DOI:
10.1039/C8AN00233A
(Paper)
Analyst, 2018,
143, 3708-3721
In vitro antibacterial activity of oxide and non-oxide bioceramics for arthroplastic devices: I. In situ time-lapse Raman spectroscopy
Received
6th February 2018
, Accepted 14th June 2018
First published on 15th June 2018
Abstract
Over the next two decades, a strong demographic demand for arthroplastic devices coupled with a decreased efficacy of antibiotics has been predicted to result in an exponential increase in the number of periprosthetic joint infections (PJIs). Advanced strategies are therefore required to improve the local peri-implant immune response and curb the pathogenic events of bacterial adhesion and biofilm formation. The use of biomaterials that autonomously counter infections is one approach to improve orthopedic outcomes. Using conventional molecular biology characterization methods and advanced Raman spectroscopy, this study examined the bacteriostatic response of two bioceramic materials commonly employed as prosthetic implants: zirconia-toughened alumina (ZTA) and silicon nitride (Si3N4). Unlike the ZTA, it was found that non-oxide Si3N4 possesses an inherently anti-infective surface chemistry, which acts in a responsive way against bacterial loading. The mechanistic details of its behavior are elucidated. Non-oxide bioceramics appear to be promising, but their full development requires a transitional approach that integrates the fundamental biochemical concepts with clinical outcomes.
1. Introduction
Periprosthetic infections result from complex interactions among the host, the pathogen, and the implant.1–3 Infections originate from isolated prokaryotic cells which initially adhere to the implant's surface forming a quorum,4 followed by the generation of a biofilm which shelters the development of an organized complex community representing multicellular organisms.5 The biofilm protects bacteria from antimicrobial agents and host immune responses. In contrast to their planktonic predecessors, bacteria enclosed in a mature biofilm are quite stable and resistant to elimination.6 Post-operatively, the presence of the abiotic implant impairs the local immune response. Neutrophils release peptides resulting in the deactivation of granulocytes;7 their deactivation has the effect of gradually reducing the minimum amount of microbes required to initiate a septic condition.8 Consequently, an enhanced risk of infection persists for the entire lifetime of the implant.9 The most frequently reported bacteria responsible for periprosthetic joint infections (PJIs) are Gram-positive cocci, most commonly Staphylococcus aureus and Staphylococcus epidermidis.10–12
The Staphylococcus genus has developed an antibiotic resistance to certain classes of drugs (e.g., rifampicin) requiring the use of companion medications such as fluoroquinolones (e.g., ciprofloxacin) to be effective.13 However, the utility of this combination has recently diminished due to the growing resistance of the genus against fluoroquinolones as well.14 Alternative drugs have been employed, including fusidic acid, trimethoprim-sulfamethoxazole, minocycline, daptomycin and linezolid.15–19 Kim et al.20 have recently reported about the effectiveness of a new class of synthetic retinoid antibiotics effective against bacterial persisters. Yet, idiopathic comor bidities associated with the use of these compounds limit their broad usage. Consequently, the best conceivable solution today is prevention rather than treatment of infections.
Efforts have thus been directed along two parallel paths: (i) the design of implants that slowly elute antibacterial agents (e.g., vancomycin, amoxicillin, gentamicin, silver, zinc, copper, iodine and so forth)21–25 and (ii) the design of implants with inherently “smart” surfaces that are capable of counteracting bacterial adhesion and biofilm formation.26–31 The advantage of an implant that elutes a drug resides in the fact that localized antibiotic penetration into the biofilm can be up to 1000 times more efficient than oral or parenteral administration.32 As typical examples, methyl methacrylate and ceramic bone cements containing a broad spectrum of antibiotics have been used to create a local antimicrobial periprosthetic environment.33,34 However, the uncontrolled release of antibiotics and possibly reduced fixation represent the tangible shortcomings of this method.35 Regarding biofilm resistant surfaces, one widely employed method has been the use of titanium surfaces that release bactericidal superoxide radicals from the TiO2 passivation layer; although this requires UV irradiation to be fully activated.36 Unlike bulk silver and Ag-containing biomaterials, which are also well-known antibacterial agents,37 TiO2 surfaces do not induce significant cytotoxic effects on mammalian cells38 unless they release nanoparticles in high concentrations (e.g., upon wear).39 In contrast to the plethora of experimental and clinical literature studies on active materials that elute bactericidal compounds or contact biocides and passively activated surfaces,27,30 the antibacterial properties of bulk bioceramics are less well-known.
In this paper, the in vitro antibacterial performances of two popular bioceramics – oxide ZTA and non-oxide Si3N4 – are compared using Staphylococcus epidermidis (S. epidermidis). The impetus to conduct this work was based on several previous publications including: (i) a series of in vitro and in vivo studies on non-oxide Si3N4 ceramics40–43 which showed significant resistance to several bacilli; and (ii) a published survey by Kurtz et al. which statistically showed a reduced incidence of infection from alumina (Al2O3)-based bioceramics in Medicare patients.44 A previously published in vitro study on Si3N4 bioceramic indicated the possibility of an intrinsic (chemistry-related) mechanism for its bacteriostatic behavior including the formation of peroxynitrite as a selective oxidative agent.40 A study by Kurtz et al. revealed a reduced risk of readmission for patients implanted with Al2O3-based femoral heads. The authors of the survey interpreted the statistical results as supporting their initial hypothesis that oxide ceramic bearings improve certain clinical outcomes after revision surgery including infection. Accordingly, the purposes of this paper are: (i) to compare for the first time the antibacterial performance of Al2O3-based (oxide) and Si3N4 (non-oxide) ceramics in the same series of biological tests, (ii) to quantitatively assess their respective abilities to inhibit biofilm formation, and (iii) to propose mechanisms for their observed bacteriostatic behaviors.
2. Experimental procedures
2.1 Investigated samples
The Si3N4 employed in this study was a commercially available bulk bioceramic from Amedica Corporation (MC2®; Salt Lake City, UT, USA). Details of its processing and basic properties have been published previously.45,46 Briefly, this biomaterial has a nominal composition of 90 wt% Si3N4, 6 wt% Y2O3, and 4 wt% Al2O3; the two latter compounds are necessary additives for densification. These constituents and a minor fraction of native silica (SiO2) react with the bulk Si3N4 grains during sintering to form an intergranular partially crystalline SiYAlON oxynitride phase. The dense bioceramic consists of about 90 vol% crystalline Si3N4 and 10 vol% SiYAlON. Identical disc samples (∅ 12.7 × 1 mm) from the same Si3N4 powder lot were pressed, densified, polished, and subsequently used for biological and in situ spectroscopic testing. ZTA disks of the same geometry were obtained by machining and finely polishing BIOLOX®delta femoral head components (CeramTec, GmbH, Plochingen, Germany). This ZTA contains approximately 80 vol% Al2O3, 17 vol% ZrO2, and 3 vol% chromium and strontium oxides. During reactive sintering, a small fraction of strontium aluminate is generated within the microstructure and chromium preponderantly enters the Al2O3 lattice forming a solid solution.47 Polyetheretherketone (PEEK Optima® Invibio, West Conshohocken, PA, USA) samples of the same geometry were also prepared and employed as a negative control. Although PEEK is widely used as a structural biomaterial, it is known to possess poor bacteriostatic properties.41–44,48 The PEEK samples were also finely polished to roughness values similar to the Si3N4 and ZTA components, ranging from 10–100 nm Ra. All samples were UV-C sterilized (254 nm, Phillips, Denver, CO, USA) for 30 min prior to biological testing.
2.2 Bacterial culture and biological tests
Freeze-dried pellets of S. epidermidis (ATCC™ 14990®) were hydrated in heart infusion (HI) broth (Nissui, Tokyo, Japan) and incubated at 37 °C for 18 hours in brain heart infusion (BHI) agar (Nissui). The mixture was subsequently assayed for colony forming units (CFU) and diluted to a concentration of 1 × 108 CFU ml−1 using phosphate-buffered saline (PBS). An aliquot of 100 μl of the bacterial suspension was spread onto individual BHI agar plates. The Si3N4, ZTA, and PEEK samples were then placed in contact with the agar for inoculation purposes, followed by incubation at 37 °C under aerobic conditions for 8, 12, 24, or 48 hours.
At each time point, the test and control samples were observed by fluorescence microscopy (BZ-X700; Keyence, Osaka, Japan). For visualization, bacteria were stained with two different solutions: (i) 4′,6-diamidino-2-phenylindole (DAPI) which binds to and stains DNA blue thereby imaging the nucleus location and (ii) Sytox® Green nucleic acid, which stains only dead bacteria green but is impermeable to live cells. Nitric oxide (NO) real-time activity within living bacteria was monitored by fluorescence imaging using a membrane permeable fluorescent indicator DAF-2(NO) (i.e., diaminofluorescein-2 diacetate, Goryo Chemical, Inc., Sapporo, Japan). Once inside the bacteria, this substance was deacetylated via intracellular esterase and it was detected with an excitation/emission maximum of 495/515 nm. Experiments using DAF-2 were performed in a dark room because the dye is light sensitive. Then, the samples were transferred into a chamber on the stage of the fluorescence microscope and intensities of DAF-2 were determined by in situ confocal microscopy using a 488 nm Krypton/Argon laser as the excitation source.
2.3
In situ Raman spectroscopy
In situ Raman spectra were collected using a highly sensitive instrument (LabRAM HR800, Horiba/Jobin–Yvon, Kyoto, Japan) with a 20× optical lens. This spectroscope operated in microscopic measurement mode with confocal imaging in two dimensions. A holographic notch filter within the optical circuit was used to efficiently achieve high-resolution spectral acquisitions.49 A spectral resolution of 1.5 cm−1 was obtained using a 532 nm excitation source operating at 10 mW. The Raman emission was monitored by using a single monochromator connected to an air-cooled charge-coupled device (CCD) detector (Andor DV420-OE322; 1024 × 256 pixel). The acquisition time was fixed at 10 s. Thirty spectra were collected and averaged at each analysis time-point. Raman spectra were deconvoluted into Gaussian–Lorentzian sub-bands using commercially available software (LabSpec 4.02, Horiba/Jobin–Yvon, Kyoto, Japan).
