected with the same processing as the synthesis of COM-elliptical.
5) COM-flower-like.
A total of 150 mL of Na2Ox (10 mM) and 150 mL of NaCl (0.5 M) were mixed in a 500 mL beaker, evenly stirred, and heated to 70 °C. Afterward, 150 mL of CaCl2 (5 mM) solution preheated to 70 °C was added to the reaction mixture, and the reaction was maintained at 70 °C under continuous stirring at 900 rpm for 2 min. The final solution was incubated overnight at room temperature. The prepared crystals were collected with the same processing as the synthesis of COM-elliptical.
2.3 Characterization of COM crystals with various morphologies
The morphology and structure of COM of various morphologies were observed with an XL type environmental scanning electron microscope (SEM) operated at 30 kV. The X-ray powder diffraction (XRD) patterns of the products were recorded with Cu-Kα radiation at a scanning rate of 8° per min in the 2θ range from 5° to 55°. Fourier-transform infrared spectroscopy (FT-IR) analysis was conducted with KBr discs in the region of 4000 cm−1 to 400 cm−1 with a resolution of 4 cm−1.
2.4 Specific surface area measurement of COM crystals
The COM crystals with various morphologies were degassed at 80 °C. Subsequently, adsorption experiments were conducted at −196 °C using an N2 adsorbent. The specific surface area (SBET) of the crystals was calculated using the Brunauer–Emmett–Teller (BET) equation. The pore-size distribution curves, pore volume, and average pore diameter were determined using the Barrett–Joyner–Halenda method.
2.5 Zeta potential and conductivity detection of COM crystals
Appropriate amounts of COM crystals of various morphologies were dispersed in distilled water or culture medium until the final crystal concentration was 0.2 mg mL−1. After 5 min of ultrasonication, the zeta potential and conductivity were detected using Zetasizer Nano ZS90 apparatus at 25 °C.
2.6 Detection of cytotoxicity of COM crystals of various morphologies using CCK-8 assay
African green monkey renal epithelial (Vero) cells were cultured in DMEM culture medium containing 10% fetal bovine serum and 100 U ml−1 penicillin–100 μg ml−1 streptomycin antibiotics at 37 °C in a 5% CO2 humidified environment. Upon reaching an 80–90% confluent monolayer, cells were trypsinized to form a cell suspension for the following cell experiment.
100 μL of cell suspension with a cell concentration of 1.0 × 105 cells per mL was inoculated per well in 96-well plates and incubated for 24 h. The culture medium was removed by suction and the cells were washed twice with PBS. The experimental model was divided into two groups: (A) control group: only serum-free culture medium was added and (B) treatment group: Vero cells were exposed to five shapes of COM crystals suspension (800 μg mL−1) in serum-free culture medium. Each experiment was repeated in five parallel wells. After incubation for 6 h, 10 μL CCK-8 was added to each well and incubated for 1.5 h. Absorbance (A) was measured using an enzyme mark instrument at 450 nm. Cell viability was determined using the equation below.
2.7 Lactate dehydrogenase (LDH) release assay
100 μL of cell suspension with a cell concentration of 1 × 105 cell per mL was inoculated per well and incubated for 24 h. Afterward, the medium was changed to serum-free culture medium and then incubated for another 12 h to achieve synchronization. The experimental model was divided into four groups: (A) cell-free culture medium wells (control wells of background), (B) control wells without drug treatment (sample control wells), (C) cells without drug treatment for the subsequent cleavage of the wells (sample maximum enzyme activity control wells), and (D) the treated group with five shapes of COM crystals suspension (800 μg mL−1) in serum-free culture medium (drug-treated wells). After incubation, the absorbance was analyzed at 490 nm according to the LDH kit instruction.
2.8 Detection of reactive oxygen species (ROS) generation
2 mL of cell suspension with a cell concentration of 1.0 × 105 cells per mL was inoculated per well in 12-well plates and incubated for 24 h. The culture medium was removed by suction and the cells were washed twice with PBS. After the exposure of cells to the five shapes of COM crystal suspension (800 μg mL−1) in serum-free culture medium for 6 h, the samples were then stained with 2′,7′-dichloro-fluorescein diacetate (DCFH-DA). ROS distribution was observed under a fluorescence microscope.
For quantitative analysis, the cells were inoculated in 96-well plates with a concentration of 1 × 105 cells per mL and 100 μL per well, and the cells are treated the same as above. The fluorescence intensity of intracellular ROS was quantitatively detected by microplate reader.
2.9 Statistical analysis
Statistical analyses were performed using the SPSS 13.0 software. Data were expressed as mean ± SD. Multiple group comparisons were performed using one-way ANOVA, followed by the Tukey post hoc test. If p < 0.05, there was a significant difference, if p < 0.01, the difference was extremely significant and if p > 0.05, there was no significant difference.
3. Results and discussion
3.1 Synthesis of the different morphologies of COM
Fig. 1 shows the SEM images of the COM crystals with various morphologies and sizes of about 10 μm. The hexagonal COM was a (
01) face elongated crystal. The main growth of the crystal was the (
01) face, whereas the (010) face growth was inhibited (Fig. 1a and 2). The elliptical COM crystal was smooth and flake-like, and presented a wide (
01) face and a thin (100) face (Fig. 1b and 3). The surface of peanut-like COM was rough, and a depression was observed in the middle part of the crystal (Fig. 1c). Both spherical aggregate and flower-like COM crystals were formed via aggregation of COM of a single different morphology. Thin hexagonal crystals assembled to the center and exhibited a spherical structure (Fig. 1d). The hexagonal crystal extended from the center to the periphery, and thereby the whole crystal exhibited a flower-like morphology, which had sharp edges and corners (Fig. 1e).
