DOI:
10.1039/C8BM00471D
(Review Article)
Biomater. Sci., 2018,
6, 2282-2297
Mucins as multifunctional building blocks of biomaterials†
Received
27th April 2018
, Accepted 6th July 2018
First published on 13th July 2018
Abstract
Mucins are large glycoproteins that are ubiquitous in the animal kingdom. Mucins coat the surfaces of many cell types and can be secreted to form mucus gels that assume important physiological roles in many animals. Our growing understanding of the structure and function of mucin molecules and their functionalities has sparked interest in investigating the use of mucins as building blocks for innovative functional biomaterials. These pioneering studies have explored how new biomaterials can benefit from the barrier properties, hydration and lubrication properties, unique chemical diversity, and bioactivities of mucins. Owing to their multifunctionality, mucins have been used in a wide variety of applications, including as antifouling coatings, as selective filters, and artificial tears and saliva, as basis for cosmetics, as drug delivery materials, and as natural detergents. In this review, we summarize the current knowledge regarding key mucin properties and survey how they have been put to use. We offer a vision for how mucins could be used in the near future and what challenges await the field before biomaterials made of mucins and mucin-mimics can be translated into commercial products.
| Georgia Petrou is a PhD student at KTH, Royal Institute of Technology. She holds a masters in biomedicine from the Karolinska Institute. During her PhD studies, Georgia is developing and studying new mucus materials and mucus-interacting materials for biomedical applications. These include mucin-based hydrogels for wound healing applications and engineered mucoadhesive recombinant silk materials for mucosal treatments. |
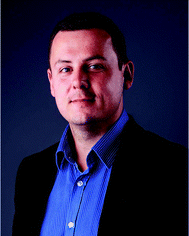 Thomas Crouzier | Dr Thomas Crouzier is team leader at the Royal Institute of Technology in the department of Chemistry. He received a doctoral degree in biomedical science from the University of Montpellier in 2010 in team of Prof. Picart and completed a postdoctoral stay at the Massachusetts Institute of Technology in the group of Prof. Ribbeck, where he blended his background in biomaterial engineering with the field of mucus. His current research interests lie in the development of new mucus engineering strategies and mucin-based biomaterials to solve biomedical challenges. |
Introduction
Mucins are a family of large glycoprotein polymers expressed both as cell membrane-tethered molecules and as a major component of the mucus gel, when secreted by the goblet cells of the epithelium. Mucins have a vital role in the offensive and defensive mechanisms of many species. The multiple and diverse physiological roles of mucins have long been of interest, but it is only more recently that some of their biophysical and biological properties have been investigated in more detail. The main properties attributed to mucins include barrier properties, dynamicity, hydration, lubrication, and bioactivity, as summarized in Table 1. In this review, we present mucin glycoproteins as a biomaterial with a strong potential for medical and chemical applications. We hypothesise that mucins could develop in a similar fashion as other biopolymers extracted from animal or plant tissues such as collagen, heparin, hyaluronic acid, and alginate, which have become major industries with applications in the food, agriculture, and biomedical sectors. Several research groups have started exploring the applicative potential of purified mucins, as briefly mentioned in previous review articles.1–3 Mucins are then considered not only as an important part of animal physiology, but also as functional building blocks that can be used to create new multifunctional biomaterials. We provide an overview of the current understanding of each key mucin property, then describe how the various properties have been exploited. We go beyond the current state of the art and analyse how recent discoveries involving mucins could result in technological innovations. Finally, we suggest future directions for mucus research and point to a number of challenges that awaits the nascent field of mucin engineering.
Table 1 List of the properties for which mucins have been applied. The references to relevant landmark studies and reviews are listed for each of the properties
Property |
Description |
Ref. |
Barrier |
Mucins assemble through covalent and noncovalent intermolecular interactions into a mesh of polymers that forms a size exclusion barrier to molecules, particles, and pathogens. Mucins typically bind particles and molecules that bear positive charges, thiol groups, hydrophobic moieties, and sugar-binding domains. Mucins also form stable coatings with anti-fouling properties on a variety of surfaces. |
4 and 5
|
Dynamicity |
The rheological properties of mucin gels depend on concentration, pH, mucin–mucin associations mediated by numerous factors such as Ca2+ ions, and the mucin glycan composition. A constant fine-tuning of mucus rheological properties occurs in vivo, determining mucus clearance rates and barrier properties. |
6–8
|
Hydration |
Mucin gels hold water to the surface of the epithelial cells, which is thought to protect the mucosa from dehydration. The mucin-associated glycans can associate with water molecules via hydrogen bonding. The hydrated mucin molecules can entangle into networks that trap large amounts of water. Hydration of mucins is intimately linked with their rheological, tribological, and barrier properties. |
9 and 10
|
Lubrication |
Mucins are highly lubricating molecules when placed on surfaces, comparable with some of the best synthetic lubricants. Mucins’ exceptional lubricity is due to its structure, hydration, and charge that contribute to the formation of hydration shells at the surface, acting as lubricating elements. |
11 and 12
|
Bioactivity |
Mucins are bioactive towards microbes and mammalian cells. As discussed in the text below, mucins can directly act as ligands for cell surface receptors or sequester biologically active molecules. |
13 and 14
|
What are mucins?
A thin layer of a few hundreds of micrometers of hydrogel protects our mucosa from dehydration caused by exposure to air, from the mechanical stress of the food we swallow, and from the millions of viruses and bacteria that we encounter everyday. This feat is in large part achieved by mucins; a family of highly functional glycoproteins, of which a subcategory assembles in vivo to form mucus gels.15 Mucins are endowed with a rich chemical diversity, since they combine a long protein backbone with O-linked glycans terminated by a variety of sugar units (Fig. 1A). Structurally, the dense glycosylation of mucins confers a relatively extended conformation to the molecule, which can associate into large oligomers (in the tens of MDa) via disulfide bonds and other weaker interactions (Fig. 1B–D). Membrane-tethered mucins contain transmembrane domains and decorate the cell surface, extending several hundreds of nanometers outwards.16 Similarly to their secreted counterparts, membrane-tethered mucins contribute to the protection of the cell, while also being involved in intracellular signalling events. The chemical nature of mucins is reviewed in great detail elsewhere17–20 and thus will not be covered here. It is worth noting that the term mucin is used rather loosely.21 Although several mucins clearly belong to the same family of proteins due to strong amino acid sequence homologies, others possess rather unrelated protein structures and were named mucins mainly because they colocalize and share some of the functionalities with the mucin protein family. Since this review focuses on mucin functionalities, we will use a loose definition and include all glycoproteins that have been named mucins or mucin-like.
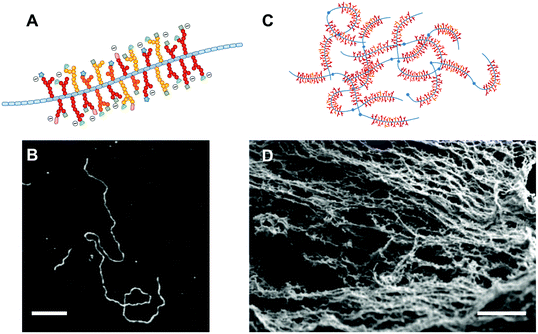 |
| Fig. 1 Mucin molecules and hydrogels. (A) All mucin molecules share the common feature of a central protein core (depicted in blue) decorated with regions of dense O-linked glycosylation. The terminal sugars of the glycans display an impressive diversity and can bear a negative charge, if composed of the sialic acid sugar. (B) Atomic force microscopy images of mucin molecules adsorbed onto surfaces reveal a uni-dimensional molecule that can be in the hundreds of nanometers in length and only a few nanometers thick. The bar scale is 200 μm. (C) The mucin monomers assemble into high molar mass oligomers that can interact through intermolecular bonds and entanglement into physical hydrogels. (D) Electron scanning microscopy images of a mucus gel, composed of the mucin fibrillar network, supplemented with a range of soluble proteins, lipids, and salts. The scale bar is 200 nm. The Fig. 1B is reprinted from ref. 22 with permission from Elsevier and Fig. 1D reprinted from ref. 23. | |
Sources of secreted mucins
In nature, mucins are ubiquitous. Their complex chemistry and diversity allows them to fulfil a variety of roles, including organism protection,24 locomotion,25 and food capture.26 They can be extracted from mucosal tissues either by collecting the mucus itself and extracting the mucins,27 or by homogenizing whole tissues.28–30 Purification is achieved by leveraging mucins’ unique physical–chemical characteristics, including their solubility profiles in solvents, large size, and strong net negative charge. Although the glycosylated fractions of mucins are relatively resistant to proteolytic degradation, the unglycosylated sections are more easily degraded,31 which can alter the gelling properties of the mucins.32–34 Gel forming mucins can be purified, while preserving their structure, by using a combination of gentle water dissolution, size exclusion chromatography and cesium chloride density gradient centrifugation to remove mucin-bound impurities, including lipids and nucleotides.35,36 Mucins are commonly extracted from abundant animal sources, including pigs, cows, snails, chicken eggs, and jellyfish. The protein sequence differs based on the organism and tissue, varying in size, extent of glycosylation, and in the presence of domains such as Von Willebrand19 or cysteine rich regions. The glycosylation diversity, length, and structure are even more prone to variations. For instance, pig gastric mucins have longer glycan chains but with less terminal sialic acids than bovine submaxillary mucins.37,38 However, even within the same organism, differences in inflammatory39 and disease40 states of the epithelium and in the composition and activity of the microbiota41 can alter the glycan composition. The common features of the mucins referred to herein are: an extended central protein core, regions of dense O-glycosylation, and the presence of acidic sugars providing an overall negative charge to the molecule.
The challenges inherent to the extraction of natural products from animal tissues, such as batch to batch variability and risk of pathogen contamination, could be addressed by developing synthetic mucins. Recent advances in synthetic mucins have been reviewed by Authimoolam et al.1 and by Kiminori et al.2 These elegant approaches recapitulate some of the properties attributed to mucins. For instance, the Bertozzi group has been successful in generating mimics of the shorter membrane-bound mucins that bind cell receptors.42 However, the synthesis of complex mucins, including tens of different terminal glycan structures, a peptide core with thousands of amino acids, and the ability to auto-assemble into megadalton structures still remain elusive.