2.4 Statistical analysis
The unpaired Student's t-test was utilized for statistical analysis. In the Results section, the sample size, n, for each experiment is stipulated in the figure's insets. A p value <0.05 was considered statistically significant and labeled with two asterisks. Values of p > 0.05 were considered statistically insignificant and labeled “n.s.”.
3. Experimental results
3.1 Molecular biology response of oxide vs. non-oxide bioceramics
Fig. 1 shows the merged DAPI (blue) and Sytox® (green) fluorescence images for S. epidermidis after 12 and 48 h of exposure to the Si3N4, ZTA, and PEEK substrates. Bacteria exposed to the Si3N4 substrate increasingly and preponderantly stained green (cf.Fig. 1(a) and (b)) with exposure time. The green stain indicates the presence of dead bacteria. In contrast, the bacteria on PEEK substrates stained predominately blue (cf.Fig. 1(e) and (f)). The blue stain indicates the presence of live and active bacilli. The behavior of the bacteria on the ZTA substrates was intermediate between the Si3N4 and PEEK biomaterials (cf.Fig. 1(c) and (d)). Some green spots were apparent in the fluorescence micrographs after both 12 and 24 h but living bacteria were also detected. Fig. 2 provides a quantitative assessment of the presence of living bacteria found on each substrate material along with their corresponding statistical inferences. These data were obtained using >12 fluorescence micrographs for each sample and n = 4 samples for each substrate. The results revealed an exponential increase in living bacteria for both PEEK and ZTA, with the latter increase being less pronounced than the former. In contrast, living bacteria on the surfaces of Si3N4 underwent an initial increase up to 24 h, and then decreased (with statistical validity) after 48 h. The outcomes of the DAPI/Sytox® stain experiments pointed to the presence of an intrinsic mechanism counteracting S. epidermidis proliferation on the Si3N4 samples. This mechanism was obviously not available to either the PEEK or ZTA substrates.
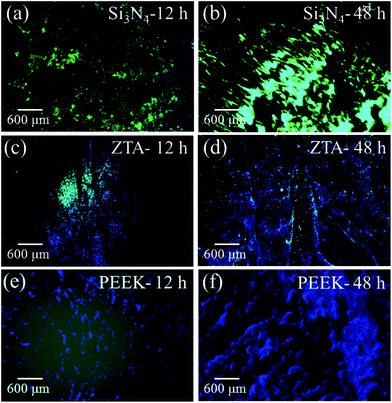 |
| Fig. 1 Merged DAPI (blue) and Sytox® (green) fluorescence images of S. epidermidis after 12 and 48 h exposure to Si3N4 ((a) and (b)), ZTA ((c) and (d)), and PEEK ((e) and (f)) substrates. | |
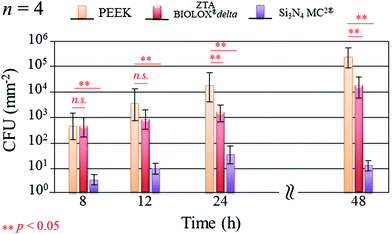 |
| Fig. 2 Quantitative assessment of living bacteria proliferation and its statistical validation based on the analysis of fluorescence micrographs covering the entire samples (n = 4 per substrate type). | |
Fig. 3 presents the results of time-lapse fluorescence spectroscopy on DAF-2(NO)-stained S. epidermidis on the Si3N4 ((a), (b), and (c)) and ZTA bioceramics ((d), (e), and (f)) for 12, 24, and 48 h, respectively. For bacteria exposed to the Si3N4 samples, note that its nitric oxide (NO) concentration suddenly increased after 24 h. Furthermore, bacteria on the Si3N4 surfaces displayed few NO green-stain pixels after 48 h. No similar NO acquisition trend was observed for the ZTA substrates; only a moderate increase in green pixels could be seen up to 48 h. The statistically validated results (n = 4) for these time-lapse experiments are plotted in Fig. 4. A NO peak for bacteria on the Si3N4 substrates at intermediate testing times contrasts with a mild linear increase on the ZTA samples. The natural metabolic activity induces NO formation in bacteria.50 This is likely the source of NO observed for bacteria on the ZTA substrates. However, it appears that an additional (and perhaps preponderant) source of NO was present within the bacteria exposed to the Si3N4 surfaces. It is hypothesized that an external flow of nitrogen from the Si3N4 substrates into the intracellular space activated NO synthases within the living bacteria and thereby altered their intrinsic metabolism.
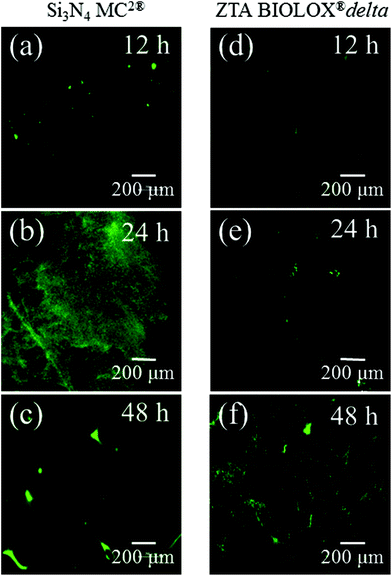 |
| Fig. 3 Time-lapse fluorescence spectroscopy on DAF-2(NO)-stained S. epidermidis on both Si3N4 ((a), (b), and (c)) and ZTA bioceramics ((d), (e), and (f)). | |
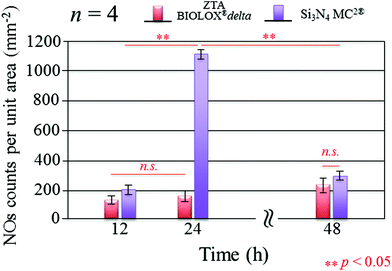 |
| Fig. 4 Statistically validated results for experiments of NO-green counting pixels on fluorescence micrographs during time-lapse bacterial acquisition (n = 4 for each Si3N4 and ZTA substrates). | |
3.2
In situ Raman monitoring of metabolism in living bacteria
In situ Raman monitoring of S. epidermidis exposed to the Si3N4 and ZTA substrates was performed with the twofold purposes of interpreting the results of biological assays described in the previous sub-section and providing further insight into bacterial metabolism. Fig. 5(a) and (b) respectively show in situ collected and deconvoluted Raman spectra of living S. epidermidis after 48 h of exposure to the Si3N4 substrates. The spectra (between 600 and 900 cm−1) displayed a number of bands belonging to the bacteria's organic molecules. They are labeled in the inset and their physical meaning is provided in Table 1 together with their precise spectral locations and literature references.51–55 The intensity of Band 7 located at ∼860 cm−1 served as a reference to normalize the spectra. It was attributed to the C–O–C 1,4 glycosidic link in carbohydrates51 and showed a constant intensity independent of exposure because of the contributions from the testing medium. At 48 h of exposure to the Si3N4 substrates, Bands 1, 2, and 5 (at 655, 670, and 782 cm−1, respectively) completely disappeared. These three bands belong to the bacteria's DNA. They specifically represent the vibrations in deoxyadenosine triphosphate (dATP),52 ring breathing of guanine (Gua),53 and phosphodiester stretching,54 respectively. Moreover, Band 3 at ∼730 cm−1, which belongs to adenine DNA, showed a significant decrease and broadening in intensity. These variations in the DNA bands were not observed for bacteria exposed to the ZTA substrate. In Fig. 5(c), the relative Raman intensity I5/I7 is plotted as a function of exposure time for bacteria exposed to both Si3N4 and ZTA substrates. This ratio is a measure of DNA degradation. Lower intensities correlated with increased DNA damage. The plot in Fig. 5(c) shows that DNA degradation within the bacteria exposed to Si3N4 substrates was barely observed at the initial exposure, but it abruptly appeared after ∼24 h. Conversely, no tangible DNA degradation was detected within the bacteria exposed to the ZTA substrates.
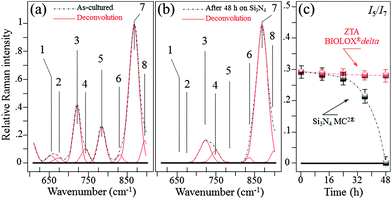 |
| Fig. 5
In situ collected and deconvoluted (average) Raman spectra of living S. epidermidis as cultured (a) and after 48 h of exposure (b) to Si3N4 substrates in the frequency interval of 600–900 cm−1. In (c), relative Raman intensity I5/I7 as a function of exposure time, which was assumed as a measure of DNA degradation and acquired for bacteria exposed to both Si3N4 and ZTA substrates. | |
Table 1 Spectral location, labeling of vibrational origin, and related references of the Raman bands of S. epidermidis in the frequency interval of 600–900 cm−1
Band |
Assignment |
Frequency (cm−1) |
Ref. |
1 |
Ring vibrations in poly-deoxyadenosine |
655 |
51
|
2 |
Ring breathing in guanine |
670 |
51
|
3 |
Adenine DNA & phosphatidylserine |
730 |
52 and 53
|
4 |
Ring breathing DNA |
746 |
52
|
5 |
Phosphodiester stretching |
782 |
54
|
6 |
Out-of-plane ring breathing in DNA |
830 |
52 and 55
|
7 |
COC 1,4 glycosidic link in carbohydrates |
860 |
54
|
8 |
C–C stretching in the protein backbone |
896 |
55
|
In previously published literature,56–58 the observed vibrational response of bacterial DNA was specifically explained in terms of alterations of the DNA polymerase and backbone structure. Note that Band 3, located at 730 cm−1, decreased with exposure time. This band is associated with phosphatidylserine53 which is a phospholipid belonging to the bacterial membrane. It plays a key role in bacterial cell signaling death. As discussed below, the chemical changes in membrane lipids were complex. Their time-lapse evolution is better described using explicit phospholipid Raman fingerprints at higher frequencies.