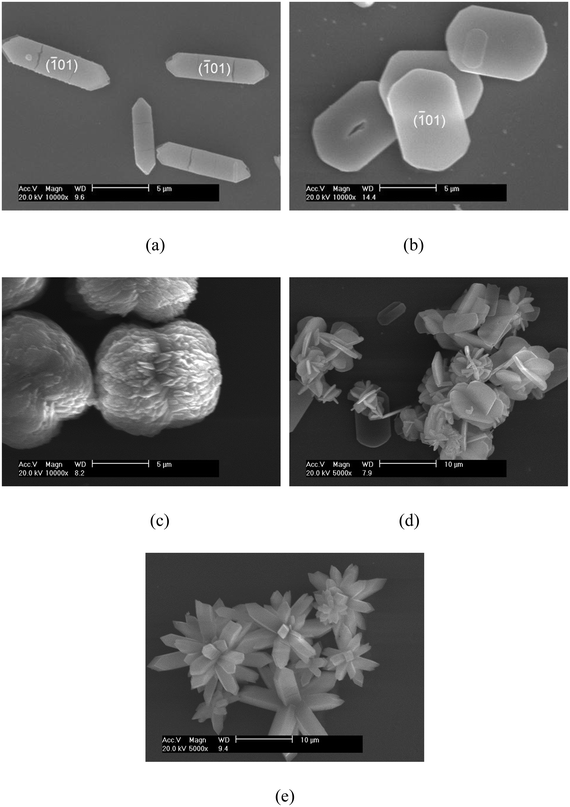 |
| Fig. 1 SEM images of the COM crystals with different morphologies: (a) COM-hexagonal, (b) COM-elliptical, (c) COM-peanut-like, (d) COM-spherical, and (e) COM-flower-like. | |
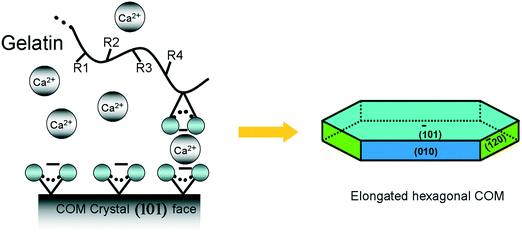 |
| Fig. 2 Schematic diagram of gelatin promoting the growth of the ( 01) face of COM crystals. : –COOH. | |
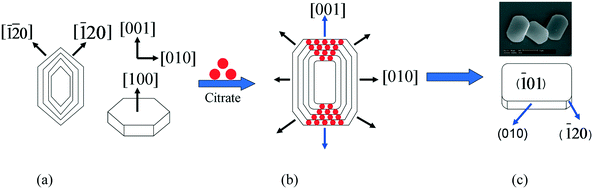 |
| Fig. 3 Schematic diagram of the formation of an elliptical COM crystal: (a) hexagonal COM crystal, (b) effect of Na3cit on ( 01) face of COM, and (c) elliptical COM | |
Various synthesis conditions for the different morphologies of COM crystals are summarized in Table 1. Crystal morphologies were apparently different when various additives were used. Gelatin was utilized as an additive to crystallize (
01) face elongated hexagonal COM. Elliptical COM was obtained through the addition of sodium citrate. Employing the Na2EDTA additive resulted in the peanut-like COM with a rough surface, whereas employing Na3Cit and NaCl caused the formation of spherical aggregates. Furthermore, only the NaCl addition could prepare flower-like aggregates.
Table 1 Experimental conditions for the preparation of COM crystal with different morphologies
|
c(Ca2+)/mmol L−1 |
c/(Ox2−)/mmol L−1 |
Reaction temperature/°C |
Additive |
Stirring speed/rpm |
COM-hexagonal |
20 |
20 |
75 |
Gelatin |
300 |
COM-elliptical |
5 |
10 |
75 |
Na3cit |
0 |
COM-peanut-like |
40 |
10 |
65 |
Na2EDTA |
0 |
COM-spherical |
5 |
10 |
70 |
Na3cit + NaCl |
300 |
COM-flower-like |
5 |
10 |
70 |
NaCl |
900 |
1) Effect of gelatin on COM morphology.
Hexagonal COM could be obtained through gelatin addition. Gelatin is a linear macromolecule that is composed of different amino acids via peptide bonds; this molecule also contains a large number of –COOH and –NH2 groups in its side chain, which can serve as nucleation sites for crystal formation.12Fig. 2 indicates that the negatively charged anionic groups (carboxyl) of gelatin were chelated with the Ca2+ ions of the crystal surface and formed the calcium bridges COO–… Ca2+… –OOC (inhibitor), which interacted with the surface of the COM crystal.13 The polarity and charge density of the (
01) face of COM crystals were matched with the electrostatic field of the gelatin template; thus the growth of the positively charged (
01) face was promoted, and the (010) face of COM was inhibited.
2) Effect of citrate on COM morphology.
Elliptical COM crystals were obtained using a Na3cit additive. Citrate can form highly soluble calcium citrate with the surface Ca2+ of the COM crystal, which is difficult to dissociate.14 The step of the (
01) crystal face of COM had three directions, namely, [001], [
20], and [![[1 with combining macron]](https://www.rsc.org/images/entities/char_0031_0304.gif)
0], which can be modified by citrate from three different directions (Fig. 3a). Additionally, the inhibition degree of the [001] direction was significantly larger than that of the two other directions (Fig. 3b). Consequently, the crystal growth along the [001] direction was inhibited, the length of COM in the [001] direction was reduced, and the edges and corners became blunt because of the strong chelation of Na3cit, which showed a quasi-rectangular elliptic morphology (Fig. 3c).13
3) Effect of Na2EDTA on COM morphology.