Mucin-based protective barriers
Mucins are our first line of defence in the protection of our exposed epithelium, forming a physical barrier that prevents the passage of deleterious molecules.4 The pore size of mucin gels in vivo is thought to be in the hundreds of nanometers in cervical mucus, excluding larger objects such as bacteria and yeast cells.43 In the colon, a double layer of mucus protects the epithelium from the billions of bacteria thriving in the lumen. A top loose layer allows bacteria to interact with the mucin, while a more compact bottom layer creates a near sterile buffer zone to protect the epithelium.44 In addition to acting as a filter, the mucus gels act as a barrier by affinity sorting. Objects smaller than the pore size such as viruses, also can be trapped in the mucin network by binding to the ligands present on the tip of the glycans. Once bound to the mucins, the pathogens are removed from the mucosa by an active turnover of the mucus layer.24
These properties have inspired bioengineers to build mucin-based barriers to pathogens. The spontaneous formation of stable mucin coatings on a range of materials makes this approach particularly attractive to prevent microorganisms from colonizing surfaces. Purified mucins reduce the adhesion of Streptococcus pneumoniae, Staphylococcus aureus, Staphylococcus epidermidis, and Candida albicans, when adsorbed on synthetic polymers45–47 (Fig. 2D and E). Similar effects were reported with mammalian cells. For instance, neutrophils adhere poorly on mucin coatings, which was also correlated with lower activation levels compared to uncoated surfaces.48,49 In our group, we have used mucins and microfluidic devices to create anti-adhesive patterns, effectively confining fibroblasts, myoblasts, and epithelial cells for several days50 (Fig. 2F). The surface of poly lactic-co-glycolic acid (PLGA) nanoparticles has also been functionalized with pig gastric mucins to limit protein adsorption, shield surface antigens and enhance blood compatibility.51 The anti-fouling and cell repellent properties of mucin coatings were first applied in vivo by Janairo et al. who functionalized small synthetic vascular grafts of (1 mm diameter) with mucins to improve the hemocompatibility of the device.52 When implanted in mice, the mucin-functionalized grafts exhibited a good patency rate and promoted cell infiltration. Mucin coatings have also been used to coat the surface of porous PDMS matrices, mimicking the affinity-based selective filtering properties of mucus gels. Due to the negative charges on mucin glycans, the mucin-functionalized filters bound to positively charged particles while allowing negatively charged particles to flow through.53
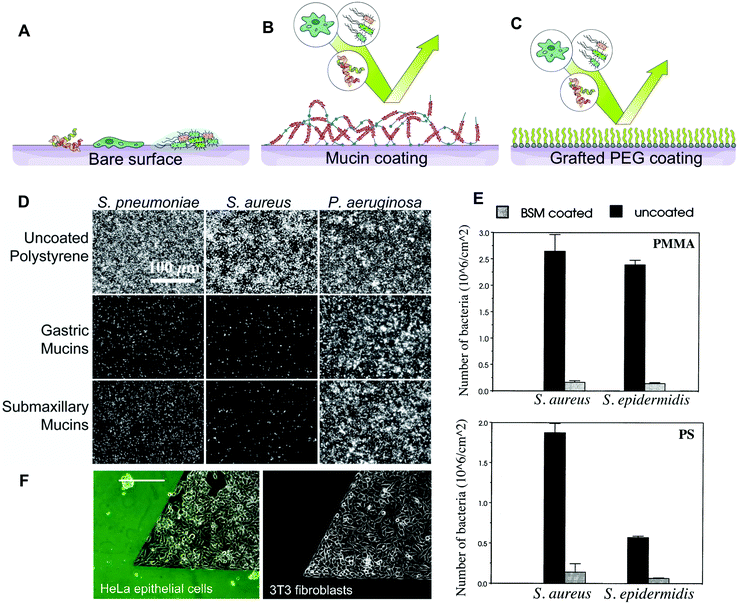 |
| Fig. 2 Barrier properties of mucin coatings. (A) Most surfaces are susceptible to colonization by macromolecules, cells and bacteria. (B) Mucins can adsorb to form stable coatings on a number of surface chemistries. Mucin coatings can repel bacteria, proteins, and mammalian cells. (C) Mucin coatings are hypothesised to resemble those of PEG-based antifouling surfaces. (D–E) The antifouling properties of mucin coatings as shown by microscopy observation and quantification of bacteria binding. Pseudomonas aeruginosa and other bacteria possess receptors that bind the glycan and protein domains of the mucins, allowing them to overcome the repellent effect. (F) The repellent effect of mucin coatings towards mammalian cells was utilized to patterned epithelial, fibroblast and myoblast cells on tissue culture polystyrene. (D) is reprinted from ref. 45 with permission from Wiley Materials, (E) is reprinted from ref. 47 with permission from Elsevier, and (F) is reprinted with permission from ref. 50, copyright 2018 American Chemical Society. | |
The anti-fouling effect that exists for some mucin coatings, is likely due to the specific chemical nature of the mucins and to the mucins’ arrangement at the surface. On hydrophobic surfaces, it is hypothesized that mucins extend their highly glycosylated and highly hydrated domains into the aqueous solvent, while the non-glycosylated protein domains interact with the surface. This conformation is suggested from quartz crystal microbalance with dissipation monitoring (QCM-D) that measures large frequency shifts and dissipations for mucins physisorbed on a number of surfaces, when mucin is adsorbed on the sensor.54 These mucin-coated surfaces are somewhat comparable to PEG brushes commonly used for anti-fouling coatings,55 since both form a hydration shell and cause steric hindrance preventing adsorption (Fig. 2A–C). It is worth noting that the adsorption of mucins is very much dependent on the type of mucins used,56 the surface properties47,50 and coating conditions.46 In addition, some microorganisms are equipped with membrane receptors that are able to bind mucins and overcome the repulsive effect.
The strong interaction of mucins with the surface might have denaturing effects that affect important intra- and inter-molecular interactions. The auto-assembly of mucins into thicker coatings by the layer-by-layer technique with other polymers and proteins3 provides better mimics of the intermolecular interactions found in mucus gels. Such thin films can also be combined with bioactive compounds, such as antimicrobial peptides57 found in intestinal mucus. However, mucins are the closest to their original conformation when presented in solutions or gels. Mucin gels and solutions have been used to investigate mucins’ protective role against microbes,58–61 viruses62,63 and toxins64,65 (Fig. 3A). It is not entirely clear whether the protective effects of mucin gels are due to steric hindrances (i.e. physically preventing the pathogens from reaching the host cells) or rely on specific signalling, perhaps mediated by surface proteins and the mucins’ glycans or peptidic core. However, polymer solutions mimicking mucus gel rheological properties do not recapitulate the protective effect to the same extent58,59 (Fig. 3B). The importance of functional groups on mucins is also suggested by recombinant mucin-like proteins66 which are unable to polymerise into gels but can bind to viral adhesion proteins67 and toxins68 and by artificially poly-sialiated mucins that can counteract the cytotoxicity of extracellular histones secreted by activated neutrophils.69
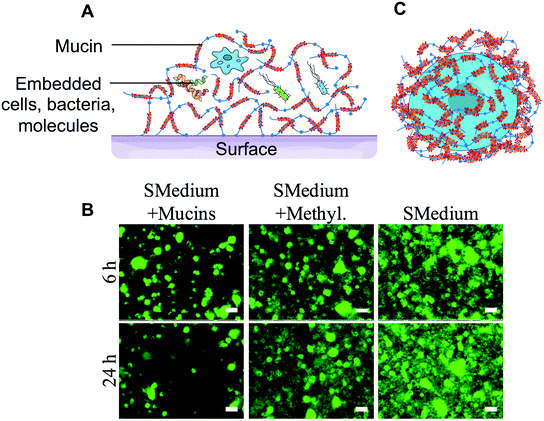 |
| Fig. 3 Barrier properties of mucin hydrogels. (A) Three-dimensional mucin hydrogels mimic the native mucus barrier by preventing bacteria and other pathogens from accessing the surface. (B) Salivary mucin gels could limit the colonization of glass surfaces by Streptococcus mutans grown in BHI containing 1% sucrose (SMedium), while methylcellulose (denoted by Methyl.), a polymer of similar viscosity to mucins, had no effect. (C) Certain parasites and cancer cells secrete mucins on their surface to create a barrier and evade the immune system. The encapsulation of cells with mucins could constitute a strategy to provide chemical and biological protection to individual cells. (B) is adapted from ref. 60 with permission from the American Society for Microbiology. | |
Mucins have also been suggested to form physico-chemical barriers. For instance, mucins can act as a buffer layer to protect the epithelial lining from the strong acidity and high protease activity found in the stomach.70 The charged terminal sialic acid sugar residues can act as a biological mask, for instance, by shielding antigens from recognition by the immune system.71–73 This strategy is employed by the Trypanosoma cruzi parasite, that secretes a coat of mucin-like molecules that shield the pathogen from the host's immune response. Mucinous tumors also produce a physical mucin barrier that shields tumors from the immune system and limits cell–cell interactions that facilitate metastasis.74 Interestingly, it was standard procedure from the 1930s up to the 1970s and even occasionally more recently,75 to co-administer mucins along with pathogenic bacteria,76,77 viruses,78 and fungi79 in small animal models, such as mice, to lower their resistance and develop pathologies. Mucins were also found to prolong the survival of bacterial cultures.80 The formation of a protective mucin coating around the bacteria, which was shown to prevent the entrance of Indian dye inside the cells, could explain the effect.81 However, one must also consider that the enhanced virulence could also be a direct effect of mucin preparation impurities on the host immune system. Although this line of research seems to have faded, the prospect of coating cells with protective macromolecules, such as mucins, is attractive and could find application in probiotic delivery or cell therapies (Fig. 3C).
Mucins could also provide protection against proteolytic activities due to the dense glycosylation on the mucin protein core that limits enzymatic access to the protein.31 Loomes et al., showed that replacing a glycosylated section of the human milk cholesterol esterase protein with a glycosylated section of the MUC6 mucin could confer good proteolytic resistance.82 Their results suggest that mucin fragments could be used to confer protease resistance to proteins and increase the half life of biologics used in therapies.
What lies ahead
With our improved understanding of mucins’ barrier function and properties, biomimetic approaches, consisting of the treatment of epithelial surfaces with exogeneous mucins to reinforce the mucosal barrier in the mouth, stomach, or gut, can again be considered. Indeed, studies from the 1930s suggest that pig gastric mucin solutions can be successfully used as treatment against gastric ulcers83 and a later study showed that their oral ingestion could provide some protection to rats against N-methyl-N′-nitro-N-nitrosoguanidine and NaCl induced gastric carcinomas.84 To our knowledge, the molecular mechanisms behind these effects have not been thoroughly investigated. One also can hypothesise that exogenous mucins can reconstitute a temporary protective patch over the epithelium, lowering inflammation levels, and leaving sufficient time for the epithelium to regenerate. More recently, mice overexpressing mucins or mucin fragments have shown a superior ability to be protected from pathogens in both the intestines85 and lungs.86 The exogenous delivery of mucins would be a simpler approach than the overexpression of mucins. However, the delivery of fully functional mucin molecules and their lasting presence at the site of interest remain challenges to be resolved. The barrier properties of mucins could also be used for the encapsulation and delivery of cells. For instance, a mucin encapsulation could protect probiotic bacteria from the low pH and proteolytic activity in the stomach, then release the bacteria in the intestines where the local microflora can degrade mucins.87
Exploiting the complex chemistry of mucins
The multi-functionality of mucins is closely related to their chemical complexity. The non-glycosylated domains of the glycoprotein exhibit a rich chemical diversity, including charged domains, hydrophobic domains, and thiolated groups that can engage in disulfide bridges. The mucin protein core is heavily substituted by glycan chains directly O-linked to tandem repeats of proline, threonine, and serine. A recent review by A. Corfield lists the glycan structures that make up mucin glycosylation.18 The diversity of the mucin glycan structures that have already been identified is striking, with tens of possible terminal sugars mediating interactions with cell receptors, extracellular biopolymers, lipids, and other small molecules and particles. Sulfate groups on N-acetylglucosamine and galactose, and carboxylic groups on sialic acid sugars provide an overall negative charge to mucins in most pH conditions. Mucin glycans are rich in hydroxyl groups, which can engage in intramolecular and intermolecular hydrogen bonds. The chemical diversity of mucins has found several interesting applications.