Fig. 6 shows the average Raman spectra collected from S. epidermidis in the frequency interval between 900 and 1750 cm−1(ref. 59–62) for (a) as-cultured, (b) 24 h exposure and (c) 48 h exposure to the Si3N4 substrates. In this zone there were 13 deconvoluted sub-bands. Their physical origins are listed in Table 2 together with their related literature references. The time-lapse changes observed in these bands were associated with metabolic reactions between the bacteria and the substrate. The reference band for intensity emission in this spectral zone was Band 9 at 937 cm−1. It contained contributions from the C–C α-helix protein stretching, C–C–N+ symmetric stretching in lipids, and C–O–C glycosidic carbohydrate linkage. This band remained constant during the time-lapse experiments because of the large contribution by the medium.63 The Raman zone between 1200 and 1500 cm−1 contained five bands (labeled 14–18 in Fig. 6). According to Table 2, the two stronger Bands 16 (at 1313 cm−1) and 18 (at 1440 cm−1) were due to the twisting and bending of the saturated CH2 bonds in membrane lipids, respectively. By comparing (a), (b) and (c) of Fig. 6, it was noted that these two bands underwent significant enhancement in their relative intensity with respect to the reference Band 9 (as well as the as-cultured conditions (a)) after 24 h of exposure to the Si3N4 surface (b); this was followed by a reduction in intensity after 48 h (c). The intensity changes for Bands 16 and 18 signify the acidification of the membrane lipids.60 The lipid doublet of Bands 12 and 13 occurring at 1092 (C–C gauche stretching) and 1125 cm−1 (C–C trans stretching), respectively, also indicated alterations of the lipid membrane structure at intermediate times. Fatty acid tails, packed in an orderly trans conformation in the solid phase, were numerous at 24 h (Band 13 in the doublet), whereas gauche conformers formed a disordered fluid phase at 48 h (Band 12 in the doublet).61 There is a wealth of literature published on the ability of bacteria to control the biophysical properties of membrane phospholipids in the presence of an external physiological stress.64–71 It is conceivable that the physiological stress experienced by S. epidermidis after 24 h was due to the release of ammonia from the Si3N4 substrates; its presence as various NO moieties was likely metabolized by the bacteria. If this hypothesis is true, the observed structural alterations of the membrane lipids may be attributed to the attempts made by the bacteria to maintain the membrane functionality. Such a physiological reaction along with the presence of a thick peptidoglycan layer on the external side of the lipid membrane might delay bacterial disruption, but these are mere intermediate steps in the cascade of events leading to chemical lysis.
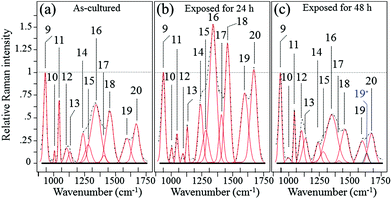 |
| Fig. 6 Average Raman spectra collected on as cultured (a), 24 h-exposed (b), and 48 h-exposed bacteria (c) on Si3N4 substrates in the frequency interval of 900–1750 cm−1. | |
Table 2 Spectral location, labeling of vibrational origin, and related references of the Raman bands of S. epidermidis in the frequency interval 900–1750 cm−1
Band |
Assignment |
Frequency (cm−1) |
Ref. |
9 |
C–O–C glycosidic linkage of carbohydrates |
937 |
51
|
10 |
Ring breathing in phenylalanine |
1004 |
60
|
11 |
C–H in-plane bending in phenylalanine |
1043 |
55
|
12 |
C–C gauche stretching in membrane lipids |
1092 |
51 and 60
|
13 |
C–C trans stretching in membrane lipids |
1125 |
60 and 61
|
14 |
C–N stretching Amide III in β-sheets |
1255 |
62
|
15 |
C–N stretching Amide III in β-turns |
1284 |
59 and 62
|
16 |
Twisting of saturated CH2 in membrane lipids |
1313 |
51
|
17 |
Symmetric C O stretching of COO− |
1400 |
55
|
18 |
Bending of saturated CH2 in membrane lipids |
1440 |
54 and 60
|
19 |
C O stretching Amide I (α-helix) |
1600 |
55 and 60
|
19* |
C O stretching Amide I (degraded structure) |
1630 |
55
|
20 |
C O stretching Amide I (β-sheets) |
1680 |
51
|
The trends of protein-related bands, mainly Bands 10 (1004 cm−1) and 11 (1043 cm−1) (synthetic ring breathing in phenylalanine and C–C vibrations in carbohydrates, respectively), Band 19 (1600 cm−1) (a mix of C
C vibrations in the aromatic ring and C
O stretching, Amide I in α-helixes), and Band 20 (1680 cm−1) (C
O stretching, Amide I in β-sheets) could also be considered as the result of membrane lipid peroxidation.72 In response to perturbations (e.g. NO radicals), membrane lipids modify their polar head groups,73 which in turn affects the lipid–protein interactions.74 Stress conditions and the subsequent structural response of lipids increase the amount of specific proteins and/or de novo protein synthesis.75–81 The appearance of a band at ∼1630 cm−1 after 48 h of exposure to the Si3N4 substrates is an interesting new feature. Labeled as Band 19* and emphasized in blue color in Fig. 6(c), this band was interpreted as an upshift of the C
O stretching vibrations in Amide I;82 it suggests a structural change from an α-helix into a degraded configuration.
Among the protein-related bands, a specific fingerprint for the external layer of the membrane in Gram-positive bacteria is the symmetric C
O stretching of COO−, located at ∼1400 cm−1 (Band 17 in Fig. 6).83 This band represents peptide sub-units in peptidoglycan structures. The intensity trend of Band 17 is similar to that of bands from lipids and other bands related to proteins, which were observed in the same spectral zone. Its increase at intermediate exposure times (i.e., 24 h; cf. Fig. 6(a) and (b)) suggested an initial thickening of the peptidoglycan layer as a protective metabolic effort by bacteria, whereas, its almost complete disappearance after 48 h (Fig. 6(c)) was interpreted as a dismantling of the peptidoglycan structure under the antibacterial influence of the Si3N4.
4. Discussion
4.1 Antibacterial performance of oxide and non-oxide ceramics
The recently published review by Kurtz et al. of total hip arthroplasty patients from the Medicare population associated a reduced risk of PJI with the use of Al2O3-based ceramics.44 However, their review was insufficient to assign causality. The authors suggested that further research was necessary to clearly associate the use of ceramic bearings with peri- and post-operative complication avoidance. The current study was therefore designed to compare the bacteriostatic behavior of oxide and non-oxide bioceramics for use in hip arthroplasty. Concurrent evaluations of bacteria proliferation, NO intracellular activity, and in situ changes in the bacteria's metabolism demonstrated that Si3N4 bioceramics possess intrinsic antibacterial characteristics. While ZTA fared better than PEEK, there was no tangible evidence of any inherent antibacterial mechanism. In fact, although the bacteria growth was slower for ZTA, it followed a similar linear tendency to PEEK. The trend of increasing and then decreasing bacterial proliferation on non-oxide Si3N4 suggests that minute elution of nitrogen from its surface played a key role in the lysis of S. epidermidis. Only Si3N4 possessed this surface-chemistry mechanism among the tested materials. Moreover, the only slightly lower amount of bacteria proliferation for ZTA compared to PEEK was another significant output of this study. In the absence of a bactericidal effect of chemical nature in both ZTA and PEEK samples, as confirmed by the present data, and excluding topographic differences among the tested samples (in both cases finely polished and with similar roughness values), such a slight difference could simply be related to a different kinetics in the biofilm formation. In support of this hypothesis, it could be noted that the difference in the CFU concentration was statistically insignificant up to 24 h (cf.Fig. 2), which suggests the difference in bacterial proliferation being progressively established rather than merely concentrated at the initial stage of bacterial adhesion. In a recently published bacteriostatic study comparing ZTA, Al2O3, CoCr, and highly cross-linked polyethylene, Sorrentino et al. proposed that favorable protein adsorption (e.g., albumin) onto the Al2O3-based ceramics competitively prevented significant adhesion of two bacteria (S. epidermidis and S. aureus).84 Nevertheless, their overall results are consistent with the findings of this study; they also demonstrated an increasing number of viable S. epidermidis colonies on ZTA between 24 and 48 h.
Finally, note that the current study partly contradicts the biological results previously reported by Piconi et al. which showed comparable antibacterial behavior for ZTA and Si3N4.85 The ZTA they examined was the same material used in the current study. However, they unfortunately did not disclose details on the type of Si3N4 used in their investigation including its sintering additives, impurity levels, overall composition, and stoichiometry. These compositional details may be crucial in determining the interactions between Si3N4 and bacteria. For example, it is known that certain impurity ions (e.g., Ca2+, Mg2+, and Fe3+), which are common in raw Si3N4 powders may protect prokaryotic membranes and thereby prolong the life of adherent bacteria.86 In addition, sintering conditions can markedly affect the formation of grain-boundary phases and the stoichiometry of the Si3N4 polycrystals (e.g., from Si- to N-rich surface lattice configurations).87 An altered stoichiometry could in turn affect the efficacy of the antibacterial effect in Si3N4 bioceramics, because of the chemical nature of such an effect. In the next section, we describe the main chemical reactions, which ultimately lead to bacterial lysis. According to such chemical reactions, the need for a balance between the dissociation of silanol and amine sites at the Si3N4 surface in aqueous solution is hereafter discussed as a key factor in the development of an efficient antibacterial kinetics.