The peanut-like COM crystals were obtained through the addition of the Na2EDTA additive in the crystallization process. Two carboxylates of Na2EDTA were chelated with Ca2+. This Ca2+ was wrapped in the interior of the molecule and formed CaEDTA (stability constant, K = 1011.0). After the addition of Na2Ox, Ca2+ ions were slowly released, which retarded the reaction. Simultaneously, Na2EDTA also inhibited the growth of the (
01) crystal plane of COM along the [001] direction, hence making it dull. Under hydrothermal conditions, the reduction of surface energy is the main driving force of crystal growth and morphology evolution.13,15 In the initial nucleation stage, Ca2+ and Ox2− were mixed to form a large number of high-surface energy nanoparticles (Fig. 4a). To reduce the surface energy, spherical nanoparticles grew and aggregated to form large microcrystals (Fig. 4b). The nanoparticles were attached and deposited along both ends to the middle of the microcrystal because of the structural symmetry of the crystals. Ultimately, a peanut-like COM crystal was formed (Fig. 4c). The surface of the peanut-like COM was rough (Fig. 1c).
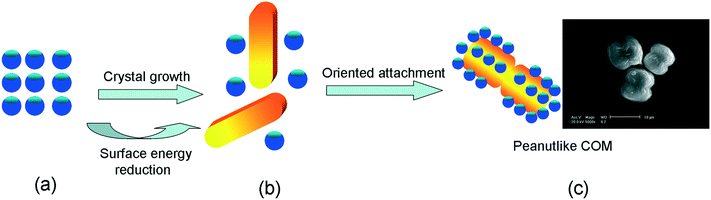 |
| Fig. 4 Schematic diagram of the formation of peanut-like COM crystals: (a) COM nanoparticle, (b) COM micron crystal, and (c) peanut-like COM crystal. | |
4) Effect of NaCl on COM morphology.
During the crystallization process of calcium oxalate, the ionic strength of the solution was increased through NaCl addition, which promoted the aggregation of calcium oxalate. When NaCl was added in distilled water, flower-like aggregates were formed via the aggregation of hexagonal COM (Fig. 1e). When Na3cit and NaCl were added in the preparation process, spherical aggregates (Fig. 1d) were obtained through the aggregation of elliptical COM.
The schematic diagram of the growth mechanism of spherical and flower-like COMs is illustrated in Fig. 5. When the CaCl2 solution was mixed with the Na2Ox solution, a large amount of small calcium oxalate particles was formed. Afterward, these small particles gradually grew. When the crystals grew along the [001] direction, the hexagonal crystals began to appear, and the other hexagonal structure of the microframe was extended from the center and first formed the hexagonal plane, which subsequently formed the embryo of the rose petals and further grew as a template.16,17 In addition, many hexagonal crystals developed via self-assembly and formed flower-like aggregate structures.
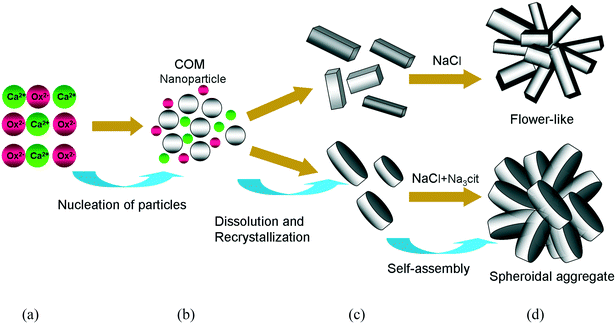 |
| Fig. 5 Schematic diagram of the formation of spherical and flower-like COM crystals: (a) Ca2+ and Ox2− ions in solution, (b) COM nanoparticles, (c) COM microparticles, and (d) spherical and flower-like COM. | |
5) Effect of other factors on COM morphology.
Stirring speed. Fast stirring speed generated crystals with rough surfaces and sharp edges, whereas stationary conditions were conducive to crystal formation with blunt edges and smooth surfaces. For example, 0 rpm stirring speed produced peanut-like and elliptical COMs, which had rounded corners and no obvious edges. Nevertheless, 300 and 900 rpm stirring speed resulted in hexagonal and flower-like COMs, respectively, thus indicating that physical forces affected the crystal morphology.
Reactant concentration. High Ca2+ concentration was beneficial to increasing the thickness of the crystal. When the Ca2+ concentration was 40 and 20 mM, hexagonal and peanut-like COM crystals, which had high thickness, were generated, respectively. Furthermore, the 5 mM Ca2+ concentration produced elliptical COM, which was composed of thin crystals.