The rich library of mucin glycan structures are ligands to a wide range of sugar binding proteins found in viruses, bacteria, animal cells, and plant cells. For instance, mucin-associated glycans have binding affinities with many lectin-type proteins extracted from plant tissues, several of which are now industrially produced. The specificity of lectin binding has been used to probe the glyco-composition of mucins and mucus.88 Our group has exploited the multivalent binding of lectins to mucins to generate sugar-responsive thin films that are assembled layer-by-layer.89 The high avidity of the lectin–mucin interactions led to thin films surprisingly resilient to pH and ionic strength that could be selectively disassembled in the presence of a sugar solution. These assemblies were then used as sacrificial substrates to create self-standing films90 but could also be used for drug delivery, especially for insulin that could be released when triggered by high glucose concentrations.
The presence of hydrophobic protein domains and hydrophilic glycosylated domains effectively make mucins good surfactants. Mucins have been exploited as natural polymeric surfactants to host and release hydrophobic drugs. Bovine submaxillary mucins functionalized with methacrylate resulted in robust UV-crosslinked hydrogels91 (Fig. 4E). Anti-cancer drug paclitaxel and the positively charged antibiotic polymyxin B were successfully loaded and released in a sustainable manner over days from the covalently cross-linked mucin hydrogels (Fig. 4F), while lidocaine was shown to be released over hours from mixed bovine submaxillary mucin (BSM)-gelatin hydrogels.92 The sustained release of the drugs could be attributed to interactions with mucins, since the high molar mass but poorly mucoadhesive molecules released faster than the two small model drugs. The hydrophilicity and negative charge of mucins were also used by Nowald et al. who mixed pig gastric mucins with methylcellulose to create thermo-sensitive self-standing hydrogels that bound to positively charged molecules.93 By removing some of the pig gastric mucin glycosylation, Fukui et al. were able to generate nanoparticles that could incorporate positively charged antibacterial lysozyme enzymes.94 The particles degraded over time and released their content, due to the proteolytic action of the encapsulated lysozyme.
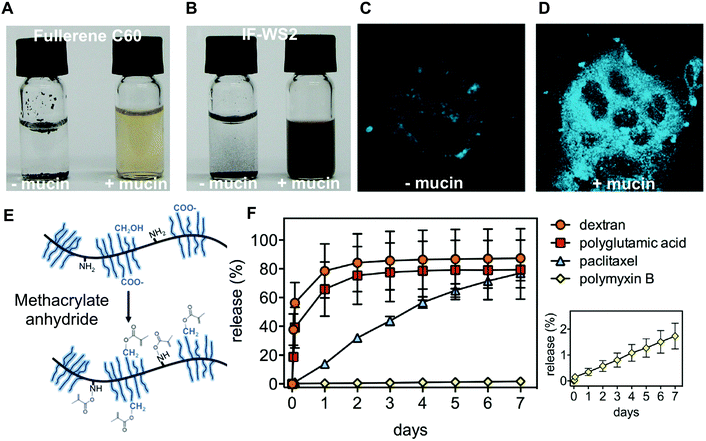 |
| Fig. 4 Mucin used for its amphiphilic properties. Comparison of aqueous solutions (vial to the left) or mucin solutions (vial to the right) of (A) fullerene C60 and (B) inorganic fullerene-like disulfide tungsten (IF-WS2). The amphiphilic properties of mucins were shown to enhance the uptake of benzo[a]pyrene (BaP) into CaCo2 epithelial cells. (C) Fluorescence microscopy (excitation at 390 nm) of BaP exposed CaCo2 cells. (D) Fluorescence microscopy (excitation at 390 nm) of BaP-BSM exposed CaCo2 cells. (E) Methacrylate BSM was generated to create photocrosslinkable mucin hydrogels. (F) The covalently cross-linked mucin hydrogels release in a sustained manner both a hydrophilic and positively charged antibiotic and a hydrophobic anticancer drug. Dextran and polyglutamic acid are control molecules showing limited interactions with the mucin hydrogel. (A and B) are reprinted from ref. 95 with permission from Wiley Materials, (C and D) are reprinted with permission from ref. 96, copyright 2018 American Chemical Society. (E and F) are reprinted from ref. 91 with permission from Elsevier. | |
The amphiphilicity of mucins has also been used to generate water-soluble carbon nanomaterials, a prerequisite to apply carbon nanotubes in biomedical applications. This was achieved by using both a mimic of membrane tethered mucins, consisting of a long C18 lipid hydrophobic anchor,42 and the full bovine submaxillary mucins.95 In related work, Gozin and his group have thoroughly investigated the solubilization of hydrophobic nanoparticles by mucins. In particular, they investigated the ability of mucins to promote the cellular uptake of two hydrocarbons: anthracene and coronene96 (Fig. 4A and B). The same group also showed that BSM can bind and solubilize these hydrocarbons, which enhanced their uptake by the epithelial Caco-2 cell line (Fig. 4C and D). Deglycosylated BSM had no effect on the hydrocarbon uptake, which suggested that mucin-associated glycans are essential for the effect to occur. Similar results were also reported for pig gastric mucins (PGM).95,97 Although these studies point to the potential dangers of mucin-mediated cellular uptake of hydrocarbons, they also suggest that mucins could help to efficiently deliver hydrophobic therapeutics to cells. Similarly, the capacity of jellyfish mucus to strongly interact with gold nanoparticles implies that mucus could mediate the toxicity of nanoparticles but also points to the use of mucin-based materials for decontamination purposes.98 Other applications of mucins’ promiscuous interaction properties include the separation of dyes to create white luminescent devices,99 the promotion of organic reactions,100 and the synthesis of chiral silver nanoparticles.101
What lies ahead
The diverse repertoire of glycan structures that decorate mucins holds great potential for various applications. Milk-derived mucin glycans have been suggested to be used to modulate bacterial population of the gut.102 One can also foresee that materials functionalized with mucin glycans could capture viruses, bacteria, and toxins that contain glycan-binding proteins and be used as the basis for filtration systems and sensors for diagnostic purposes. Recent academic work has shown that mucins can be used to carry and deliver therapeutic molecules and several patents mention the use of mucins to deliver proteins and peptides.103,104 Mucins’ surfactant properties could also find new uses. Surfactants are widely used in industrial processes as foaming agents, pigment dispersants, wetting agents, solubilizers, and emulsifiers. Natural surfactants like mucins and mucin-like proteins are good candidates to replace their synthetic counterparts that are increasingly disfavored over greener alternatives. Indeed, salivary mucins are known to affect emulsions in the mouth105 and ovomucins extracted from egg white are used for emulsion and as a stabilizing agent of foams.106 These properties could be exploited to create new foods. Böni et al. recently investigated mixing hagfish slime containing mucins with soy emulsions and soy protein isolate suspension, to form a cohesive hydrogel.107 Once cooked, this mixture resembled cooked tofu in many respects.
Exploiting the hydration and lubrication properties of mucins
Another core property of secreted mucins is their ability to hydrate and lubricate the mucosal epithelium.12 On synthetic surfaces, even from dilute solutions, mucins can form highly hydrated coatings containing more than 95% water that can efficiently lubricate (Fig. 5A). Mucins’ exceptional lubricity is attributed to aqueous boundary lubrication, a mechanism reviewed elsewhere by Coles et al.12 The hydration and lubrication role of mucins is perhaps most obvious in saliva, which contains MUC5B and MUC7 mucins108 and which is essential for speech and swallowing. Xerostomia, also termed dry mouth, is caused by deficiencies in salivary mucins due to aging, chemotherapy, radiotherapy, or antidepressant medications and affects millions throughout the world.109
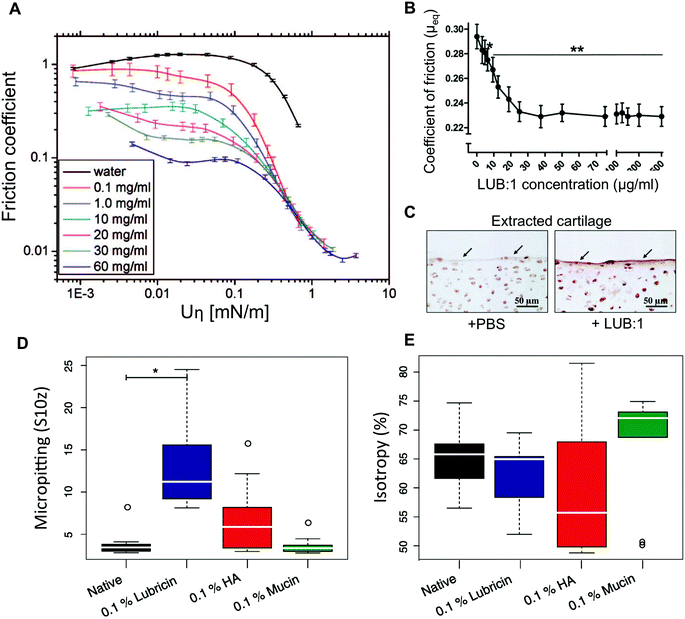 |
| Fig. 5 Hydration and lubrication of mucins. (A) Stribeck curve of pig gastric mucins dissolved in pure water lubricating smooth polydimethylsiloxane (PDMS) surfaces. (B) Boundary friction coefficient measured on cartilage explants lubricated with lubricin. (C) Histology images revealing the beneficial effect of a lubricin (LUB:1) injection in the knee compared to control (PBS) in a rat model of osteoarthritis. The sections were immunostained with anti-lubricin antibody. In addition to lubricity, mucins and lubricins are able to limit wear of surfaces in friction. In a steel on cartilage model system, the surface topography of the cartilage was analysed for the effect of wear. (D) The cartilage lubricated with 0.1% (w/v) hyaluronic acid (HA) or lubricin solutions led to micropitting effects. (E) Lubrication by 0.1% (w/v) HA led to the formation of tracks in the cartilage that decreased surface isotropy compared to samples lubricated with mucins at a similar concentration. The whiskers denote the 1.5 IQR (n = 9). A is reprinted with permission from ref. 130 copyright 2018 American Chemical Society. (B and C) are reprinted from ref. 122 with permission from Wiley Materials, and (D and E) are reprinted from ref. 123 with permission from Elsevier. | |
Existing saliva substitutes recapitulate some of the rheological properties of saliva. However, they fail to restore the original mouthfeel for extended periods of time. In one study, salivary substitutes, containing mucins extracted from porcine stomachs, were shown to be better at relieving dry mouth symptoms than other polymer solutions.110–113 However, a later double-blinded, single-phase placebo-controlled trial of the mucin-containing oral spray did not show a significant difference over a water-based placebo.114 It is possible that the hydrating and lubricating effect is compromised, due to alterations made to the mucin molecules during the rather harsh tissue extraction and protein purification protocols that are used to fabricate this product.115 Protein degradation and even partial deglycosylation of mucins negatively affect their hydration and lubrication in vitro.10 The mucin-based saliva substitutes may, however, carry other advantages, such as their ability to bind to bacteria and prevent oral infections.116 Mucins are efficient boundary lubricators for a surprisingly large number of materials.12 They are, therefore, good candidates as biolubricants to be used in the prevention of implant wear or treatment of osteoarthritis. One study used qniumucin extracted from the Aurelia aurita jellyfish injected intra-articularly in a rabbit osteoarthritis model either alone or mixed with hyaluronic acid.117 The combination of hyaluronic acid and mucins showed a significant reduction in cartilage degeneration. Mucins extracted from porcine stomachs were also used to lubricate contact lenses and tested in contact with corneal tissue explants. Although mucins were not better at lubricating than hyaluronic acid, the careful analysis of the corneal tissue surface showed that the mucins were better at preventing tissue damage.118 Although such reports on the use of mucins for their lubricity are still limited, there is a relatively large body of literature reporting the use of lubricin, a mucin-like glycoprotein which is naturally present in the joints.119,120 A recombinantly expressed version of this mucin-like protein was shown to lubricate explanted bovine cartilage121 and prevent cartilage degeneration in a rat osteoarthritis model122 (Fig. 5B and C). However, also on explanted cartilage, mucins were shown to outperform recombinant lubricin in wear reduction123 (Fig. 5D and E).