4.2 The chemical origin of the antibacterial behavior of Si3N4
For Si3N4 in an aqueous solution, the homolytic cleavage of the covalent Si–N bonds results in the formation of silicon oxide moieties (Si–O) and ammonia (NH3). The Si–O moieties ultimately react with water to form surface silanols in accordance with the following equations: | Si3N4 + 6H2O → 3SiO2 + 4NH3 | (1) |
Previous phenomenological studies have reported that silanols (which form reactive radicals) and ammonia (which increases paracellular permeability) counteract several types of bacteria.88,89 However, transient drifts in the Si3N4 stoichiometry are also believed to have played a fundamental role in the cascade of events leading to the observed antibacterial action. Emission of free electrons, splitting of water, and the dissociation of silanol and amine sites occur during these transient reactions in accordance with the following equations:90–92
| Si–OH2+(s) → Si–OH(s) + H+(aq) | (5) |
| Si–OH(s) → Si–O−(s) + H+(aq) | (6) |
| Si–NH3+(s) → Si–NH2(s) + H+(aq) | (7) |
where the subscripts (s) and (aq) refer to the solid and aqueous states, respectively. Note that the dissociation of surface silanols (
i.e.,
eqn (5)–(7)) leads to the formation of superoxide radicals, such as (Ξ Si–O˙) and (Ξ Si–O˙
2). Unpaired electrons not only split water (
eqn (4)) but also react with adsorbed O
2 on the Si
3N
4 surface to yield O
2˙
− anions and other highly oxidative protonated radicals. Ammonia initially oxidizes into hydroxylamine (NH
2OH) according to a process of ammonia monooxygenase.
93 The surface superoxide radicals (
cf.eqn (4)–(6)) and two electrons from ammonia contribute one O atom in order to form NH
2OH. An additional reductive contribution leads to oxidation of NH
2OH into nitrite (NO
2–) and forms H
2O through a process of hydroxylamine oxidoreductase. This process releases four electrons, two of which sustain further ammonia oxidation, while the remaining two electrons are metabolized by the bacteria generating proton gradients.
93 Besides nitrite, which is the principal product of ammonia oxidation, additional highly reactive gaseous by-products are also developed by ammonia oxidation. These include nitrogen dioxide, NO
2, and nitric oxide, NO, leading to the formation of peroxynitrite (
−OONO).
94 An overview of this process is given in
Fig. 7. The cascade of Si
3N
4 surface chemical reactions, which include monooxygenase, oxidoreductase, and the formation of gaseous by-products from the ammonia, can be given as follows.
95,96 | NH3 + 2e− + 2H + O2 → NH2OH + H2O | (8) |
| NH2OH + H2O → HNO2 + 4e− + 4H+ | (9) |
| 2HNO2 → NO + NO2 + H2O | (10) |
| 2NH3 + 3O2 → 2NO2− + 2H2O + 2H+ | (11) |
| 4NO2− + 4H+ + O2 → 4NO2 (or 2N2O4) + 2H2O | (12) |
| 3NH3 + 3N2O4 → NO2− + 6NO + N2 + 4H2O + H+ | (13) |
| NH3 + NO2− → N2 + H2O + OH− | (14) |
| O2˙− + ˙NO → −OO–N O | (15) |
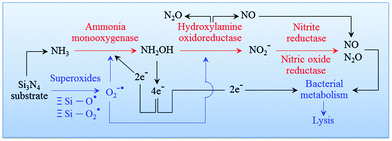 |
| Fig. 7 Pathway of an oxidation cascade of events, which includes: (i) ammonia monooxygenase; (ii) hydroxylamine oxidoreductase; (iii) nitrite reductase; and, (iv) nitric oxide reductase. | |
The equilibrium points of eqn (10) as well as eqn (12) and (13) are dependent upon pH. Their equilibrium influences the amount of NO at the Si3N4/bacteria interface. It is important to note that as the bacteria tend to proliferate the local pH is shifted to acidic values. In turn, this results in more NO production and in a consequent reduction of bacterial population, while counterbalancing the pH change and reestablishing an alkaline environment. In other words, the pH buffering effect should be considered as the phenomenological manifestation of a chemical mechanism behind the Si3N4's bacteriostatic behavior, which starts from the elution of ammonia from the solid Si3N4 surface (eqn (1)). In a companion paper,97 the above-mentioned pH buffering effect at the surface of Si3N4 in an aqueous environment will be visualized and quantified by means of systematic experiments using a specially tailored pH microscope. For the sake of the present discussion, we anticipate here that in the immediate neighborhood of the Si3N4 surface, the initial rate of pH restoration from an acidic environment at pH = 4.5 is on the order of 0.3 min−1 in the first 5 min exposure. Then, the surface reaction achieves an intermediate stage of substantially lowered pH-buffering (average) rate ∼0.04 min−1 toward physiological pH values in the successive 10 min exposure. By borrowing a commonly used tag, we could define the pH buffering reaction as an “intelligent” effect, which regulates itself according to the external need for locally restoring the physiological pH value.
The diatomic free radical NO is known to play a fundamental role in the human immunology as a potent antimicrobial agent;98 and it was detected in copious amounts on the cytoplasmic side of S. epidermidis after 24 h of exposure to Si3N4. In combination with superoxides, NO is the chemical species that activates macrophages to counteract bacterial infection and the increased presence of cytokines. It induces oxidative stress in bacteria by creating a multitude of reactive intermediates, including nitrous oxide (N2O) and peroxynitrite (−OONO). Peroxynitrite is lethal to bacteria because it damages their membranes through lipid peroxidation.99 Moreover, the bacterial membrane is highly permeable to NO. This allows its prompt intracellular diffusion where it attacks lipids, targets DNA strands with nitration/nitrosylation of their amino acid residues,100 and causes irreparable damage to protein structures.101,102 More specifically, NO nitration of tyrosine and cysteine protein residues occurs because of additional reaction products (e.g., N2O3– and N2O4–) causing irremediable alterations to overall protein functions.103 The effects of NO on lipids, DNA, and proteins were observed in the current study using in situ Raman spectroscopy on living bacteria (cf., section 3.2). Raulli et al. provided direct demonstration of the bactericidal effect of nitric oxide against a range of both Gram-positive and Gram-negative species including those relevant to implant infections.104 Given this supportive literature, it is suggested that the concurrent development of superoxide and ammonia moieties on Si3N4 surfaces leads to NO and −OONO formation. In turn, these compounds impart an effective chemical mechanism in resisting bacterial adhesion.
4.3 Time-dependent alteration of bacterial metabolism
The in situ Raman spectroscopy data in section 3.2 represent a unique source of information for rationalizing the chemical nature of the Si3N4 bacteriostatic behavior. There were three main physiological changes which occurred in S. epidermidis upon exposure to Si3N4 substrates: (i) annihilation of the major DNA-related bands; (ii) alterations of the phospholipid-related bands reflecting the major modifications of the membrane structure, and (iii) alterations of the protein-related bands revealing irreversible damage to their structure. Fig. 8 provides a schematic diagram of the interaction between Si3N4 and S. epidermidis (a), and the non-stoichiometric reactions occurring at the Si3N4 surface (b). The data collected in the frequency interval 600–900 cm−1 showed that prolonged exposure to Si3N4 damaged the DNA structure of S. epidermidis as follows: (i) the disappearance of Band 1 at 655 cm−1, which is a vibrational signature of dATP – a main nucleoside triphosphate, catalytically active as a substrate in DNA polymerase and an energy-transferring molecule for bacterial cell viability;56 (ii) the disappearance of Band 2 at 670 cm−1, the Raman fingerprint of Gua – one of the main nucleobases and basic building blocks of DNA, and (iii) annihilation of Band 5 at 782 cm−1, the main Raman emission from phosphodiester in the backbone of the DNA strands. Additionally, the appearance of a new sub-band, labeled as Band 19*, emitted from a random coil after 48 h of exposure to Si3N4 suggested that proteins changed from their α-helix conformation into a disordered random coil due to the fragmentation induced by reactions with free radicals.
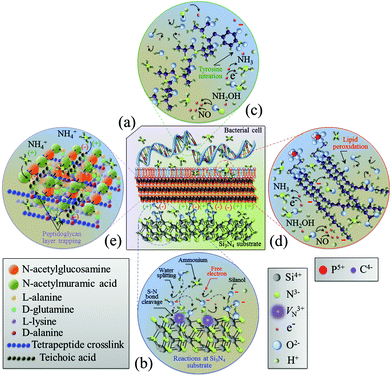 |
| Fig. 8 Schematic diagrams of (a) the surface interaction between Si3N4 and S. epidermidis, (b) the off-stoichiometric reactions taking place at the Si3N4 surface in the biological environment, (c) peroxidation of membrane lipids, and (d) charge-driven ammonium trapping in the peptidoglycan layer. | |
It is known that the reaction of NO with the tyrosyl radical in the catalytic turnover of ribonucleotide reductase is responsible for the suppression of DNA synthesis in bacteria.57,58 It has also been reported that the principal targets of peroxynitrite are sulfhydryl and tyrosyl side chains with nitration of tyrosine to 3-nitrotyrosine.105–107 Protein tyrosine nitration interferes with a range of biochemical processes108 and prevents post-translational modifications (e.g., phosphorylation).109 Once started, the process of DNA fragmentation accelerates. Free radical damage to secondary structures opens the DNA chains and increases their susceptibility to additional free radicals. This eventually results in the deterioration of the α-helix structure into random coils. Fig. 8(c) schematically shows free-radical-induced DNA structural modifications through tyrosine nitration.