3.2 XRD patterns of the different morphologies of COM
In the XRD patterns of the COM crystals with various morphologies (Fig. 6A), the diffraction peaks of all the COM crystals were detected at d = 0.593, 0.365, 0.296, 0.249, 0.235, 0.227, 0.207 and 0.197 nm, which were assigned to the (
01), (020), (
02), (112), (130), (202), (321) and (
03) planes of COM crystals (PDF card number: 20-231), respectively.18
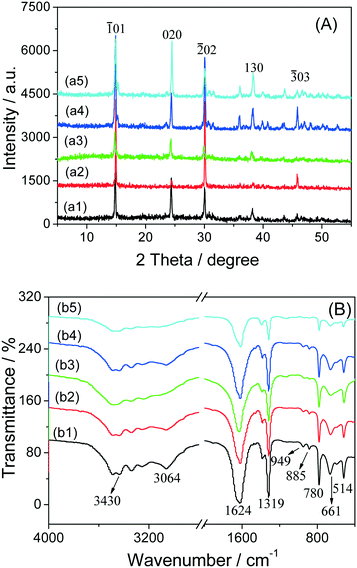 |
| Fig. 6 XRD patterns (A) and FT-IR spectra (B) of the COM crystals with different morphologies: (a1 and b1) COM-hexagonal, (a2 and b2) COM-elliptical, (a3 and b3) COM-peanut-like, (a4 and b4) COM-spherical, and (a5 and b5) COM-flower-like. | |
The intensity ratio of the (
01) and (010) planes of COM with varying morphologies followed this order: COMelliptical > COMspherical > COMpeanut-like > COMhexagonal > COMflower-like (Table 2). The differences in the intensity ratio of the crystal plane can be explained as follows. When gelatin, Na3cit, and Na2EDTA were added in the synthesis process of hexagon, elliptical, and peanut-like COMs, respectively, negatively charged molecules of the additives were preferentially adsorbed on the COM positively charged (
01) plane. Consequently, the crystal plane was stabilized, and the growth of the (010) crystal plane was inhibited, which decreased the area of the (010) plane. The inhibitory effect of these three kinds of additives on the COM (010) plane was in the following order: Na3cit > Na2EDTA > gelatin. Spherical COM was assembled via massive elliptical COM, despite the crystal plane strength ratio of spherical COM being smaller than that of elliptical COM. This finding may be caused by the fact that the crystal aggregates would cover part of the crystal face, hence making its crystal face area smaller than that of the corresponding monocrystal. The flower-like COM was formed through the aggregation of single hexagonal COM, and the change in the strength ratio of the crystal face was similar to that of spherical COM.
Table 2 Specific surface area, (
01)/(010) crystal face ratio, pore size, pore volume, zeta potential, conductivity and dissolved oxalate concentration of COM with various morphologies
Crystal morphology |
Crystal size/μm |
Specific surface area SBET/m2 g−1 |
( 01)/(010) |
Pore size/nm |
Pore volume mm3/g |
Zeta potential in water/mV |
Zeta potential in DMEM medium/mV |
Conductivity μS cm−1 |
Dissolved oxalate concentration/10−4 mol L−1 |
COM-hexagonal |
9.5 ± 1.8 |
1.62 |
1.33 |
6.21 |
1.35 |
−25.5 ± 2.9 |
−9.93 ± 1.34 |
40.3 ± 0.33 |
2.31 |
COM-elliptical |
9.4 ± 1.1 |
1.15 |
3.83 |
4.93 |
2.30 |
−20.3 ± 1.8 |
−6.35 ± 0.92 |
43.2 ± 7.35 |
2.49 |
COM-peanut-like |
10.1 ± 0.7 |
1.18 |
1.50 |
5.39 |
5.76 |
−18.5 ± 2.1 |
−4.59 ± 0.33 |
23.7 ± 2.15 |
1.32 |
COM-spherical |
9.3 ± 1.2 |
2.54 |
2.52 |
10.84 |
7.04 |
−17.0 ± 1.5 |
−6.24 ± 0.92 |
25.3 ± 3.57 |
1.42 |
COM-flower-like |
10.2 ± 1.8 |
1.22 |
1.09 |
5.50 |
1.20 |
−13.9 ± 6.0 |
−5.91 ± 0.47 |
27.4 ± 3.92 |
1.54 |
3.3 FT-IR spectra of the different morphologies of COM
The FT-IR spectra of the COM crystals in various morphologies are shown in Fig. 6B. All the COM crystals had a broad band, which corresponds to the symmetric and asymmetric stretching vibration peaks of the O–H bond of crystal water at 3491–3058 cm−1, and split into 5 absorption peaks, which were the characteristic peaks of COM.19 The asymmetric (νas) and symmetric stretching vibrations (νs) of the carboxyl group (–COO) in COM were at about 1620 cm−1 and 1321 cm−1, respectively.20 In the fingerprint region, the absorption peaks of COM occurred at about 947 (C–O stretching vibration), 885, 785 (C–C stretching vibration), 663 and 514 cm−1 (O–C–O plane bending vibration).
The results of FT-IR and XRD analysis revealed that all prepared COM were pure target products.
3.4 Specific surface area and pore structure analysis of COM with various morphologies
The N2 adsorption–desorption curves of the COM crystals in various morphologies are shown in Fig. 7. In the high-pressure part (p/p0 > 0.85), the nitrogen adsorption quantity increased sharply, indicating that a certain pore structure existed in the crystal. The nitrogen adsorption quantity of spherical COM had the biggest increase, probably due to the spherical aggregate formed by the accumulation of many elliptical COMs, which had a large gap and pore diameter (Table 2).