In addition to its role in lubrication, the mucus layer also ensures proper hydration of the mucosal surfaces exposed to air. This constant struggle against dehydration is carried by mucins thanks to their exceptional hygroscopic properties.124 Mucins’ ability to bind large amounts of water could make them interesting alternatives to the synthetic and natural polymers commonly used in cosmetics to maintain skin hydration. This particular aspect was investigated for bovine maxillary mucin (BSM)125 and jellyfish mucins which seemed to perform better than hyaluronic acid.126 Skin cosmetics, based on snail mucins, date back to the 18th century when snail and slug mucus-based concoctions were used for treating skin wounds and inflammations127 and are re-gaining their popularity. In addition to mucins, snail and slug mucus contains minerals, vitamins, and bioactive peptides that are likely to play a role in skin rejuvenation and wound healing reported in several studies.128,129
What lies ahead
Mucin-rich materials are obviously good hydrators and lubricators and, therefore, have been used for centuries for lubrication, such as when slugs were used to lubricate the wooden axle-trees.131 Today, mucins are investigated as a way to recapitulate the lubrication and hydration of ocular and oral surfaces, which are the results of properties and complex interplay between the components of the saliva or the tear film. Although our limited understanding of these phenomena hampers progress towards effective saliva and tear film substitutes, mucins are good candidates to be used in mucus moisturizing products.132 Mucins could also be used to functionalize medical devices that encounter potentially damaging friction with soft tissues, including contact lenses, catheters,133 and endoscopes. However, these applications will depend on the availability of purified, full-length, and non-degraded mucin molecules that retained their original properties.
In cosmetics, mucins could be investigated as dermal fillers. Similarly to hyaluronic acid and collagen, which are currently used as dermal fillers, mucins have a high molecular weight and hydrated molecules that can be injected subcutaneously to stretch the dermis. However, in contrast to hyaluronic acid and collagen, mucins are more resistant to enzymatic degradation31,82,134 and could, therefore, persist longer without the use of chemical crosslinking. Another impressive feat of secreted mucins is their rapid hydration and swelling, when expelled from the secretory granules of epithelial cells. An acidic and calcium-rich environment keeps the mucins extremely compacted. The fusion of the vesicles with the cell membrane results in ionic exchanges and a sudden expansion of mucins into a hydrogel.6,135 Although not fully understood for mucins, the basic mechanisms were successfully reproduced in vitro using synthetic anionic polymers.136 The delivery of macromolecules in such a compacted format, could protect the molecules from degradation, while keeping the viscosity of the solutions low. This type of system could find useful applications in drug delivery, tissue engineering, and cosmetics.
Exploiting mucins for their bioactivity
A recent increase in interest about mucins’ biological and biophysical properties is leading to a paradigm shift in our perception of mucins. Mucins are not only a passive protective coat on our epithelium but are also bioactive molecules regulating biological processes. In many ways, this shift is similar to the realization that the extracellular matrix in animal tissues acts as a reservoir for biochemical signals that determine, to a large extent, the fate of the cells it embeds. Mucins are key for the bioactivity of the mucus matrix. Mucins can illicit biological responses by binding to bacterial and mammalian cell receptors,137 but can also sequester bioactive molecules such as proteins138 and peptides,139 thus mediating their bioavailability and bioactivity (Fig. 6).
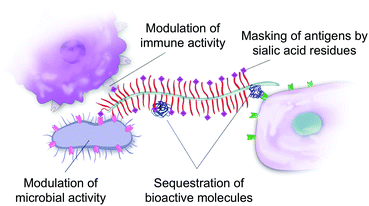 |
| Fig. 6 The bioactivities of mucins. Mucins can illicit biological responses from bacteria and mammalian cells through several mechanisms. Mucin-associated glycans contain ligands to carbohydrate binding surface receptors (such as galectins and siglec). Domains of the protein core can also serve as ligands. Sialic acid residues have a physical role in mediating cell adhesion and shielding antigens from antibody recognition. Mucins and mucin gels can also sequester a number of bioactive molecules, perhaps mediating their activities in ways that are still not understood. | |
The non-glycosylated protein domains of mucins contribute to the intrinsic bioactivity of the mucin molecules. For instance, the mucin epidermal growth factor-like domain (EGF-like) is a cysteine-rich region found in several membrane-tethered mucins. The domain can bind to EGF receptors that are involved in modulating growth, migration and differentiation of cells.140 For instance, the EGF-like region of MUC13 was found to stimulate cell migration, inhibit apoptosis, and accelerate wound healing in the colon.141 The membrane-bound MUC13 was also shown to protect the colonic epithelium by inhibiting toxin-induced apoptosis, perhaps through signaling of the intracellular domain of the mucin.142 The mucins bearing EGF-like domains are membrane-bound, but can also be found in the mucus layer because they are constitutively released from the cells.143 The rich library of glycan structures decorating the mucin molecules are also bioactive. For instance, Kawakubo et al. showed that the terminal alpha1,4-linked N-acetylglucosamines in gastric mucins inhibit the biosynthesis of cholesteryl-alpha-D-glucopyranoside in Helicobacter pylori,144 a causative agent for gastric ulcers and cancer.14,145 A particularly active role of mucin glycans in the regulation of the immune response was recently suggested by a study suggesting that intestinal mucin (MUC2) glycans can induce immune tolerance in dendritic cells13 by binding their galectin-3 receptor proteins. Other sugar residues, such as the terminal sialic acids, modulate both the innate and adaptive immune systems through their interactions with the siglec receptor family.71,146 For instance, siglec receptors binding to sialic acid regulate the B cell tolerance signaling, by mediating their recognition of “self”, with siglec knockout mice developing autoimmune phenotypes. The sialic acid–siglec systems is, for instance, exploited by parasites73,147, bacteria,148,149 viruses,150 and by cancer cells74,151,152 to depend and evade the immune clearance.