The interaction between dissociating surface silanols and quaternary ammonium groups has long been considered the essential key in assessing the degradation of membrane phospholipids.89,110 The ability of bacteria to control the biophysical properties of their membrane phospholipids is a well-known phenomenon. By modifying various types of fatty acids, they are able to adapt to a number of environmental factors such as temperature, pressure, ions, nutrients, and toxic substances.64 In the current study, Raman follow-up experiments elucidated the evolution of thee fatty acids which provided an efficient biomarker for changes in the physiological status of S. epidermidis by external factors.65 Isomerization of cis unsaturated fatty acids to trans and the presence of trans fatty acids have also been associated with bacteria survival at low nutrient levels.66–68 The Raman results from the current study are consistent with this prior literature. The data strongly suggest that S. epidermidis underwent significant physiological stress after being exposed to Si3N4; the bacteria responded by isomerizing their membranes to create a trans fatty acid barrier. Moreover, it is hypothesized that the NO radicals released by the substrates, which are lipophilic and freely diffusible through the membrane, damaged the DNA and initiated lipid peroxidation.69–71 As previously discussed, deprotonated silanols likely accounted for the peroxidation of lipids. Abstraction of hydrogen from a methylene group by a ˙OH radical is the most efficient process in fatty acids,111 whereas a surface dissociated silanol, Ξ Si–O−, effectively links to a membrane tetraalkylammonium group, +N(CH3)3–CH2–R, in phospholipids:98
| –CH2– + ˙OH → –˙CH– + H2O | (16) |
| Ξ Si–O− + +N(CH3)3–CH2–R → Ξ Si–O˙−⋯+N(CH3)3–CH2–R | (17) |
where R represents a repetitive sequence of methylene groups. However, unlike Gram-negative bacteria, a thick layer of peptidoglycans protects the external membrane in Gram-positive bacilli. Consequently, a direct interaction between membrane phospholipids and deprotonated silanols from the Si
3N
4 substrate did not immediately occur; it only appeared after a characteristic time lag of ∼24 h.
The Raman probe results for peptide sub-units in peptidoglycan structures (i.e., Band 17 at ∼1400 cm−1) revealed an initial thickening of the peptidoglycan layer after 24 h exposure, but its full disassembly was only observed after 48 h of exposure (cf.Fig. 6). This was the general trend for all proteins. Hochgräfe et al. reported that Staphylococcus aureus showed an increased rate of protein synthesis under anaerobic conditions after exposure to NO stress.79 Indeed NO disrupts the respiratory chain of bacteria by binding to cytochromes.80,81 An accelerated synthesis of proteins promoting anaerobic metabolism is a crucial activity in tolerating NO stress. It is assumed that the intensity increase in protein-related Raman bands at the intermediate time-points (<24 h, cf., Fig. 2) occurred because of this same mechanism. However, the Raman experiments showed that exposure above a given time-threshold (i.e., ∼24 h) was necessary for the antibacterial effect to be observed. This time-lag was interpreted as the sum of the bacteria's initial metabolic reaction plus the time necessary for the dismantling of the external peptide wall. In this context, ion-exchange interactions between deprotonated silanol groups and peptides are strong;112 dismantling of the peptidoglycan layer to ultimately collapse the membrane wall may occur by a cumulative process of ionic abstraction. A more likely scenario is the strong electrostatic attraction between the negatively charged teichoic acid chains in the peptidoglycan layer and the positively charged NH4+ species produced by the Si3N4 substrates. Ammonium moieties become trapped in the peptidoglycan layer and start to produce reactive oxidizing N-intermediates. A similar mechanism has been proposed for charged nanoparticles with positively charged holes.113,114 Conversely, NO may have contributed to peptidoglycan dismantling through a mechanism of oxidative and nitrosative damage of the internal lipid membrane.115Fig. 8(d) and (e) provide schematic diagrams of the hypothesized peroxidation of lipids leading to the degradation and the structure of the peptidoglycan layer trapping NH4+ moieties, respectively. Additional characterization of the same bioceramic samples by Fourier transform infrared spectroscopy will be provided in a forthcoming companion paper. It attempts to substantiate the Raman results by interpreting the chemical fingerprints present in the infrared zone of the S. epidermidis spectrum.
4.4 Limitations of the current study
This study had a number of limitations. The foremost among them was that the comparative testing used only one bacteria species, Gram-positive S. epidermidis. Previous Si3N4 studies examined a number of nosocomial strains, both Gram-positive and Gram-negative, using several comparative biomaterials (e.g., PEEK, titanium, stainless steel).40–43 However, none of the prior work compared Al2O3-based oxide ceramics with non-oxide Si3N4. Consequently, additional experimental efforts are needed to determine whether the results from this work will hold for alternative bacteria genus and species. The current study provided clear hints about the chemical nature of the antibacterial mechanism activated on the surface of Si3N4 ceramic. Note, however, that the present protocol was designed to keep the bacteria on the material surface and to evaluate their physiological reaction with the substrate. Therefore, the present evaluation was not comprehensive of the initial stage of bacterial adhesion, which usually renders the antimicrobial behavior of any abiotic material multivariate.116,117 Surface charge and wettability are likewise contributing factors for the initial adhesion of prokaryotic or eukaryotic cells and plasma proteins.41,46,84 All of these issues have yet to be fully elucidated when comparing the anti-infective properties of oxide and non-oxide ceramics. Again, this will be part of ongoing and future research. Lastly, the efficacy of Si3N4 in reducing the incidence of clinical infections is yet unknown. While the in vitro data presented in this study and other similar studies suggest that improved clinical outcomes might be expected, these results are currently limited to the studied experimental conditions.
5. Conclusion
This study combined biological testing using fluorescence microscopy (DAPI, Sytox® Green, and DAF-2(NO)) and in situ time-lapse label-free Raman spectroscopy to compare the smooth surfaces of oxide and non-oxide bioceramics with respect to their antibacterial behavior against Gram-positive S. epidermidis. The experimental results consistently showed that only non-oxide Si3N4 possessed an intrinsic chemistry-related antibacterial mechanism. These results contrast with the previously published studies that either (i) retrospectively associated a lack of infection in Medicare patients with Al2O3-based oxide ceramics;44 (ii) correlated the bacteriostatic ability of oxide bioceramics with increased protein adsorption;84 or (iii) described the equivalent antimicrobial performance for non-oxide and oxide ceramics.85 The bacteriostatic behavior of the Si3N4 examined in the current experiments was a result of its unique surface chemistry. The simple cleavage of the Si–N covalent bond led to the formation of surface silanols and ammonia. The oxidation of ammonia into hydroxylamine along with the concurrent presence of superoxides and highly volatile species such as nitric oxide and peroxynitrite damaged bacterial DNA and membrane components and altered the protein structures. Both fluorescence and Raman time-lapse experiments consistently showed a time lag of ∼24 h between the exposure of the bacteria to the Si3N4 substrates and their eventual death. This delay was the result of a metabolic reaction by S. epidermidis in an attempt to adjust the biophysical properties of proteins and membrane phospholipids in response to a cascade of adverse chemical reactions ultimately leading to bacterial lysis.
Conflicts of interest
Giuseppe Pezzotti is a consultant to Amedica Corporation, and Bryan McEntire, Ryan M. Bock and B. Sonny Bal work at Amedica Corporation.
Acknowledgements
The authors gratefully thank Mr S. Horiguchi for his help with biological tests on bacteria.
References
- K. J. Bozic, E. Lau, S. Kurtz, K. Ong, H. Rubash, T. P. Vail and D. J. Berry, Patient-Related Risk Factors for Periprosthetic Joint Infection and Postoperative Mortality Following Total Hip Arthroplasty in Medicare Patients, J. Bone Jt. Surg., Am. Vol., 2012, 94, 794–800 CrossRef PubMed.
- M. G. Wilson, K. Kelley and T. S. Thornhill, Infection as a Complication of Total Knee-Replacement Arthroplasty. Risk Factors and Treatment in Sixty-Seven Cases, J. Bone Jt. Surg., Am. Vol., 1990, 72A, 878–883 CrossRef.
- W. Zimmerli, A. Trampuz and P. E. Ochsner, Prosthetic-joint infections, N. Engl. J. Med., 2004, 351, 1645–1654 CrossRef PubMed.
- A. G. Gristina, Biomaterial-Centered Infection: Microbial Adhesion Versus Tissue Integration, Science, 1987, 237, 1588–1595 CrossRef PubMed.
- J. W. Costerton, P. S. Stewart and E. P. Greenberg, Bacterial Biofilms: A Common Cause of Persistent Infections, Science, 1999, 284, 1318–1322 CrossRef PubMed.
- N. Høiby, O. Ciofu, H. K. Johansen, Z. Song, C. Moser, P. Ø. Jensen, S. Molin, M. Givskov, T. Tolker-Nielsen and T. Bjarnsholt, The Clinical Impact of Bacterial Biofilms, Int. J. Oral Sci., 2011, 3, 55–65 CrossRef PubMed.
- W. Zimmerli, P. D. Lew and F. A. Waldvogel, Pathogenesis of Foreign Body Infection. Evidence for a Local Granulocyte Defect, J. Clin. Invest., 1984, 73, 1191–1200 CrossRef PubMed.
- R. M. Donlan and J. W. Costerton, Biofilms: Survival Mechanisms of Clinically Relevant Microorganisms, Clin. Microbiol. Rev., 2002, 15, 167–193 CrossRef PubMed.
- D. R. Murdoch, S. A. Roberts, V. G. Fowler Jr., M. A. Shah, S. L. Taylor, A. J. Morris and G. R. Corey, Infection of Orthopedic Prostheses after Staphylococcus aureus Bacteremia, Clin. Infect. Dis., 2001, 32, 647–649 CrossRef PubMed.