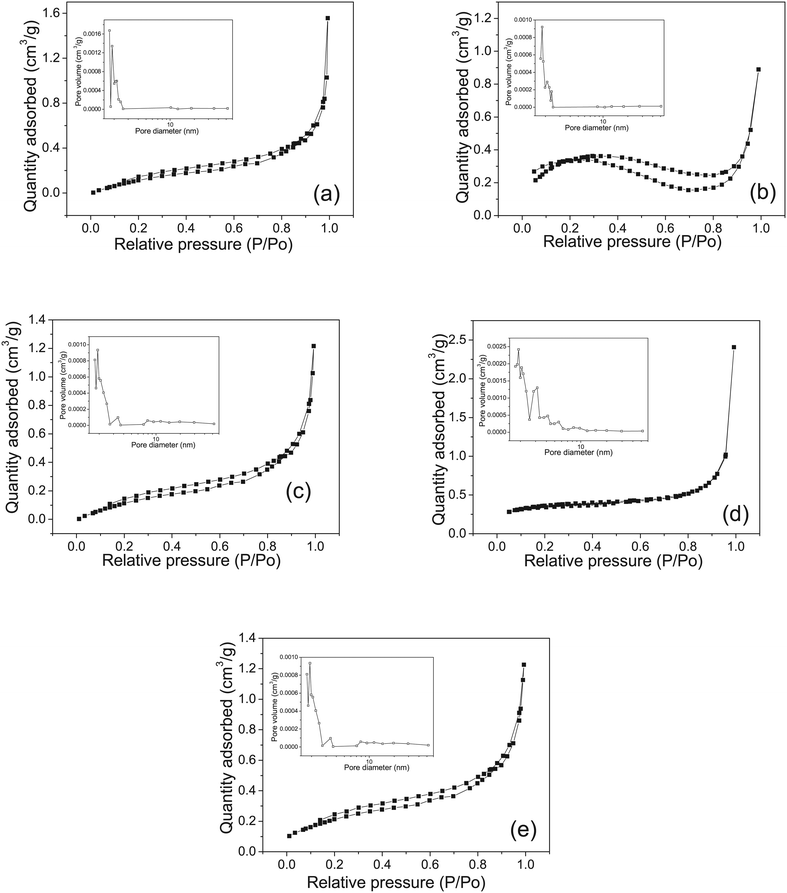 |
| Fig. 7 N2 adsorption–desorption curves and pore size distribution curves (illustration) of COM crystals with varying morphologies: (a) COM-hexagonal, (b) COM-elliptical, (c) COM-peanut-like, (d) COM-spherical, and (e) COM-flower-like. | |
The specific surface area (SBET), pore size, and pore volume of COM with different morphologies are listed in Table 2. Spherical aggregates had the largest specific surface area, which had a great relationship with its own morphology. Spherical aggregates were formed via a series of nucleation, growth, and self-assembly of multiple elliptical COMs, and had the largest pore volume (7.04 mm3 g−1) compared with the other four kinds of crystals. The specific surface area was positively correlated with the pore volume.
3.5 Zeta potential and conductivity of COM crystals with various morphologies
The zeta potential of COM with various morphologies in pure water and cell culture medium is shown in Table 2. The surface of the COM crystals with different morphologies had negative charges, and all the zeta potentials in pure water and culture medium were negative. The absolute value of the zeta potential of COMhexagonal in culture medium was the largest (−25.5), and that of COMflower-like was the least (−13.9 mV). The zeta potential is often affected by the additives during the crystal preparation process or the ions in dispersed medium. When the prepared COM crystals are dispersed in aqueous solution or DMEM, the surface of the crystal, especially the Ca2+ ion-rich face, is easily adsorbed by the residual anionic additives (Gelatin, Na3cit and Na2EDTA) during the preparation process and the anions in the medium, thus resulting in a negative zeta potential on the crystal surface. For example, for the COM-flower-like crystal, no organic additives were added during the crystal preparation process, its zeta potential has a less negative value. Barhoum et al.21 modified calcium carbonate particles with cationic cetyltrimethylammonium bromide (CTAB) and anion sodium oleate surfactants; the zeta potentials of the prepared samples are changed from −15.6 to −0.5 and −22 mV, respectively. The results indicate that the surface charge of calcium carbonate decreases to more negative values with the addition of 2 wt% sodium oleate and increases to less negative values with the addition of 2 wt% CTAB compared to the unmodified samples.
The absolute value of the zeta potential of each COM morphology in culture medium was lower than that of the crystal in pure water. The DMEM culture medium is composed of high quantities of inorganic ions (Ca2+ and Mg2+), amino acids, and vitamins. The exposed Ox2− on the surface of the COM crystals will strongly interact with the Ca2+ and/or Mg2+ ions in the culture medium, leading to the neutralization of some Ox2− anions. This will decrease the absolute values of the zeta potential of the COM crystals in culture medium.
The charge density of the crystal surface was the key factor affecting the zeta potential. The higher negative charge density resulted in a more negative zeta potential. The densities of oxalate with negative charges in the (
01) and (010) planes were (
01) (0.0542 site per A2) and (010) (0.0333 site per A2), respectively.22 Gelatin showed the weakest inhibition effect on the COM (010) plane, and therefore, hexagonal COM showed the larger (010) plane with a large amount of negatively charged oxalates. Hence, the hexagonal COM had the largest zeta potential when dispersed in aqueous solution.
The conductivity values were 40.3, 43.2, 23.7, 25.3, and 27.4 μS cm−1 for COM-hexagonal, COM-elliptical, COM-peanut-like, COM-spherical, and COM-flower-like, respectively. Using these conductivity values, we can calculate the concentration of the released Ox2− ions for the five morphologies of crystals. These concentrations ranged from 0.132 to 0.249 mmol L−1 (Table 2).
3.6 Cellular toxicity of COM crystals with various morphologies
Cell viability results using CCK-8 assay (Fig. 8a) revealed that Vero cells were injured in varying degrees after the exposure to various morphologies of COM for 6 h, which thereby resulted in the decrease of cell viability. Elliptical and flower-like COM had large decrease of cell viability at 84.49% and 86.61%, respectively.