In addition to mucin intrinsic signaling, mucins interact with a variety of bioactive proteins and peptides. Several examples already suggest that mucins’ ability to act as reservoirs for bioactive molecules is essential for maintaining an effective mucus barrier.137,153 An example is the association of the intestinal mucus layer with antimicrobial peptides (AMPs) to constitute the body's first line of defence against bacterial infections.154 The antimicrobial spectrum of AMPs is broad, ranging from antifungal and antibacterial, to antiviral activity.155 AMPs are strongly colocalized with mucus, with tens of antimicrobial peptides found in the colonic mucus extracts of healthy individuals156 and mice.157 At neutral pH, many antimicrobial peptides are positively charged, which favors their interactions with the negatively charged membranes of microbes, but also with the negatively charged sulfate and sialic acid residues of mucins. The cathelicidin LL-37 AMP has been shown to electrostatically interact with human airway mucus as well as with porcine gastric mucus and bovine submaxillary mucins, which decreased its antimicrobial activity.139,158 In contrast, human beta-Defensin-3 and the CSA-13 synthetic antimicrobial agent maintained a high level of bactericidal activity in mucin,156,158 even though their antimicrobial action also relies on their positive charges. Similarly, the intestinal MUC2 mucin suppressed the antimicrobial activity of β-defensin 2 against nonpathogenic and enteropathogenic Escherichia coli. However, in vivo, Muc2−/− mice demonstrated reduced expression of β-defensin 2 in the colon, compared with Muc2+/− and Muc2+/+, suggesting that MUC2 regulates the expression and antimicrobial activity of β-defensin 2.159 It, therefore, appears that the physico-chemical properties of the AMPs mediate their interactions with mucins and other extracellular macromolecules and their bioactivity. It is still unknown how the mucus reservoir of AMPs performs under such inhibitory conditions. Nevertheless, their role as bactericidal molecules in mucus could be explained by sudden bursts of AMP secretion that could overcome mucin binding, or the local degradation and release of the AMP by incoming pathogens. In both cases, the mucins would be an essential component of the AMPs’ function.153
Trefoil factors (7–12 kDa) are protease-resistant secretory peptides characterized by one (TFF1 and TFF3) or two trefoil domains (TFF2) expressed by mucosal tissues. TFFs are produced by goblet cells in the gastrointestinal mucosa and are essential for the mucosal healing through signal transduction events, such as the induction of the EGF receptor and the activation of the ERK/MAPK pathway and subsequent prevention of apoptosis. The co-production, co-secretion and co-localization of trefoil factors with mucus and mucins,160,161 imply that they may also be co-regulated and potentially assist each other's functions, jointly promoting the protection and repair of the gastrointestinal mucosa.162 Although the detailed mechanism has not been elucidated, TFFs have been shown to affect the viscoelastic properties of mucus gels. Thim et al. have shown that the addition of TFF2 to pig gastric mucin results in more than a 10-fold increase in viscosity and elasticity.163 The TFF3 dimer increased the viscosity of the mucin solution, while TFF1 and TFF3 monomers had very little effect. Moreover, in the ulcer-associated cell lineage as well as in the gastrointestinal mucosa, each TFF seems to be co-localized with a specific mucin type, hinting that TFFs could act differently when coupled with different mucins.164 Similarly to AMPs, the strong interactions and systematic co-localization could also imply functional synergies between mucins and the TFF molecules.165
Growth factors are also found co-localized with mucins, especially in the gut.166,167 EGF has been of particular interest, since it was shown to upregulate the expression of MUC2 and MU5AC at the transcriptional and protein level168,169 and is involved in mucosal regeneration.170 Although our knowledge on how EGF affects the expression of mucins and cell proliferation is well-established, details regarding the potential interactions between mucins and EGF are scarce. EGF and mucins are co-localized in saliva,171 milk, and in the digestive system.166 In the gut, EGF is thought to act as a surveillance peptide, stimulating mucosal repair, only when the altered epithelial barrier allows access to the basolateral side where the receptors have been localized.172 In this sense, the maintenance of the EGF concentration in the mucus is important, and the mucins–EGF interaction could play a role by decreasing proteolytic activity and helping to maintain a concentration gradient of EGF at the epithelial interface. Thus, the mucus matrix would play a role similar to that of the extracellular matrix (ECM), which mediates cellular processes through the sequestration of growth factors.173
Our understanding of the role of mucins in the modulation of cellular behaviors is still limited, which is why there has been a limited number of attempts at re-creating these effects in engineered systems. Early results obtained with coatings of mucin from commercial sources exposed to neutrophils, did suggest an immunomodulatory effect of mucins. The mucin coatings were found to reduce the adhesion of neutrophils and their secretion of inflammatory markers, such as reactive oxygen species.48,49 However, it is unknown if the decrease was due to the interaction between neutrophils and mucin-bound ligands, or due to differences in adhesion, which is known to be an important regulator of neutrophil activation.174 Originating from a long tradition of using snail mucus for healing purposes,127 mucins extracted from Archachatina maginata snail mucus were tested as a component of drug delivery materials175 and as injectable matrices for treating knee osteoarthritis.176 In this latter study, intramuscular injections of snail mucins seemed to lower the levels of IL-6 and MMP-3 osteoarthritis markers in an experimental model of knee osteoarthritis in dogs. Some inflammation was noticed after prolonged injection, which could be attributed to the relatively crude mucin extraction protocol used. Ovomucins are known to have anti-viral, anti-tumor and immune stimulating properties.106 A recent in vivo study demonstrated the suitability of using ovomucins as part of implantable materials by preparing an ovomucin–gelatin mix system, stabilized by crosslinking with 1-ethyl-3-(3-dimethylaminopropyl)carbodiimide (EDC)177 and implanting it in mice. The in vivo studies showed good infiltration of fibroblasts cells in the hydrogels, most likely due to the gelatin components. Interestingly, the subcutaneous implantation of the gel in rats favored the infiltration of alternatively activated macrophage cells that are favorable for tissue repair and did not trigger any allergic response measured by IgE levels in rat serum. Unfortunately, the study did not compare the mucin-gelatin to gelatin-only materials, which makes it difficult to isolate the contribution of ovomucins to these effects.
What lies ahead
The mucus lining the gastrointestinal tract hosts the billions of bacteria of our gut microbiota. Our understanding of the reciprocal interactions between bacteria and mucus is evolving, with mucins being increasingly recognized as essential modulators of the homeostatic state of these tissues. Therefore, very much like probiotics are administered in the hope of modulating the composition of the microbiota, mucins supplements could help control the composition of bacterial populations in the gut. The addition of mucins or mucin mimics could provide a competitive advantage to certain bacterial species and help modulate the gut microbiome composition and activity.178 As seen above, the co-localization of mucin gels and bioactive proteins and peptides, such as antimicrobial peptides,156 trefoil factors,160 antibodies, and growth factors167 could also point to the existence of interactions and functional synergies between such bioactive components and mucus. Given mucins’ immunomodulating properties, mucins are natural candidates to modify the surface of implantable materials in the hope of improving their biointegration. Although several approaches to surface modifications with mucins have already been reported,48,179,180 more work is needed to generate mucin-based materials that can retain their bioactivity in vivo.
Challenges and opportunities
The development of biopolymer blockbusters has typically started with their discovery in plant or animal tissues, followed by the determination of their structural, physical, chemical, and biological properties. Throughout the process, purification methods are refined, yielding purer and more defined products. In some cases, the combination of high applicative potential, low production costs, and regulatory approval lead to successful commercial products. Examples include hyaluronic acid, first extracted from rooster combs and now used in cosmetic and biomedical products.181,182 Heparin, which is used as anticoagulant, was first isolated from liver tissues while searching for anticoagulant factors in animal tissues.183 Alginates, extracted from algae, have large scale applications as food texturing agents,184 drug encapsulation material,185 and in other niche biomedical applications.186 Chitosans, extracted from the shells of shrimps and calamari, are used as antifungals in agriculture and show promise as scaffold materials for tissue engineering.187
Although the technological potential of mucins seems clear from a functional point of view, some obstacles need to be overcome before translational work can begin. First, mucin research heavily relies on the commercial sources of mucins. The batch to batch variability, the uncertainty as to what protocols are used for purification, and the presence of impurities are challenges that need to be addressed. The development of protocols to further purify commercial mucins,188 protocols to produce native-like mucin on a larger scale,35 and protocols for the quality control of the molecular189,190 and physical properties191 of the mucins will be essential. Challenges around sterilization and conservation of mucin containing products have started to be addressed,115 but could face more challenges if the products are to rely on un-altered protein and glycan structures of the mucins.
Other strategies to produce highly-reproducible mucin materials have been suggested. For instance, one approach is to first simplify the protein or glycan sections of mucins through enzymatic treatments, and then to use this natural backbone to reconstruct the mucins or mucin-mimic in a controlled manner.2 The complementary approach could also be viable, where glycan libraries are first extracted from mucins, then used to decorate a synthetic polymer backbone. This would result in mucin mimics incorporating the unique glycan ligand diversity of native mucins.
There is now a significant body of research focused on the study and engineering of the chemical and physical functionalities of mucins. Scarcer, however, are studies investigating the bioactivity of mucin-materials either in vitro or in vivo. We found seven studies reporting on in vivo testing of mucin materials, providing only initial indications that valuable functionalities could result, but also clearly showing the very early stages of mucin materials for biomedical applications (Table 2). Indeed, most of these early studies do not describe the purification protocols used to obtain mucins. Contaminants co-purified with the mucins can then lead to unwanted side effects. Two more recent experiments performed with purified mucins and implanted in rats and sheep did show encouraging results. In both cases, the investigators found the animals had a low immune reaction to the allogeneic mucin implementation.
Table 2
In vivo studies of mucin or mucin-based materials
Animal model |
Type of mucins implanted |
Implanted material |
Observations |
Ref. |
Mouse, rat |
Pig gastric and salivary |
Co-injected with bacteria |
Inhibition of phagocytosis, antibody uptake and bactericidal action. |
76
|
Sheep |
Bovine salivary mucin |
Chemically grafted or adsorbed on salinized titanium |
Physisorption of mucins is better than covalent linking. Protein activity and bactericidal action are maintained. |
192
|
Showed no sign of toxicity. Formation of cancellous and cortical bone triggered by associated growth factor. |
Rat |
Bovine salivary mucin |
Chemically grafted or adsorbed on poly(L-lactide) |
Mucin prevents platelet adhesion and has weak anticoagulant activity. Covalently bound mucin has superior patency rate and cell infiltration. |
52
|
Rat |
Ovomucin |
Mixed in gelatine hydrogel. |
Better BMSC proliferation and lower inflammation. |
177
|
Dog |
Snail mucin |
Pure. Intramuscular injection. |
IL6 and MMP-3 reduced, but adverse swelling in OA subjects. |
176
|
Rabbit |
Qniumucin |
Pure. Intra articular injection. |
Cartilage degeneration significantly inhibited. |
117
|
Rabbit |
Bovine salivary mucin |
Embedded in PEI multilayer films on PDMS disks |
No or low inflammation at surface of materials. |
57
|
Conclusions
As we better understand the properties of the mucin molecules, it is clear that the mucins are gaining interest as materials. The field, however, is still limited, with 73 articles and patents referenced in this review that investigate the properties and use of mucins and mucin-based materials. This number excludes the growing body of literature dealing with mucin mimics and synthetic mucins. Out of the 73 references, a majority have exploited the barrier properties of mucins (42%), followed by the hydration and lubrication and the chemical properties of mucins (Fig. 7A). Finally, the potential of utilizing the bioactivity of mucin as implantable materials is only in its infancy, with 9% of the referenced publications falling in that category. Although several references cited here are more than 30, even 90 years old, most of the publications are less than 10 years old (Fig. 7B), most likely stimulated by recent improvements in our understanding of mucins and their properties. Further research in mucin-based technologies will contribute to advance our understanding of the mucin molecules, while also leading to new biomimetic approaches to solve important biomedical and chemical problems.
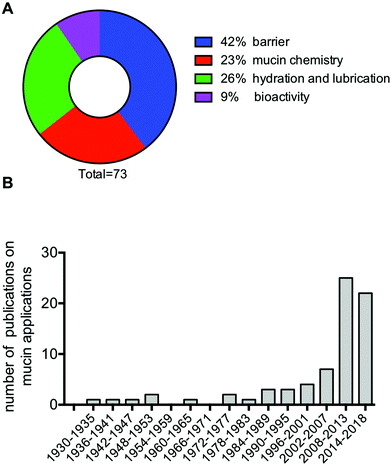 |
| Fig. 7 Thematic classification (A), and distribution by year of publication (B) of the 73 publications referenced in this review that relate to the development of mucin-based technologies. | |
Conflicts of interest
There are no conflicts to declare.
References
- S. Authimoolam and T. Dziubla, Polymers, 2016, 8, 71 CrossRef.
-
U. Kiminori and M. Takeomi, in Studies in Natural Products Chemistry 39, ed. Atta-ur-Rahman, Elsevier, 2013, ch. 3, vol. 39, pp. 115–159 Search PubMed.
- O. Svensson and T. Arnebrant, Curr. Opin. Colloid Interface Sci., 2010, 15, 395–405 CrossRef.
- R. A. Cone, Adv. Drug Delivery Rev., 2008, 61, 75–85 CrossRef PubMed.
- L. M. Ensign, R. Cone and J. Hanes, Adv. Drug Delivery Rev., 2012, 64, 557–570 CrossRef PubMed.