- L. Pulido, E. Ghanem, A. Joshi, J. J. Purtill and J. Parvizi, Periprosthetic Joint Infection: The Incidence, Timing, and Predisposing Factors, Clin. Orthop. Relat. Res., 2008, 466, 1710–1715 CrossRef PubMed.
- L. T. Buller, F. Y. Sabry, R. W. Easton, A. K. Klika and W. K. Barsoum, The Preoperative Prediction of Success Following Irrigation and Debridement With Polyethylene Exchange for Hip and Knee Prosthetic Joint Infections, J. Arthroplasty, 2012, 27, 857–864 CrossRef PubMed.
- C. L. Romanò, D. Romanò, N. Logoluso and E. Meani, Septic Versus Aseptic Hip Revision: How Different?, J Orthop. Traumatol., 2010, 11, 167–174 CrossRef PubMed.
- T. J. Foster, Antibiotic resistance in Staphylococcus aureus. Current status and future prospects, FEMS Microbiol. Rev., 2017, 41, 430–449 CrossRef PubMed.
- G. N. Forrest and K. Tamura, Rifampin Combination Therapy for Nonmycobacterial Infections, Clin. Microbiol. Rev., 2010, 23, 14–34 CrossRef PubMed.
- A. Trampuz and W. Zimmerli, Diagnosis and Treatment of Implant-Associated Septic Arthritis and Osteomyelitis, Curr. Infect. Dis. Rep., 2008, 10, 394–403 CrossRef PubMed.
- N. Rao and D. M. Regalla, Uncertain Efficacy of Daptomycin for Prosthetic Joint Infections: A Prospective Case Series, Clin. Orthop. Relat. Res., 2006, 451, 34–37 CrossRef PubMed.
- A. K. John, D. Baldoni, M. Haschke, K. Rentsch, P. Schaerli, W. Zimmerli and A. Trampuz, Efficacy of Daptomycin in Implant-Associated Infection due to Methicillin-Resistant Staphylococcus aureus: Importance of Combination with Rifampin, Antimicrob. Agents Chemother., 2009, 53, 2719–2724 CrossRef PubMed.
- C. A. Aboltins, M. A. Page, K. L. Buising, A. W. J. Jenney, J. R. Daffy, P. F. M. Choong and P. A. Stanley, Treatment of Staphylococcal Prosthetic Joint Infections with Debridement, Prosthesis Retention and Oral Rifampicin and Fusidic Acid, Clin. Microbiol. Infect., 2007, 13, 586–591 CrossRef PubMed.
- J. Segreti, J. A. Nelson and G. M. Trenholme, Prolonged Suppressive Antibiotic Therapy for Infected Orthopedic Prostheses, Clin. Infect. Dis, 1998, 27, 711–713 CrossRef PubMed.
- W. Kim, W. Zhu, G. L. Hendicks, D. Van Tyne, A. D. Steele, C. E. Keohane, N. Fricke, A. L. Conery, S. Shen, W. Pan, K. Lee, R. Rajamuthiah, B. B. Fuchs, P. M. Vlahovska, W. M. Wuest, M. S. Gilmore, H. Gao, F. M. Ausubel and E. Mylonakis, A new class of synthetic retinoid antibiotics effective against bacterial persisters, Nature, 2018, 556, 103–107 CrossRef PubMed.
- N. J. Hickok, I. M. Shapiro and A. F. Chen, The impact of incorporating antimicrobials into implant surfaces, J. Dent. Res., 2018, 97, 14–22 CrossRef PubMed.
- K. Anagnostakos and C. Meyer, Antibiotic elution from hip and knee acrylic bone cement spacers: a systematic review, BioMed. Res. Int., 2017, 2017, 4657874 Search PubMed.
- J. Gallo, A. Panacek, R. Pricek, E. Kriegova, S. Hradilova, M. Hobza and M. Holinka, Silver nanocoating technology in the prevention of prosthetic joint infection, Mater., 2016, 9, 337 CrossRef PubMed.
- T. Kabata, T. Maeda, Y. Kajino, K. Hasegawa, D. Inoue, T. Yamamoto, T. Takagi, T. Ohmori and H. Tsuchiya, Iodine-supported hip implants: short term clinical results, BioMed. Res. Int., 2015, 2015, 368124 Search PubMed.
- C. L. Romanò, S. Scarponi, E. Gallazzi, D. Romanò and L. Drago, Antibacterial coating of implants in orthopaedics and trauma: a classification proposal in an evolving panorama, J. Orthop. Surg. Res., 2015, 10, 157 CrossRef PubMed.
- E. M. Hetrick and M. H. Schoenfisch, Reducing Implant-Related Infections: Active Release Strategies, Chem. Soc. Rev., 2006, 35, 780 RSC.
- K. Vasilev, J. Cook and H. J. Griesser, Antibacterial Surfaces for Biomedical Devices, Expert Rev. Med. Devices, 2009, 6, 553–567 CrossRef PubMed.
- D. Campoccia, L. Montanaro and C. R. Arciola, A Review of the Biomaterials Technologies for Infection-Resistant Surfaces, Biomaterials, 2013, 34, 8533–8554 CrossRef PubMed.
- J. Hasan, R. J. Crawford and E. P. Ivanova, Antibacterial Surfaces: The Quest for a New Generation of Biomaterials, Trends Biotechnol., 2013, 31, 295 CrossRef PubMed.
- C. Desrousseaux, V. Sautou, S. Descamps and O. Traoré, Modification of the Surfaces of Medical Devices to Prevent Microbial Adhesion and Biofilm Formation, J. Hosp. Infect., 2013, 85, 87–93 CrossRef PubMed.
- A. Francesko, M. M. Fernandes, K. Ivanova, S. Amorim, R. L. Reis, I. Pashkuleva, E. Mendoza, A. Pfeifer, T. Heinze and T. Tzanov, Bacteria-responsive multilayer coatings comprising polycationic nanospheres for bacteria biofilm prevention on urinary catheters, Acta Biomater., 2016, 33, 203–212 CrossRef PubMed.
- C. G. Zalavras, M. J. Patzakis and P. Holtom, Local Antibiotic Therapy in the Treatment of Open Fractures and Osteomyelitis, Clin. Orthop. Relat. Res., 2004, 427, 86–93 CrossRef.
- P. Hinarejos, Use of Antibiotic-Loaded Cement in Total Knee Arthroplasty, World J. Orthop., 2015, 6, 877 CrossRef PubMed.
- M. Stravinskas, M. Nilsson, P. Horstmann, M. M. Petersen, S. Tarasevicius and L. Lidgren, Antibiotic containing bone substitute in major hip surgery: a long term gentamicin elution study, J. Bone Jt. Infect., 2018, 3, 68–72 CrossRef PubMed.
- H. Van de Belt, D. Neut, J. R. van Horn, H. C. van der Mei, W. Schenk and H. J. Busscher, Or not to treat?, Nat. Med., 1999, 5, 358–359 CrossRef PubMed.
- S. N. Pleskova, I. S. Golubeva and Y. K. Verevkin, Bactericidal Activity of Titanium Dioxide Ultraviolet-Induced Films, Mater. Sci. Eng., C, 2016, 59, 807–817 CrossRef PubMed.
-
B. Domènech, M. Muñoz, D. N. Muraviev and J. Macanás, Polymer-Silver Nanocomposites as Antibacterial Materials, in Microb. Pathog. Strateg. Combat. Them Sci. Technol. Educ., ed. A. Mendez-Vilas, Formatex Research Center, Badajoz, Spain, 2013, pp. 630–640 Search PubMed.
- L. Liu, R. Bhatia and T. J. Webster, Atomic layer deposition of nano-TiO2 thin films with enhanced biocompatibility and microbial activity for orthopedic implants, Int. J. Nanomed., 2017, 12, 8711–8723 CrossRef PubMed.
- S. K. Verma, E. Jha, P. K. Panda, A. Thirumurugan, S. K. S. Parashar, S. Patro and M. Suar, Mechanistic insight into size-dependent enhanced cytotoxicity of industrial antibacterial titanium oxide nanoparticles on colon cells because of reactive oxygen species quenching and neutral lipid alteration, ACS Omega, 2018, 3, 1244–1262 CrossRef.
- G. Pezzotti, R. M. Bock, B. J. McEntire, E. Jones, M. Boffelli, W. Zhu, G. Baggio, F. Boschetto, L. Puppulin, T. Adachi, T. Yamamoto, N. Kanamura, Y. Marunaka and B. S. Bal, Silicon Nitride Bioceramics Induce Chemically Driven Lysis in Porphyromonas Gingivalis, Langmuir, 2016, 32, 3024–3035 CrossRef PubMed.
- R. M. Bock, E. N. Jones, D. A. Ray, B. S. Bal, G. Pezzotti and B. J. McEntire, Bacteriostatic Behavior of Surface-Modulated Silicon Nitride in Comparison to Polyethereetherketone and Titanium, J. Biomed. Mater. Res., Part A, 2017, 105, 1521–1534 CrossRef PubMed.
- D. J. Gorth, S. Puckett, B. Ercan, T. J. Webster, M. Rahaman and B. S. Bal, Decreased Bacteria Activity on Si3N4 Surfaces Compared with PEEK or Titanium, Int. J. Nanomed., 2012, 7, 4829–4840 Search PubMed.
- T. J. Webster, A. A. Patel, M. N. Rahaman and B. S. Bal, Anti-Infective and Osteointegration Properties of Silicon Nitride, Poly (Ether Ether Ketone), and Titanium Implants, Acta Biomater., 2012, 8, 4447–4454 CrossRef PubMed.