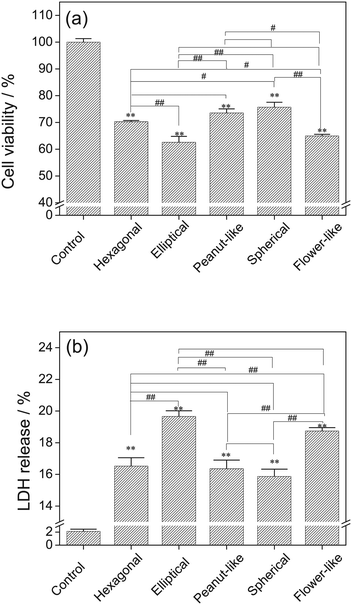 |
| Fig. 8 Cell viability detection by CCK-8 assay (a) and lactate dehydrogenase (LDH) release assay of Vero cells after exposure to 800 μg mL−1 COM with different morphologies for 6 h. Compared with the control group, *p < 0.05, **p < 0.01. Compared with other treated groups, #p < 0.05, ##p < 0.01. | |
Lactate dehydrogenase (LDH) is a stable enzyme of cytoplasm that is released extracellularly when the cell membrane ruptures. Therefore, the released amount of LDH can be used as a marker of cell membrane integrity. The released amount of LDH of Vero cells was changed after the exposure to COM with different morphologies for 6 h, as shown in Fig. 8b. The released amount for the elliptical COM-treated group was the highest (19.66%), followed by that of flower-like COM (18.74%). Spherical COM had the lowest (15.87%) released amount.
Reactive oxygen species (ROS) levels, which can be elevated by pathological and physiological stimuli, indicate the degree of oxidative stress inside the cell. Oxidative stress occurs when the generation of ROS overwhelms the natural antioxidant defenses of cells. The ROS produced using the COM treated cells were measured with a DCFH-DA fluorescence probe. The amount of generated ROS of Vero cells was changed after the exposure to COM with different morphologies for 6 h, as shown in Fig. 9. Compared with the ROS level in the control group, the ROS levels in the five treated groups increased to different degrees. The amounts of generated ROS in five COM-treated groups were ranked as COM-elliptical > COM-flower-like > COM-hexagonal > COM-peanut-like > COM-spherical (Fig. 9). The amount of generated ROS for the elliptical COM-treated group was the highest (50
390 a.u.); spherical COM resulted in the lowest generated amount (35
290 a.u.).
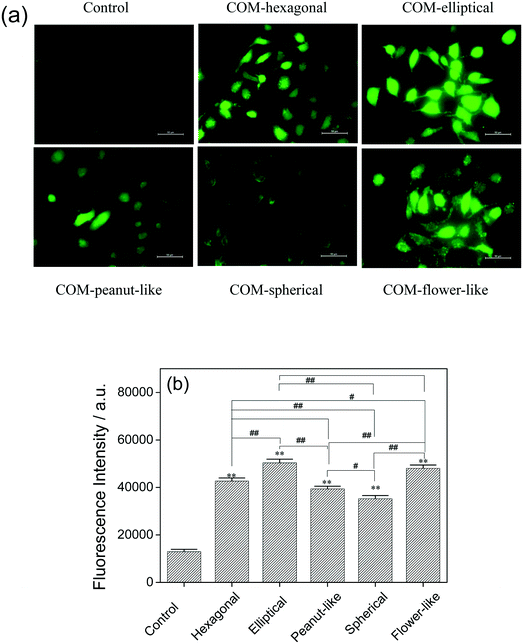 |
| Fig. 9 ROS level assay of Vero cells after exposure to 800 μg mL−1 COM with different morphologies for 6 h. ROS distribution was observed under a fluorescence microscope (a); the fluorescence intensity of intracellular ROS was quantitatively measured using a microplate reader (b). Compared with the control group, *p < 0.05, **p < 0.01. Compared with other treated groups, #p < 0.05, ##p < 0.01. | |
The effect of the physical parameter of the COM crystals and their cytotoxicity is summarized and ranked in Table 3. The cytotoxicity of the different morphologies of crystals was mainly caused by the following:
Table 3 Effect of the physical parameter of COM crystals on crystal cytotoxicity
Crystal morphology |
( 01) face area |
Crystal sharpness |
Specific surface area |
Zeta potential |
Cytotoxicity |
1 represents the maximum cytotoxicity, and 5 represents the least cytotoxicity.
|
COM-hexagonal |
4 |
2 |
2 |
5 |
3 |
COM-elliptical |
1 |
5 |
5 |
4 |
1 |
COM-peanut-like |
3 |
4 |
4 |
1 |
4 |
COM-spherical |
2 |
3 |
1 |
3 |
5 |
COM-flower-like |
5 |
1 |
3 |
2 |
2 |
1) The larger Ca2+ ion-rich (
01) crystal plane area results in more easily damaged integrity of cell membrane, hence resulting in cell injury. The Ca2+ ion density of the (
01) crystal plane (0.0542 Ca2+/A2) was about 63% higher than that of the (010) crystal plane (0.0333 Ca2+/A2);22 thus, the (
01) crystal plane had a stronger interaction with the cell surface. Sheng et al.23 proved that the adhesion force between the COOH-modified AFM tip and the (
01) crystal plane is fourfold higher than that of the (010) crystal plane. Fig. 1 and Table 2 show that elliptical COM had the largest (
01) crystal plane, and the crystal face ratio of I
01/I010 was 3.83. Therefore, the elliptical COM-treated group showed the lowest cell viability and the highest LDH released amount.
2) Sharper crystal edges cause more injury to the cell membrane. The flower-like COM has sharp edges and corners, which resulted in larger injury to the cell membrane. However, peanut-like COM showed less toxicity on the Vero cell, in which the reason may be that Na2EDTA inhibited the growth of the (
01) face, and the crystals became blunt and had no obvious edges and corners (Fig. 1c).
3) The zeta potential values of crystals with five morphologies in DMEM were all negative, and all the values ranged from −4 to −9. No obvious variation in the zeta potential was observed among the five crystals in DMEM. The surface of most cell types was negatively charged; thus lower negative zeta potential of crystals will increase cell–crystal interaction.