- D. Ambort, M. E. V. Johansson, J. K. Gustafsson, H. E. Nilsson, A. Ermund, B. R. Johansson, P. J. B. Koeck, H. Hebert and G. C. Hansson, Proc. Natl. Acad. Sci. U. S. A., 2012, 109, 5645–5650 CrossRef PubMed.
- N. M. A. Chaudhury, P. Shirlaw, R. Pramanik, G. H. Carpenter and G. B. Proctor, J. Dent. Res., 2015, 94, 1660–1667 CrossRef PubMed.
- J. Celli, B. Gregor, B. Turner, N. H. Afdhal, R. Bansil and S. Erramilli, Biomacromolecules, 2005, 6, 1329–1333 CrossRef PubMed.
- Y. Znamenskaya, J. Sotres, S. Gavryushov, J. Engblom, T. Arnebrant and V. Kocherbitov, J. Phys. Chem. B, 2013, 117, 2554–2563 CrossRef PubMed.
- T. Crouzier, K. Boettcher, A. R. Geonnotti, N. L. Kavanaugh, J. B. Hirsch, K. Ribbeck and O. Lieleg, Adv. Mater. Interfaces, 2015, 2, 1500308 CrossRef.
- L. Ma, A. Gaisinskaya-Kipnis, N. Kampf and J. Klein, Nat. Commun., 2015, 6, 6060 CrossRef PubMed.
- J. M. Coles, D. P. Chang and S. Zauscher, Curr. Opin. Colloid Interface Sci., 2010, 15, 406–416 CrossRef.
- M. Shan, M. Gentile, J. R. Yeiser, A. C. Walland, V. U. Bornstein, K. Chen, B. He, L. Cassis, A. Bigas, M. Cols, L. Comerma, B. Huang, J. M. Blander, H. Xiong, L. Mayer, C. Berin, L. H. Augenlicht, A. Velcich and A. Cerutti, Science, 2013, 342, 447–453 CrossRef PubMed.
- M. Fukuda, M. Kawakubo, Y. Ito, M. Kobayashi, H. Lee and J. Nakayama, Methods Enzymol., 2006, 415, 164–179 Search PubMed.
- R. Bansil and B. S. Turner, Curr. Opin. Colloid Interface Sci., 2006, 11, 164–170 CrossRef.
- C. L. Hattrup and S. J. Gendler, Annu. Rev. Physiol., 2008, 70, 431–457 CrossRef PubMed.
- R. Bansil and B. S. Turner, Curr. Opin. Colloid Interface Sci., 2006, 11, 164–170 CrossRef.
- A. P. Corfield, Biochim. Biophys. Acta, 2015, 1850, 236–252 CrossRef PubMed.
- J. Perez-Vilar and R. L. Hill, J. Biol. Chem., 1999, 274, 31751–31754 CrossRef PubMed.
- H. C. Hang and C. R. Bertozzi, Bioorg. Med. Chem., 2005, 13, 5021–5034 CrossRef PubMed.
- J. Dekker, J. W. A. Rossen, H. A. Büller and A. W. C. Einerhand, Trends Biochem. Sci., 2002, 27, 126–131 CrossRef PubMed.
- T. J. McMaster, M. Berry, A. P. Corfield and M. J. Miles, Biophys. J., 1999, 77, 533–541 CrossRef PubMed.
- A. S. Critchfield, G. Yao, A. Jaishankar, R. S. Friedlander, O. Lieleg, P. S. Doyle, G. McKinley, M. House and K. Ribbeck, PLoS One, 2013, 8, e69528 CrossRef PubMed.
- M. A. McGuckin, S. K. Lindén, P. Sutton and T. H. Florin, Nat. Rev. Microbiol., 2011, 9, 265–278 CrossRef PubMed.
- M. Denny, Nature, 1980, 285, 160–161 CrossRef.
- J. B. Lewis and W. S. Price, J. Zool., 1975, 176, 527–544 CrossRef.
-
J. R. Davies and I. Carlstedt, in Glycoprotein Methods and Protocols, ed. A. P. Corfield, Humana Press, 2000, pp. 3–13 Search PubMed.
- G. Tettamanti and W. Pigman, Arch. Biochem. Biophys., 1968, 124, 41–50 CrossRef PubMed.
- H. D. Hill Jr., J. A. Reynolds and R. L. Hill, J. Biol. Chem., 1977, 252, 3791–3798 Search PubMed.
- V. Schoemig, E. Isik, L. Martin and S. Berensmeier, RSC Adv., 2017, 7, 39708–39717 RSC.
- S. Takehara, M. Yanagishita, K. A. Podyma-Inoue and Y. Kawaguchi, PLoS One, 2013, 8, e69059 CrossRef PubMed.
- J. Kocevar-Nared, J. Kristl and J. Smid-Korbar, Biomaterials, 1997, 18, 677–681 CrossRef PubMed.
- F. Madsen, K. Eberth and J. D. Smart, Pharm. Pharmacol. Commun., 1996, 2, 563–566 Search PubMed.
- T. A. Waigh, A. Papagiannopoulos, A. Voice, R. Bansil, A. P. Unwin, C. D. Dewhurst, B. Turner and N. Afdhal, Langmuir, 2002, 18, 7188–7195 CrossRef.
- V. J. Schömig, B. T. Käsdorf, C. Scholz, K. Bidmon, O. Lieleg and S. Berensmeier, RSC Adv., 2016, 6, 44932–44943 RSC.
- A. Allen, J. P. Pearson, D. A. Hutton, A. H. Mall, R. M. Coan and L. A. Sellers, Symp. Soc. Exp. Biol., 1989, 43, 241–248 Search PubMed.
- M. Scawen and A. Allen, Biochem. J., 1977, 163, 363–368 CrossRef PubMed.
- V. P. Bhavanandan and J. D. Hegarty, J. Biol. Chem., 1987, 262, 5913–5917 Search PubMed.
- J. M. H. Larsson, H. Karlsson, J. G. Crespo, M. E. V. Johansson, L. Eklund, H. Sjövall and G. C. Hansson, Inflammatory Bowel Dis., 2011, 17, 2299–2307 CrossRef PubMed.
- I. Brockhausen, EMBO Rep., 2006, 7, 599–604 CrossRef PubMed.
- L. Arike, J. Holmén-Larsson and G. C. Hansson, Glycobiology, 2017, 27, 318–328 Search PubMed.
- X. Chen, G. S. Lee, A. Zettl and C. R. Bertozzi, Angew. Chem., Int. Ed., 2004, 43, 6111–6116 CrossRef PubMed.
- S. K. Lai, Y.-Y. Wang, K. Hida, R. Cone and J. Hanes, Proc. Natl. Acad. Sci. U. S. A., 2009, 107, 598–603 CrossRef PubMed.
- M. E. V. Johansson, M. Phillipson, J. Petersson, A. Velcich, L. Holm and G. C. Hansson, Proc. Natl. Acad. Sci. U. S. A., 2008, 105, 15064–15069 CrossRef PubMed.
- J. Y. Co, T. Crouzier and K. Ribbeck, Adv. Mater. Interfaces, 2015, 17, 1500179 CrossRef.
- I. A. Bushnak, F. H. Labeed, R. P. Sear and J. L. Keddie, Biofouling, 2010, 26, 387–397 CrossRef PubMed.
- L. Shi, R. Ardehali, K. D. Caldwell and P. Valint, Colloids Surf., B, 2000, 17, 229–239 CrossRef.
- T. Sandberg, J. Carlsson and M. Karlsson Ott, J. Mater. Sci. Mater. Med., 2008, 20, 621–631 CrossRef PubMed.
- T. Sandberg, M. Karlsson Ott, J. Carlsson, A. Feiler and K. D. Caldwell, J. Biomed. Mater. Res., Part A, 2009, 91A, 773–785 CrossRef PubMed.
- T. Crouzier, H. Jang, J. Ahn, R. Stocker and K. Ribbeck, Biomacromolecules, 2013, 14, 3010–3016 CrossRef PubMed.
- Y. M. Thasneem, M. R. Rekha, S. Sajeesh and C. P. Sharma, J. Colloid Interface Sci., 2013, 409, 237–244 CrossRef PubMed.
- R. R. R. Janairo, Y. Zhu, T. Chen and S. Li, Tissue Eng., Part A, 2014, 20, 285–293 CrossRef PubMed.
- B. Winkeljann, B. T. Käsdorf, J. Boekhoven and O. Lieleg, Macromol. Biosci., 2017, 18, 1700311 CrossRef PubMed.
- A. A. Feiler, A. Sahlholm, T. Sandberg and K. D. Caldwell, J. Colloid Interface Sci., 2007, 315, 475–481 CrossRef PubMed.
- H. Ma, J. Hyun, P. Stiller and A. Chilkoti, Adv. Mater., 2004, 16, 338–341 CrossRef.
- M. Malmsten, E. Blomberg, P. Claesson, I. Carlstedt and I. Ljusegren, J. Colloid Interface Sci., 1992, 151, 579–590 CrossRef.
- X. Xu, T. Jin, B. Zhang, H. Liu, Z. Ye, Q. Xu, H. Chen and B. Wang, Polym. Test., 2017/2, 57, 270–280 Search PubMed.
- N. L. Kavanaugh, A. Q. Zhang, C. J. Nobile, A. D. Johnson and K. Ribbeck, mBio, 2014, 5, e01911 CrossRef PubMed.
- M. Caldara, R. S. Friedlander, N. L. Kavanaugh, J. Aizenberg, K. R. Foster and K. Ribbeck, Curr. Biol., 2012, 22, 2325–2330 CrossRef PubMed.
- E. S. Frenkel and K. Ribbeck, Appl. Environ. Microbiol., 2015, 81, 332–338 CrossRef PubMed.
- C. D. Bavington, R. Lever, B. Mulloy, M. M. Grundy, C. P. Page, N. V. Richardson and J. D. McKenzie, Comp. Biochem. Physiol., Part B: Biochem. Mol. Biol., 2004, 139, 607–617 CrossRef PubMed.
- O. Lieleg, C. Lieleg, J. Bloom, C. B. Buck and K. Ribbeck, Biomacromolecules, 2012, 13, 1724–1732 CrossRef PubMed.
- H. H. Habte, G. J. Kotwal, Z. E. Lotz, M. G. Tyler, M. Abrahams, J. Rodriques, D. Kahn and A. S. Mall, Neonatology, 2007, 92, 96–104 CrossRef PubMed.
- D. R. Strombeck and D. Harrold, Infect. Immun., 1974, 10, 1266–1272 Search PubMed.
- C. G. Monferran, G. A. Roth and F. A. Cumar, Infect. Immun., 1990, 58, 3966–3972 Search PubMed.
- A. Gustafsson and J. Holgersson, Expert Opin. Drug Discovery, 2006, 1, 161–178 CrossRef PubMed.
- S. Gaunitz, J. Liu, A. Nilsson, N. Karlsson and J. Holgersson, Glycoconjugate J., 2014, 31, 145–159 CrossRef PubMed.