- S. M. Kurtz, E. Lau, D. Baykal and B. D. Springer, Outcomes of Ceramic Bearings After Primary Total Hip Arthroplasty in the Medicare Population, J. Arthroplasty, 2016, 32, 743–749 CrossRef PubMed.
- B. J. McEntire, R. Lakshminarayanan, P. Thirugnanasambandam, J. Seitz-Sampson, R. Bock and D. O'Brien, Processing and Characterization of Silicon Nitride Bioceramics, Bioceram. Dev. Appl., 2016, 6, 1000093 Search PubMed.
- R. M. Bock, B. J. McEntire, B. S. Bal, M. N. Rahaman, M. Boffelli and G. Pezzotti, Surface Modulation of Silicon Nitride Ceramics for Orthopaedic Applications, Acta Biomater., 2015, 26, 318–330 CrossRef PubMed.
- W. Burger and H. G. Richter, High Strength and Toughness Alumina Matrix Composites by Transformation Toughening and “in situ” Platelet Reinforcement (ZPTA) - The New Generation of Bioceramics, Key Eng. Mater., 2001, 192–195, 545–548 Search PubMed.
- R. H. Khonsari, P. Berthier, T. Rouillon, J.-P. Perrin and P. Corre, Severe Infectious Complications after PEEK-Derived Implant Placement: Report of Three Cases, J. Oral Maxillofac. Surg. Med. Pathol., 2014, 26, 477–482 CrossRef.
-
T. J. Kessler, J. Barone, C. Kellogg and H. Huang, Holographic transmission gratings for spectral dispersion, in LLE Review Quarterly Report, ed. P. B. Radha, University of Rochester Laboratory for Laser Energetics, Springfield, VA, 2000, vol. 82, pp. 71–77 Search PubMed.
- B. R. Crane, J. Sudhamsu and B. A. Patel, Bacterial Nitric Oxide Synthases, Annu. Rev. Biochem., 2010, 79, 445–470 CrossRef PubMed.
- I. Notingher and L. L. Hench, Raman Microspectroscopy: A Noninvasive Tool for Studies of Individual Living Cells in vitro, Expert Rev. Med. Devices, 2006, 3, 215–234 CrossRef PubMed.
- L. Movileanu, J. M. Benevides and G. J. Thomas, Temperature Dependence of the Raman Spectrum of DNA. I. Raman Signatures of Premelting and Melting Transitions of Poly(dA-dT)·poly(dA-dT), J. Raman Spectrosc., 1999, 30, 637–649 CrossRef.
- G. I. Dovbeshko, V. I. Chegel, N. Y. Gridina, O. P. Repnytska, Y. M. Shirshov, V. P. Tryndiak, I. M. Todor and G. I. Solyanik, Surface Enhanced IR Absorption of Nucleic Acids from Tumor Cells: FTIR Reflectance Study, Biopolymers, 2002, 67, 470–486 CrossRef PubMed.
- A. C. Williams and H. G. M. Edwards, Fourier Transform Raman Spectroscopy of Bacterial Cell Walls, J. Raman Spectrosc., 1994, 25, 673–677 CrossRef.
- A. C. S. Talari, Z. Movasaghi and I. Rehman ur Rehman, Raman Spectroscopy of Biological Tissues, Appl. Spectrosc. Rev., 2015, 50, 46–111 CrossRef.
- M. J. P. Burgers and F. Eckstein, A Study of the Mechanism of DNA Polymerase I from Escherichia coli with Diastereomeric Phosphorothioate Analogs of Deoxyadenosine Triphosphate, J. Biol. Chem., 1979, 254, 6889–6893 Search PubMed.
- M. Lepoivre, F. Fieschi, J. Coves, L. Thelander and M. Fontecave, Inactivation of Ribonucleotide Reductase by Nitric oxide, Biochem. Biophys. Res. Commun., 1991, 179, 442–448 CrossRef PubMed.
- M. Lepoivre, J. M. Flaman and Y. Henry, Early Loss of the Tyrosyl Radical in Ribonucleotide Reductase of Adenocarcinoma Cells Producing Nitric Oxide, J. Biol. Chem., 1992, 267, 22994–23000 Search PubMed.
- J. De Gelder, K. De Gussem, P. Vandenabeele and L. Moens, Reference Database of Raman Spectra of Biological Moleculses, J. Raman Spectrosc., 2007, 38, 1133–1147 CrossRef.
- H. Wu, J. V. Volponi, A. E. Oliver, A. N. Parikh, B. A. Simmons and S. Singh, In vivo Lipidomics using Single-Cell Raman Spectroscopy, Proc. Natl. Acad. Sci. U. S. A., 2011, 108, 3809–3814 CrossRef PubMed.
- J. L. Lippert and W. L. Peticolas, Laser Raman Investigation of the Effect of Cholesterol on Conformational Changes in Dipalmitoyl Lecithin Multilayers, Proc. Natl. Acad. Sci. U. S. A., 1971, 68, 1572–1576 CrossRef.
- S. Cai and B. R. Singh, Identification of β-turn and random coil amide III infrared bands for secondary structure estimation of proteins, Biophys. Chem., 1999, 80, 7–20 CrossRef PubMed.
- R. J. Swain, S. J. Kemp, P. Goldstraw, T. D. Tetley and M. M. Stevens, Spectral Monitoring of Surfactant Clearance during Alveolar Epithelial Type II Cell Differentiation, Biophys. J., 2008, 95, 5978–5987 CrossRef PubMed.
- Y.-M. Zhang and C. O. Rock, Membrane Lipid Homeostasis in Bacteria, Nat. Rev. Microbiol., 2008, 6, 222–233 CrossRef PubMed.
- A. Mrozik, Z. Piotrowska-Seget and S. Łabuzek, Cytoplasmatic Bacterial Membrane Responses to Environmental Perturbations, Pol. J. Environ. Stud., 2004, 13, 487–494 Search PubMed.
- H. J. Heipieper, B. Loffeld, H. Keweloh and J. A. M. De Bont, The cis/trans Isomerisation of Unsaturated Fatty Acids in Preudomonas Putida s12: An Indicator for Environmental Stress due to Organic Compound, Chemosphere, 1995, 30, 1041–1051 CrossRef.
- J. B. Guckert, M. A. Hood and D. C. White, Phospholipid Ester-Linked Fatty Acid Profile Changes during Nutrient Deprivation of Vibrio Cholerae: Increases in the transcis Ratio and Proportions of Cyclopropyl Fatty Acids, Appl. Environ. Microbiol., 1986, 52, 794–801 Search PubMed.
- J. B. Guckert, D. B. Ringelberg and D. C. White, Biosynthesis of Trans Fatty Acids from Acetate in the Bacterium Pseudomonas atlantica, Can. J. Microbiol., 1987, 33, 748–754 CrossRef.
- C. M. Moore, M. M. Nakano, T. Wang, R. W. Ye and J. D. Helmann, Response of Bacillus subtilis to Nitric Oxide and the Nitrosating Agent Sodium Nitroprusside, J. Bacteriol., 2004, 186, 4655–4664 CrossRef PubMed.
- C. Nathan and M. U. Shiloh, Reactive Oxygen and
Nitrogen Intermediates in the Relationship between Mammalian Hosts and Microbial Pathogens, Proc. Natl. Acad. Sci. U. S. A., 2000, 97, 8841–8848 CrossRef.
- R. K. Poole and M. N. Hughes, New Functions for the Ancient Globin Family: Bacterial Responses to Nitric Oxide and Nitrosative Stress, Mol. Microbiol., 2000, 36, 775–783 CrossRef PubMed.
- K. Maquelin, C. Kirschner, L. P. Choo-Smith, N. Van Den Braak, H. P. Endtz, D. Naumann and G. J. Puppels, Identification of Medically Relevant Microorganisms by Vibrational Spectroscopy, J. Microbiol. Methods, 2002, 51, 255–271 CrossRef PubMed.
- Y. Hasegawa, N. Kawada and Y. Nosoh, Change in Chemical Composition of Membrane of Bacillus caldotenax after Shifting the Growth Temperature, Arch. Microbiol., 1980, 126, 103–108 CrossRef PubMed.
- S. B. Rosas, M. Secco and N. E. Ghittoni, Effects of Pesticides on the Fatty Acid and Phospholipid Composition of Escherichia coli, Appl. Environ. Microbiol., 1980, 40, 231–234 Search PubMed.
- R. Margesin and F. Schinner, Properties of Cold-Adapted Microorganisms and their Potential Role in Biotechnology, J. Biotechnol., 1994, 33, 1–14 CrossRef.
- H. A. Thieringer, P. G. Jones and M. Inouye, Cold Shock and Adaptation, BioEssays, 1998, 20, 49–57 CrossRef PubMed.
- N. J. Russell, P. Harrisson, I. A. Johnston, R. Jaenicke, M. Zuber, F. Franks and D. Wynn-Williams, Cold Adaptation of Microorganisms, Philos. Trans. R. Soc., B, 1990, 326, 595–611 CrossRef.
- N. J. Russell, Psychrophilic Bacteria—Molecular Adaptations of Membrane Lipids, Comp. Biochem. Physiol., Part A: Mol. Integr. Physiol., 1997, 118, 489–493 CrossRef PubMed.
- F. Hochgräfe, C. Wolf, S. Fuchs, M. Liebeke, M. Lalk, S. Engelmann and M. Hecker, Nitric Oxide Stress Induces Different Responses but Mediates Comparable Protein Thiol Protection in Bacillus subtilis and Staphylococcus aureus, J. Bacteriol., 2008, 190, 4997–5008 CrossRef PubMed.
- D. A. Wink and J. B. Mitchell, Chemical Biology of Nitric Oxide: Insights into Regulatory, Cytotoxic, and Cytoprotective Mechanisms of Nitric Oxide, Free Radical Biol. Med., 1998, 25, 434–456 CrossRef PubMed.