4) Crystals with larger specific surface area generally have larger contact area between the crystal and the cell surface, thus easily causing serious damage to the cells. Hexagonal COM has a larger specific surface area, thereby showing higher toxicity to Vero cells. The effect of the specific surface area on the toxicity of the micron-sized crystals is not as great as that of the nanocrystals. Nanocrystals can be in close contact with the cell surface. However, as the crystal size increased, micron-sized COM crystals could not completely form a contact with the cell interface and shear stress decreases accordingly.24 Especially for the micron-grade aggregated crystals, the area touched on the cell surface only accounted for a small proportion of its surface area. That is, the specific surface area of the aggregates does not reflect the real contact area between the crystal and the cell surface.
5) In many cases, conductivity is linked directly to the total dissolved particles. For COM crystal suspensions, the higher the conductivity, the more the number of free Ca2+ and Ox2− ions in the solution. Schepers et al.25 showed that oxalate with a concentration of less than 1.0 mmol L−1 had no significant toxicity to MDCK-II cells. Guo et al.26 studied the toxicity of oxalate to HPT cells and found that oxalate ions have no toxicity to HPT cells when their concentration is lower than 2.0 mmol L−1. Since the concentrations of released Ox2− ions of the five COM crystals in aqueous solution ranged from 0.132 to 0.249 mmol L−1 (Table 2), it is far lower than the reported cytotoxicity threshold value of the Ox2− concentration.25,26 Thus, the effects of released Ox2− ions from the COM crystals on cellular toxicity can be ignored.
As can be seen from Table 3, COM-elliptical with the largest (
01) face area exhibited the most serious damage in Vero cells, COM-flower-like with the sharpest crystal edge shows the second highest cytotoxicity, COM-hexagonal with the second highest crystal sharpness induced cytotoxicity, which ranked third, the cytotoxicity induced by COM-peanut-like with a minimum absolute value of zeta potential ranked fourth, and COM-spherical with the largest specific surface area exhibited minimal cytotoxicity. It can be learned that the most important factors affecting the cytotoxicity of the five micron-sized crystals are ranked as crystal face > crystal sharpness > zeta potential > specific surface area.
In summary, all the factors, namely larger (
01) face area, sharper crystal edge, and lower absolute value of zeta potential can increase the crystal toxicity to cells. However, the specific surface area is not a very important factor for these large sized crystals, because they cannot be in close contact with the cell surface, especially the flower-like aggregated COM. The cytotoxicity of the COM crystals with different morphologies was not determined via a single factor. Cytotoxicity was affected synthetically by the (
01) face area, crystal edge sharpness, zeta potential and specific surface area.
Inhibitors existing in urine exert a great influence on crystal morphology; thus, uptaking polyanionic substances, such as potassium citrate or polysaccharides, tends to induce the formation of crystals with blunt crystal edges, smaller active crystal faces and higher absolute values of zeta potential, so as to achieve the goals of reducing cell injury and stone risk.
4. Conclusions
Various morphologies of COM crystal were crystallized using different additives, such as gelatin, Na3cit, Na2EDTA, and NaCl. Gelatin molecules promoted the formation of elongated hexagonal COM crystals. Na3cit addition resulted in rounded crystal edges and corners. Na2EDTA produced crystals with rough surfaces, and NaCl addition promoted crystal aggregation. Cell viability and LDH release assays showed that COM crystals with different morphologies produced different degrees of damage to Vero cells. The damaging ability of COM crystals was correlated with the Ca2+ ion-rich (
01) face area, specific surface area, crystal edge sharpness, and zeta potential. Hence, the cytotoxicity of COM crystals was closely related to the crystal structure and morphology. These findings are helpful to understand the effects of mineralization conditions and additives on crystal morphology, and identify the relationship between crystal morphology and cytotoxicity.
Conflicts of interest
The authors declare no competing financial interest.
Acknowledgements
This work was supported by the National Natural Science Foundation of China (NO. 21371077).
References
- M. R. Naghii, E. Eskandari, M. Mofid, M. Jafari and M. H. Asadi, Antioxidant therapy prevents ethylene glycol-induced renal calcium oxalate crystal deposition in Wistar rats, Int. Urol. Nephrol., 2014, 46, 1231–1238 CrossRef CAS PubMed.
- A. Trinchier, C. Castelnuovo, R. Lizzano and G. Zanetti, Calcium Stone disease: a multiform reality, Urol. Res., 2005, 33, 194–198 CrossRef PubMed.
- N. Bouropoulos, S. Weiner and L. Addadi, Calcium oxalate crystals in tomato and tobacco plants: morphology and in vitro interactions of crystal-associated macromolecules, Chem. – Eur. J., 2001, 7, 1881–1888 CrossRef CAS.
- S. Guo, M. D. Ward and J. A. Wesson, Direct visualization of calcium oxalate monohydrate crystallization and dissolution with atomic force microscopy and the role of polymeric additives, Langmuir, 2002, 18, 4284–4291 CrossRef CAS.
- D. R. Basavaraj, C. S. Biyani, A. J. Browning and J. J. Cartledge, The role of urinary kidney stone inhibitors and promoters in the pathogenesis of calcium containing renal stones, EAU-EBU Update Ser., 2007, 5, 126–136 CrossRef.
- Y. Shirane, Y. Kurokawa, S. Miyashita, H. Komatsu and S. Kagawa, Study of inhibition mechanisms of glycosaminoglycans on calcium oxalate monohydrate crystals by atomic force microscopy, Urol. Res., 1999, 27(6), 426–431 CrossRef CAS PubMed.
- S. P. Deng, H. Zheng and J. M. Ouyang, Crystallization rules of calcium oxalate crystals in lithogenic urine and in healthy urine in vitro, Mater. Sci. Eng., C, 2006, 26(4), 683–687 CrossRef CAS.