- R. M. Cherian, C. Jin, J. Liu, N. G. Karlsson and J. Holgersson, Infect. Immun., 2016, 4, 2842–2852 CrossRef PubMed.
- S. P. Galuska, C. E. Galuska, T. Tharmalingam, K. Zlatina, G. Prem, F. C. O. Husejnov, P. M. Rudd, W. F. Vann, C. Reid, J. Vionnet, M. E. Gallagher, F. A. Carrington, S.-L. Hassett and S. D. Carrington, FEBS J., 2017, 28, 1688–1699 CrossRef PubMed.
- L. Li, O. Lieleg, S. Jang, K. Ribbeck and J. Han, Lab Chip, 2012, 12, 4071–4079 RSC.
- A. Varki and P. Gagneux, Ann. N. Y. Acad. Sci., 2012, 1253, 16–36 CrossRef PubMed.
- R. Schauer, Trends Biochem. Sci., 1985, 10, 357 CrossRef.
- V. L. Pereira-Chioccola, A. Acosta-Serrano, I. Correia de Almeida, M. A. Ferguson, T. Souto-Padron, M. M. Rodrigues, L. R. Travassos and S. Schenkman, J. Cell Sci., 2000, 113(Pt 7), 1299–1307 Search PubMed.
- M. A. Hollingsworth and B. J. Swanson, Nat. Rev. Cancer, 2004, 4, 45–60 CrossRef PubMed.
- A. F. Zuluaga, B. E. Salazar, M. Agudelo, C. A. Rodriguez and O. Vesga, J. Biomed. Sci., 2015, 22, 24 CrossRef PubMed.
- L. Olitzki, Bacteriol. Rev., 1948, 12, 149–172 Search PubMed.
- W. J. Nungester and L. F. Jourdonais, J. Infect. Dis., 1936, 59, 258–265 CrossRef.
- J. Clemmesen and K. Andersen, Acta Pathol. Microbiol. Scand., 1942, 19, 173–183 CrossRef.
- R. E. Strauss and A. M. Kligman, J. Infect. Dis., 1951, 88, 151–155 CrossRef PubMed.
- W. Sims, J. Gen. Microbiol., 1964, 37, 335–340 CrossRef PubMed.
- R. Tunnicliff, J. Infect. Dis., 1940, 66, 189–191 CrossRef.
- K. M. Loomes, H. E. Senior, P. M. West and A. M. Roberton, Eur. J. Biochem., 1999, 266, 105–111 CrossRef PubMed.
- S. J. Fogelson, Exp. Biol. Med., 1930, 28, 138–138 CrossRef.
- M. Tatematsu, M. Takahashi, M. Hananouchi, T. Shirai and M. Hirose, Gan, 1976, 67, 223–229 CrossRef PubMed.
- V. Gouyer, L. Dubuquoy, C. Robbe-Masselot, C. Neut, E. Singer, S. Plet, K. Geboes, P. Desreumaux, F. Gottrand and J.-L. Desseyn, Sci. Rep., 2015, 5, 9577 CrossRef PubMed.
- C. Ehre, E. N. Worthington, R. M. Liesman, B. R. Grubb, D. Barbier, W. K. O'Neal, J.-M. Sallenave, R. J. Pickles and R. C. Boucher, Proc. Natl. Acad. Sci. U. S. A., 2012, 109, 16528–16533 CrossRef PubMed.
-
USPTO, US Patent, 5104662, 1992 Search PubMed.
- A. P. Gunning, A. R. Kirby, C. Fuell, C. Pin, L. E. Tailford and N. Juge, FASEB J., 2013, 27, 2342–2354 CrossRef PubMed.
- T. Crouzier, C. H. Beckwitt and K. Ribbeck, Biomacromolecules, 2012, 13, 3401–3408 CrossRef PubMed.
- R. Polak, T. Crouzier, R. M. Lim, K. Ribbeck, M. M. Beppu, R. N. M. Pitombo, R. E. Cohen and M. F. Rubner, Biomacromolecules, 2014, 15, 3093–3098 CrossRef PubMed.
- C. V. Duffy, L. David and T. Crouzier, Acta Biomater., 2015, 20, 51–59 CrossRef PubMed.
- A. Serafim, E. Olaret, S. Cecoltan, L. M. Butac, B. Balanuca, E. Vasile, M. Ghica and I. C. Stancu, Mater. Plast., 2018, 55, 68 Search PubMed.
- C. Nowald, A. Penk, H.-Y. Chiu, T. Bein, D. Huster and O. Lieleg, Macromol. Biosci., 2016, 16, 567–579 CrossRef PubMed.
- Y. Fukui, M. Fukuda and K. Fujimoto, J. Mater. Chem. B, 2018, 6, 781–788 RSC.
- B. Belgorodsky, E. Drug, L. Fadeev, N. Hendler, E. Mentovich and M. Gozin, Small, 2010, 6, 262–269 CrossRef PubMed.
- E. Drug, D. Landesman-Milo, B. Belgorodsky, N. Ermakov, M. Frenkel-Pinter, L. Fadeev, D. Peer and M. Gozin, Chem. Res. Toxicol., 2011, 24, 314–320 Search PubMed.
- N. Barbero, M. Marenchino, R. Campos-Olivas, S. Oliaro-Bosso, L. Bonandini, J. Boskovic, G. Viscardi and S. Visentin, J. Nanopart. Res., 2016, 18, 1–8 CrossRef.
- A. Patwa, A. Thiéry, F. Lombard, M. K. S. Lilley, C. Boisset, J.-F. Bramard, J.-Y. Bottero and P. Barthélémy, Sci. Rep., 2015, 5, 11387 CrossRef PubMed.
- N. Hendler, B. Belgorodsky, E. D. Mentovich, M. Gozin and S. Richter, Adv. Mater., 2011, 23, 4261–4264 CrossRef PubMed.
- N. Shraga, B. Belgorodsky and M. Gozin, J. Am. Chem. Soc., 2009, 131, 12074–12075 CrossRef PubMed.
- N. Hendler, L. Fadeev, E. D. Mentovich, B. Belgorodsky, M. Gozin and S. Richter, Chem. Commun., 2011, 47, 7419–7421 RSC.
-
USPTO, US Patent, 8795746, 2014 Search PubMed.
-
USPTO, US Patent, 20020099005A1, 2002 Search PubMed.
-
USPTO, US Patent, 20080286211A1, 2008 Search PubMed.
- M. H. Vingerhoeds, T. B. J. Blijdenstein, F. D. Zoet and G. A. van Aken, Food Hydrocolloids, 2005/9, 19, 915–922 Search PubMed.
- D. A. Omana, J. Wang and J. Wu, Trends Food Sci. Technol., 2010/9, 21, 455–463 Search PubMed.
- L. Böni, P. A. Rühs, E. J. Windhab, P. Fischer and S. Kuster, PLoS One, 2016, 11, e0147022 CrossRef PubMed.
- S. A. Payment, B. Liu, G. D. Offner, F. G. Oppenheim and R. F. Troxler, J. Dent. Res., 2000, 79, 1765–1772 CrossRef PubMed.
- T. Nederfors, Adv. Dent. Res., 2000, 14, 48–56 CrossRef PubMed.
- A. J. Duxbury, N. S. Thakker and D. G. Wastell, Br. Dent. J., 1989, 166, 115–120 CrossRef PubMed.
- A. Vissink, E. J. s-Gravenmade, A. K. Panders, A. Vermey, J. K. Petersen, L. L. Visch and R. M. Schaub, Int. J. Oral Surg., 1983, 12, 232–238 CrossRef PubMed.
- L. L. Visch, E. J. ‘s-Gravenmade, R. M. H. Schaub, W. L. J. Van Putten and A. Vissink, Int. J. Oral Maxillofac. Surg., 1986, 15, 395–400 CrossRef PubMed.
- E. J. ‘s-Gravenmade and A. Vissink, Oral Surg., Oral Med., Oral Pathol, 1993, 75, 466–471 CrossRef.
- M. P. Sweeney, J. Bagg, W. P. Baxter and T. C. Aitchison, Palliat. Med., 1997, 11, 225–232 CrossRef PubMed.
-
US, US Patent, 4438100A, 1984 Search PubMed.
- G. Blixt-Johansen, K. Sjöholm, K. Wiesel and A. C. Ek, Scand. J. Caring Sci., 1992, 6, 147–150 CrossRef PubMed.
- N. Ohta, M. Sato, K. Ushida, M. Kokubo, T. Baba, K. Taniguchi, M. Urai, K. Kihira and J. Mochida, BMC Biotechnol., 2009, 9, 98 CrossRef PubMed.
- B. Winkeljann, K. Boettcher, B. N. Balzer and O. Lieleg, Adv. Mater. Interfaces, 2017, 1700186 CrossRef.
- K. A. Waller, L. X. Zhang, K. A. Elsaid, B. C. Fleming, M. L. Warman and G. D. Jay, Proc. Natl. Acad. Sci. U. S. A., 2013, 110, 5852–5857 CrossRef PubMed.
- J.-P. Bao, W.-P. Chen and L.-D. Wu, Mol. Biol. Rep., 2011, 38, 2879–2885 CrossRef PubMed.
- J. P. Gleghorn, A. R. C. Jones, C. R. Flannery and L. J. Bonassar, J. Orthop. Res., 2009, 27, 771–777 CrossRef PubMed.
- C. R. Flannery, R. Zollner, C. Corcoran, A. R. Jones, A. Root, M. A. Rivera-Bermúdez, T. Blanchet, J. P. Gleghorn, L. J. Bonassar, A. M. Bendele, E. A. Morris and S. S. Glasson, Arthritis Rheum., 2009, 60, 840–847 CrossRef PubMed.
- K. Boettcher, B. Winkeljann, T. A. Schmidt and O. Lieleg, Biotribology, 2017, 12, 43–51 CrossRef.
- Y. Znamenskaya, J. Sotres, J. Engblom, T. Arnebrant and V. Kocherbitov, J. Phys. Chem. B, 2012, 116, 5047–5055 CrossRef PubMed.
- T. Yamashita, R. Shimura, M. Oyobikawa and S. Ookura, J. Soc. Cosmet. Chem. Jpn., 1994, 27, 573–579 CrossRef.
-
USPTO, US Patent, 7829679, 2010 Search PubMed.
- B. Bonnemain, J. Evidence-Based Complementary Altern. Med., 2005, 2, 25–28 CrossRef PubMed.
- A. R. de Toledo-Piza and D. A. Maria, Adv. Skin Wound Care, 2014, 27, 538–547 CrossRef PubMed.
- D. Tsoutsos, D. Kakagia and K. Tamparopoulos, J. Dermatol. Treat., 2009, 20, 219–222 CrossRef PubMed.
- G. E. Yakubov, J. McColl, J. H. H. Bongaerts and J. J. Ramsden, Langmuir, 2009, 25, 2313–2321 CrossRef PubMed.