- B. Beltrán, A. Mathur, M. R. Duchen, J. D. Erusalimsky and S. Moncada, The Effect of Nitric Oxide on Cell Respiration: A Key to Understanding its Role in Cell Survival or Death, Proc. Natl. Acad. Sci. U. S. A., 2000, 97, 14602–14607 CrossRef PubMed.
- J. Anastassopoulou, E. Boukaki, C. Conti, P. Ferraris, E. Giorgini, C. Rubini, S. Sabbatini, T. Theophanides and G. Tosi, Microimaging FT-IR Spectroscopy on Pathological Breast Tissues, Vib. Spectrosc., 2009, 51, 270–275 CrossRef.
- R. Callender and H. Deng, Nonresonance Raman Difference Spectroscopy: A General Probe of Protein Structure, Ligand Binding, Enzymatic Catalysis, and the Structures of Other Biomacromolecules, Annu. Rev. Biophys. Biomol. Struct., 1994, 23, 215–245 CrossRef PubMed.
- R. Sorrentino, A. Cochis, B. Azzimonti, C. Caravaca, J. Chevalier, M. Kuntz, A. A. Porporati, R. M. Streicher and L. Rimondini, Reduced Bacterial Adhesion on Ceramics used for Arthroplasty Applications, J. Eur. Ceram. Soc., 2018, 38(3), 963–970 CrossRef.
- C. Piconi, A. C. Ionescu, A. Cochis, E. Iasi, E. Brambilla and L. Rimondini, Bioceramic Materials Show Reduced Pathological Biofilm Formation, Key Eng. Mater., 2015, 631, 448–453 Search PubMed.
- P. Boyaval, E. Boyaval and M. J. Desmazeaud, Survival of Brevibacterium linens during Nutrient Starvation and Intracellular Changes, Arch. Microbiol., 1985, 141, 128–132 CrossRef PubMed.
- B. J. McEntire, B. S. Bal, M. N. Rahaman, J. Chevalier and G. Pezzotti, Ceramics and Ceramic Coatings in Orthopaedics, J. Eur. Ceram. Soc., 2015, 35, 4327–4343 CrossRef.
- S. Himathongkham and H. Riemann, Destruction of Salmonella typhimurium, Escherichia coli O157:H7 and Listeria monocytogenes in Chicken Manure by Drying and/or Gassing with Ammonia, FEMS Microbiol. Lett., 1999, 171, 179–182 CrossRef PubMed.
- Y. M. Kim, S. Farrah and R. H. Baney, Membrane Damage of Bacteria by Silanols Treatment, Electron. J. Biotechnol., 2007, 10, 252–259 Search PubMed.
-
C. Kajdas, General Approach to Mechanochemistry and Its Relation to Tribochemistry, in Tribol. Eng., INTEC, 2013, pp. 209–240 Search PubMed.
- S. Mezzasalma, Characterization of Silicon Nitride Surface in Water and Acid Environment: A General Approach to the Colloidal Suspensions, J. Colloid Interface Sci., 1996, 180, 413–420 CrossRef.
- J. Sonnefeld, Determination of Surface Charge Density Parameters of Silicon Nitride, Colloids Surf., A, 1996, 108, 27–31 CrossRef.
- D. J. Arp and L. Y. Stein, Metabolism of Inorganic N Compounds by Ammonia-Oxidizing Bacteria, Crit. Rev. Biochem. Mol. Biol., 2003, 38, 471–495 CrossRef PubMed.
- L. Y. Stein and Y. L. Yung, Production, Isotopic Composition, and Atmosphereic Fate of Biologically Produced Nitrous Oxide, Annu. Rev. Earth Planet. Sci., 2003, 31, 329–356 CrossRef.
- M. Whittaker, D. Bergmann, D. Arciero and A. B. Hooper, Electron Transfer during the Oxidation of Ammonia by the Chemolithotrophic Bacterium Nitrosomonas europaea, Biochim. Biophys. Acta, 2000, 1459, 346–355 CrossRef.
- G. L. Squadrito and W. A. Pryor, The Formation of Peroxynitrite in vivo from Nitric Oxide and Superoxide, Chem.-Biol. Interact., 1995, 96, 203–206 CrossRef PubMed.
- F. Boschetto, N. Toyama, S. Horiguchi, R. M. Bock, B. J. McEntire, T. Adachi, E. Marin, W. Zhu, O. Mazda, B. S. Bal and G. Pezzotti,
In vitro antibacterial activity of oxide and non-oxide bioceramics for arthroplastic devices: II. In situ time-lapse Fourier transform infrared spectroscopy, Analyst, 2018, 143, 2128–2140 RSC.
- J. Depasse and J. Warlus, Relation between the Toxicity of Silica and its Affinity for Tetraalkylammonium Groups. Comparison between Si02 and Ti02, J. Colloid Interface Sci., 1976, 56, 618–621 CrossRef.
- F. C. Fang, Mechanisms of Nitric Oxide-Related Antimicrobial Activity, J. Clin. Invest., 1997, 99, 2818–2825 CrossRef PubMed.
- H. Rubbo, R. Radi, M. Trujillo, R. Telleri, B. Kalyanaraman, S. Barnes, M. Kirk and B. A. Freeman, Nitric Oxide Regulation of Superoxide and Peroxynitrite-dependent Lipid Peroxidation: Formation of Novel Nitrogen-containing Oxidized Lipid Derivatives, J. Biol. Chem., 1994, 269, 26066–26075 Search PubMed.
- W. G. Zumft, The Biological Role of Nitric Oxide in Bacteria, Arch. Microbiol., 1993, 160, 253–264 CrossRef PubMed.
- D. Häussinger, B. Görg, R. Reinehr and F. Schliess, Protein Tyrosine Nitration in Hyperammonemia and Hepatic Encephalopathy, Metab. Brain Dis., 2005, 20, 285–294 CrossRef PubMed.
-
D. A. Wink, M. Feelisch, Y. Vodovotz, J. Fukuto and M. B. Grisham, The Chemical Biology of Nitric Oxide, in React. Oxyg. Species Biol. Syst., ed. C. Colton and D. Gilbert, Springer, Boston, MA USA, 2002, pp. 245–291 Search PubMed.
- R. Raulli, G. McElhaney-Feser, J. Hrabie and R. Cihlar, Antimicrobial properties of nitric oxide using diazeniumdiolates as the nitric oxide donor, Recent Res. Dev. Microbiol., 2002, 6, 177–183 Search PubMed.
- H. Ischiropoulos, Protein Tyrosine Nitration-An Update, Arch. Biochem. Biophys., 2009, 484, 117–121 CrossRef PubMed.
- R. Radi, Nitric Oxide, Oxidants, and Protein Tyrosine Nitration, Proc. Natl. Acad. Sci. U. S. A., 2004, 101, 4003–4008 CrossRef PubMed.
- N. Abello, H. A. M. Kerstjens, D. S. Postma and R. Bischoff, Protein Tyrosine Nitration: Selectivity, Physicochemical and Biological Consequences, Denitration, and Proteomics Methods for the Identification of Tyrosine-Nitrated Proteins, J. Proteome Res., 2009, 8, 3222–3238 CrossRef PubMed.
- R. Radi, Protein Tyrosine Nitration: Biochemical Mechanisms and Structural Basis of its Functional Effects, Acc. Chem. Res., 2013, 46, 550–559 CrossRef PubMed.
- A. J. Gow, D. Duran, S. Malcolm, H. Ischiropoulos and J. Gow, Effects of Peroxynitrite-Induced Phosphorylation Protein Modifications and Degradation on Tyrosine, FEBS Lett., 1996, 385, 63–66 CrossRef PubMed.
- B. Fubini, E. Giamello, M. Volante and V. Bolis, Chemical Functionalities at the Silica Surface Determining its Reactivity when inhaled, Formation and Reactivity of Surface Radicals, Toxicol. Ind. Health, 1990, 6, 571–598 Search PubMed.
-
M. Repetto, J. Semprine and A. Boveris, Lipid Peroxidation: Chemical Mechanism, Biological Implications and Analytical Determination, in Lipid Peroxidation, ed. A. Catala, INTECH Open Access, Rijeka, Croatia, 2012, pp. 3–30 Search PubMed.
- G. Greco and T. Letzel, Main Interactions and Influences of the Chromatographic Parameters in HILIC Separations, J. Chromatogr. Sci., 2013, 51, 684–693 Search PubMed.
- S. Tsuneda, H. Aikawa, H. Hayashi and A. Hirata, Significance of Cell Electrokinetic Properties Determined by Soft-Particle Analysis in Bacterial Adhesion onto a Solid Surface, J. Colloid Interface Sci., 2004, 279, 410–417 CrossRef PubMed.
- R. Kumar, A. Umar, G. Kumar and H. S. Nalwa, Antimicrobial Properties of ZnO Nanomaterials: A Review, Ceram. Int., 2017, 43, 3940–3961 CrossRef.
- D. O. Schairer, J. S. Chouake, J. D. Nosanchuk and A. J. Friedman, The Potential of Nitric Oxide Releasing Therapies as Antimicrobial Agents, Virulence, 2012, 3, 271–279 CrossRef PubMed.
- M. Katsikogianni and Y. F. Missirlis, Concise Review of Mechanisms of Bacterial Adhesion to Biomaterials and of Techniques Used in Estimating Bacteria-Material Interactions, Eur. Cells Mater., 2004, 8, 37–57 CrossRef PubMed.
- Y. F. Missirlis and M. Katsikogianni, Theoretical and Experimental Approaches of Bacteria-Biomaterial Interactions, Materwiss. Werksttech., 2007, 38, 983–994 CrossRef.
|
This journal is © The Royal Society of Chemistry 2018 |