- J. Y. He, S. P. Deng and J. M. Ouyang, Morphology, particle size distribution, aggregation, and crystal phase of nanocrystallites in the urine of healthy persons and lithogenic patients, IEEE Trans. Nanobioscience., 2010, 9(2), 156–163 CrossRef PubMed.
- M. Tsujihata, Mechanism of calcium oxalate renal stone formation and renal tubular cell injury, Int. J. Urol., 2008, 15, 115–120 CrossRef CAS PubMed.
- X. Huang, X. Teng, D. Chen, F. Tang and J. He, The effect of the shape of mesoporous silica nanoparticles on cellular uptake and cell function, Biomaterials, 2010, 31(3), 438–448 CrossRef CAS PubMed.
- V. Thongboonkerd, T. Semangoen and S. Chutipongtanate, Factors determining types and morphologies of calcium oxalate crystals: Molar concentrations, buffering, pH, stirring and temperature, Clin. Chim. Acta, 2006, 367, 120–131 CrossRef CAS PubMed.
- T. A. Larichev, E. N. Kharchenko, A. B. Abisheva and B. A. Sechkarev, Study of recrystallization kinetics of AgBr microcrystals in water–gelatin gel, Colloid J., 2003, 65(6), 745–751 CrossRef CAS.
- S. Farmanesh, S. Ramamoorthy, J. Chung, J. R. Asplin, P. Karande and J. D. Rimer, Specificity of growth inhibitors and their cooperative effects in calcium oxalate monohydrate crystallization, J. Am. Chem. Soc., 2014, 136(1), 367–376 CrossRef CAS PubMed.
- A. M. Cody and R. D. Cody, Calcium oxalate trihydrate phase control by structurally-specific carboxylic acids, J. Cryst. Growth, 1994, 135(1–2), 235–245 CrossRef CAS.
- S. H. Xuan, L. Y. Hao, W. Q. Jiang, L. Song, Y. Hu, Z. Y. Chen, L. F. Fei and T. W. A. Li, FeCO3 precursor-based route to microsized peanutlike Fe3O4, Cryst. Growth Des., 2007, 7(2), 430–434 CAS.
- Y. Y. Li, J. P. Liu, X. T. Huang and G. Y. Li, Hydrothermal synthesis of Bi2WO6 uniform hierarchical microspheres, Cryst. Growth Des., 2007, 7, 1350–1355 CAS.
- L. Xu, J. N. Shen, C. L. Lu, Y. P. Chen and W. H. Hou, Self-assembled three-dimensional architectures of Y2(WO4)3: Eu: controlled synthesis, growth mechanism, and shape-dependent luminescence properties, Cryst. Growth Des., 2009, 9(7), 3129–3136 CAS.
-
M. King, W. F. Mcclure and L. C. Andrews, Powder diffraction file alphabetic
index, inorganic phases-organic phases, International center for diffraction data, Newtown Square, PA, 1992 Search PubMed.
- R. Selvaraju, G. Thiruppathi and A. Raja, FT-IR spectral studies on certain human urinary stones in patients of rural area, Spectrochim. Acta, Part A, 2012, 93, 260–265 CrossRef CAS PubMed.
- R. C. Walton, J. P. Kavanagh and B. R. Heywood, The density and protein content of calcium oxalate crystal precipitated from human urine: a tool to investigate ultrastructure and the fractional volume occupied by organic matrix, J. Struct. Biol., 2003, 143, 14–23 CrossRef CAS PubMed.
- A. Barhoum, H. Rahier, R. E. Abou-Zaied, M. Rehan, T. Dufour, G. Hill and A. Dufresne, Effect of cationic and anionic surfactants on the application of calcium carbonate nanoparticles in paper coating, ACS Appl. Mater. Interfaces, 2014, 6(4), 2734–2744 CAS.
- X. Sheng, M. D. Ward and J. A. Wesson, Crystal surface adhesion explains the pathological activity of calcium oxalate hydrates in kidney stone formation, J. Am. Soc. Nephrol., 2005, 16(7), 1904–1908 CrossRef CAS PubMed.
- X. X. Sheng, T. S. Jung, J. A. Wesson and M. D. Ward, Adhesion at calcium oxalate crystal surfaces and the effect of urinary constituents, Proc. Natl. Acad. Sci. U. S. A., 2005, 102(2), 267–272 CrossRef CAS PubMed.
- X.-Y. Sun, J.-M. Ouyang, Y.-B. Li and Q.-Z. Gan, Renal epithelial cell injury induced by calcium oxalate monohydrate depends on their structural features: Size, surface, and crystalline structure, J. Biomed. Nanotechnol., 2016, 12, 2001–2014 CrossRef CAS.
- M. S. J. Schepers, E. S. van Ballegooijen, C. H. Bangma and C. F. Verkoelen, Oxalate is toxic to renal tubular cells only at supraphysiologic concentrations, Kidney Int., 2005, 68(4), 1660–1669 CrossRef CAS PubMed.
- C. Guo, T. A. Cenac, Y. Li and K. E. McMartin, Calcium oxalate, and not other metabolites, is responsible for the renal toxicity of ethylene glycol, Toxicol. Lett., 2007, 173(1), 8–16 CrossRef CAS PubMed.
Footnote |
† Present address: Department of Chemistry, Jinan University, Guangzhou 510632, China; Institute of Biomineralization and Lithiasis Research, Jinan University, Guangzhou 510632, China, E-mail address: toyjm@jnu.edu.cn |
|
This journal is © The Royal Society of Chemistry 2018 |
Click here to see how this site uses Cookies. View our privacy policy here.