- I. Svanberg, J. Ethnobiol., 2006, 26, 299–309 CrossRef.
-
USPTO, US Patent, 6281192, 2001 Search PubMed.
- S. Woodward, Br. J. Nurs., 2005, 14, 1022–1023 CrossRef PubMed.
- Y. Hashimoto, S. Tsuiki, K. Nisizawa and W. Pigman, Ann. N. Y. Acad. Sci., 1963, 106, 233–246 CrossRef PubMed.
-
P. Verdugo, I. Deyrup-Olsen, A. W. Martin and D. L. Luchtel, in Mechanics of Swelling, Springer, Berlin Heidelberg, 1992, pp. 671–681 Search PubMed.
- P. F. Kiser, G. Wilson and D. Needham, Nature, 1998, 394, 459–462 CrossRef PubMed.
- S. Senapati, S. Das and S. K. Batra, Trends Biochem. Sci., 2010, 35, 236–245 CrossRef PubMed.
- B. L. Slomiany, Z. Fekete, V. L. Murty, M. Grabska, J. Piotrowski, F. Yotsumoto, A. Czajkowski and A. Slomiany, Int. J. Biochem., 1993, 25, 1281–1289 CrossRef PubMed.
- K. Felgentreff, C. Beisswenger, M. Griese, T. Gulder, G. Bringmann and R. Bals, Peptides, 2006, 27, 3100–3106 CrossRef PubMed.
- Y. Yarden and M. X. Sliwkowski, Nat. Rev. Mol. Cell Biol., 2001, 2, 127–137 CrossRef PubMed.
- S. B. Ho, L. A. Dvorak, R. E. Moor, A. C. Jacobson, M. R. Frey, J. Corredor, D. B. Polk and L. L. Shekels, Gastroenterology, 2006, 131, 1501–1517 CrossRef PubMed.
- Y. H. Sheng, R. Lourie, S. K. Lindén, P. L. Jeffery, D. Roche, T. V. Tran, C. W. Png, N. Waterhouse, P. Sutton, T. H. J. Florin and M. A. McGuckin, Gut, 2011, 60, 1661–1670 CrossRef PubMed.
- T. D. Blalock, S. J. Spurr-Michaud, A. S. Tisdale and I. K. Gipson, Invest. Ophthalmol. Visual. Sci., 2008, 49, 1864–1871 CrossRef PubMed.
- M. Kawakubo, Y. Ito, Y. Okimura, M. Kobayashi, K. Sakura, S. Kasama, M. N. Fukuda, M. Fukuda, T. Katsuyama and J. Nakayama, Science, 2004, 305, 1003–1006 CrossRef PubMed.
- T. L. Testerman and J. Morris, World J. Gastroenterol., 2014, 20, 12781–12808 CrossRef PubMed.
- P. R. Crocker and A. Varki, Trends Immunol., 2001, 22, 337–342 CrossRef PubMed.
- T. Jacobs, H. Erdmann and B. Fleischer, Eur. J. Cell Biol., 2010, 89, 113–116 CrossRef PubMed.
- E. Severi, D. W. Hood and G. H. Thomas, Microbiology, 2007, 153, 2817–2822 CrossRef PubMed.
- A. F. Carlin, S. Uchiyama, Y.-C. Chang, A. L. Lewis, V. Nizet and A. Varki, Blood, 2009, 113, 3333–3336 CrossRef PubMed.
- J. C. Paulson, M. S. Macauley and N. Kawasaki, Ann. N. Y. Acad. Sci., 2012, 1253, 37–48 CrossRef PubMed.
- C. Büll, M. H. den Brok and G. J. Adema, Biochim. Biophys. Acta, 2014, 1846, 238–246 Search PubMed.
- O. M. T. Pearce and H. Läubli, Glycobiology, 2016, 26, 111–128 CrossRef PubMed.
- A. Dupont, L. Heinbockel, K. Brandenburg and M. W. Hornef, Gut Microbes, 2014, 5, 761–765 CrossRef PubMed.
- E. Guaní-Guerra, T. Santos-Mendoza, S. O. Lugo-Reyes and L. M. Terán, Clin. Immunol., 2010, 135, 1–11 CrossRef PubMed.
- H. Jenssen, P. Hamill and R. E. W. Hancock, Clin. Microbiol. Rev., 2006, 19, 491–511 CrossRef PubMed.
- L. Antoni, S. Nuding, D. Weller, M. Gersemann, G. Ott, J. Wehkamp and E. F. Stange, J. Crohns. Colitis, 2013, 7, e652–e664 CrossRef PubMed.
- U. Meyer-Hoffert, M. W. Hornef, B. Henriques-Normark, L.-G. Axelsson, T. Midtvedt, K. Pütsep and M. Andersson, Gut, 2008, 57, 764–771 CrossRef PubMed.
- R. Bucki, D. B. Namiot, Z. Namiot, P. B. Savage and P. A. Janmey, J. Antimicrob. Chemother., 2008, 62, 329–335 CrossRef PubMed.
- E. R. Cobo, V. Kissoon-Singh, F. Moreau and K. Chadee, Mucosal Immunol., 2015, 8, 1360–1372 CrossRef PubMed.
- A. Wiede, W. Jagla, T. Welte, T. Köhnlein, H. Busk and W. Hoffmann, Am. J. Respir. Crit. Care Med., 1999, 159, 1330–1335 CrossRef PubMed.
- J. Madsen, O. Nielsen, I. Tornøe, L. Thim and U. Holmskov, J. Histochem. Cytochem., 2007, 55, 505–513 CrossRef PubMed.
- D. Taupin and D. K. Podolsky, Nat. Rev. Mol. Cell Biol., 2003, 4, 721–732 CrossRef PubMed.
- L. Thim, F. Madsen and S. S. Poulsen, Eur. J. Clin. Invest., 2002, 32, 519–527 CrossRef PubMed.
- R. J. Longman, J. Douthwaite, P. A. Sylvester, R. Poulsom, A. P. Corfield, M. G. Thomas and N. A. Wright, Gut, 2000, 47, 792–800 CrossRef PubMed.
- H. Kindon, C. Pothoulakis, L. Thim, K. Lynch-Devaney and D. K. Podolsky, Gastroenterology, 1995, 109, 516–523 CrossRef.
- M. S. Murphy, Nutrition, 1998, 14, 771–774 CrossRef PubMed.
- B. Dubiel, B. Mytar, A. Tarnawski, M. Zembala and J. Stachura, J. Physiol. Pharmacol., 1992, 43, 21–32 Search PubMed.
- M. Perrais, P. Pigny, M.-C. Copin, J.-P. Aubert and I. Van Seuningen, J. Biol. Chem., 2002, 277, 32258–32267 CrossRef PubMed.
- K. Takeyama, K. Dabbagh, H. M. Lee, C. Agustí, J. A. Lausier, I. F. Ueki, K. M. Grattan and J. A. Nadel, Proc. Natl. Acad. Sci. U. S. A., 1999, 96, 3081–3086 CrossRef.
- M. K. Jones, M. Tomikawa, B. Mohajer and A. S. Tarnawski, Front. Biosci., 1999, 4, D303–D309 CrossRef.
- M. McGurk, L. Hanford, S. Justice and R. A. Metcalfe, Arch. Oral Biol., 1990, 35, 653–659 CrossRef PubMed.
- R. J. Playford, A. M. Hanby, S. Gschmeissner, L. P. Peiffer, N. A. Wright and T. McGarrity, Gut, 1996, 39, 262–266 CrossRef PubMed.
- R. O. Hynes, Science, 2009, 326, 1216–1219 CrossRef PubMed.
- G. Berton, S. R. Yan, L. Fumagalli and C. A. Lowell, Int. J. Clin. Lab. Res., 1996, 26, 160–177 CrossRef PubMed.
- F. C. Kenechukwu, E. C. Ibezim, A. A. Attama, M. A. Momoh, J. D. N. Ogbonna, P. O. Nnamani, S. A. Chime, C. E. Umeyor and E. M. Uronnachi, Afr. J. Biotechnol., 2013, 12, 6661–6671 CrossRef.
- A. R. Ajadi, O. Gazal, E. Otesile and O. Kasali, Int. J. Morphol., 2013, 31, 280–286 CrossRef.
- N. T. Carpena, C. D. G. Abueva, A. R. Padalhin and B.-T. Lee, J. Biomed. Mater. Res., Part B Appl. Biomater., 2016, 105, 2107–2117 CrossRef PubMed.
-
USPTO, US Patent, 20160228507:A1, 2016 Search PubMed.
- T. Sandberg, H. Blom and K. D. Caldwell, J. Biomed. Mater. Res., Part A, 2009, 91, 762–772 CrossRef PubMed.
- T. Sandberg, M. Karlsson Ott and J. Carlsson, J. Biomed. Mater. Res., Part A, 2009, 91, 773–785 CrossRef PubMed.
- G. Kogan, L. Soltés, R. Stern and P. Gemeiner, Biotechnol. Lett., 2007, 29, 17–25 CrossRef PubMed.
-
M. A. Selyanin, P. Y. Boykov, V. N. Khabarov and F. Polyak, in Hyaluronic Acid, John Wiley & Sons, Ltd, 2015, pp. 1–8 Search PubMed.
- D. Wardrop and D. Keeling, Br. J. Haematol., 2008, 141, 757–763 CrossRef PubMed.
-
A. P. Imeson, Thickening and gelling agents for food, Springer US, 1992 Search PubMed.
- D. Jain and D. Bar-Shalom, Drug Dev. Ind. Pharm., 2014, 40, 1576–1584 CrossRef PubMed.
- K. Y. Lee and D. J. Mooney, Prog. Polym. Sci., 2012, 37, 106–126 CrossRef PubMed.
- M. Rinaudo, Prog. Polym. Sci., 2006/7, 31, 603–632 Search PubMed.
- J. B. Madsen, K. I. Pakkanen, L. Duelund, B. Svensson, M. A. Hachem and S. Lee, Prep. Biochem. Biotechnol., 2015, 45, 84–99 CrossRef PubMed.
- K. A. Ramsey, Z. L. Rushton and C. Ehre, J. Visualized Exp., 2016, 2016(112), e54153 Search PubMed.
-
A. P. Corfield, Glycoprotein Methods and Protocols: The Mucins, Humana Press, 2000 Search PubMed.
- J. P. Celli, B. S. Turner, N. H. Afdhal, R. H. Ewoldt, G. H. McKinley, R. Bansil and S. Erramilli, Biomacromolecules, 2007, 8, 1580–1586 CrossRef PubMed.
-
M. Larsson, Master, Uppsala University, 2012 Search PubMed.
Footnote |
† Electronic supplementary information (ESI) available. See DOI: 10.1039/c8bm00471d |
|
This journal is © The Royal Society of Chemistry 2018 |
Click here to see how this site uses Cookies. View our privacy policy here.