DOI:
10.1039/C7TB01241A
(Review Article)
J. Mater. Chem. B, 2017,
5, 5714-5725
Contrast agents for cardiovascular magnetic resonance imaging: an overview
Received
7th May 2017
, Accepted 4th July 2017
First published on 4th July 2017
Abstract
Cardiovascular Magnetic Resonance (CMR), a non-invasive and nonionizing imaging technique, plays a major role in research and clinical cardiology. The strength of CMR lies in its high temporal resolution, superior contrast, and unique tissue characterization capabilities. Contrast agents have been used to improve sensitivity and specificity of CMR in detecting and evaluating various pathologies. Much effort has been made to develop more efficient contrast reagents to detect cardiovascular diseases at an asymptomatic stage, which has led to a plethora of products in animal studies. However, very few of the developed contrast agents are currently approved for human use. Major obstacles are high dosages, toxicity, body clearance rate and long-term immunogenicity. In this review, we critically assess recent developments in the field of the contrast agents for CMR, highlighting both benefits and current drawbacks. A clearer insight regarding the challenges facing the development of improved contrast agents may help collaborative work to enhance images contrast, decrease toxicity and accelerate their translation into clinical use.

Marco M. Meloni
| Dr Marco M. Meloni graduated in Italy at the University of Sassari under the supervision of Professor M. Taddei. He gained his PhD at Southampton University with Professor R. C. D. Brown, developing a novel class of silicon linkers for solid phase organic synthesis. He was then a PDRA at Manchester and Sheffield Universities working with Professors S. Faulkner, S. Flitsch and S. Jones, developing targeted contrast reagents for Molecular Imaging and glycobiology. He currently holds a BHF fellowship at St George's University of London and honorary fellowships at Kingston University and UCL, under the supervision of Drs T. He, S. Barton and W. Song, developing novel contrast reagents for molecular imaging of cardiovascular diseases. |
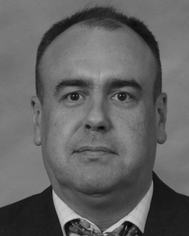
Stephen Barton
| Dr Stephen Barton CSci, CChem, MRSC is Associate Professor in Pharmaceutical Analysis at Kingston University, UK. His first degree is in chemistry and he gained a PhD in the diffusion characteristics and electrical properties of novel thermosetting resins from Kingston University. His current research interests include synthesis and properties of conducting polymer blends and analysis of pharmaceutical degradation studies using NMR and LC-MS. |

Lei Xu
| Professor of Radiology, Department of Radiology, Beijing Anzhen Hospital, Capital Medical University, China. He gained his MD and PhD degrees in diagnostic radiology from Capital Medical University in China. His research interests focus on cardiovascular CT and cardiovascular MRI. |
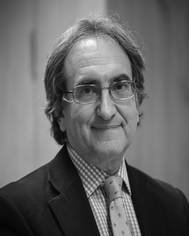
Juan C. Kaski
| J. C. Kaski, DSc, MD, DM (Hons), FRCP, FESC, FACC, FAHA, is Professor of Cardiovascular Science at St George's, University of London (SGUL), Honorary Consultant Cardiologist at St George's Hospital, London, UK, Immediate Past Director of the Cardiovascular and Cell Sciences Research Institute at SGUL and Past-President of ISCP. Prof. Kaski is Doctor of Science (University of London), Gold Medallist (Spanish Society of Cardiology and Fukushima University in Japan) and Doctor Honoris Causa of several universities worldwide. Professor Kaski is editorial board member of JACC and the European Heart Journal among 20 other peer review journals. He is Editor-in-Chief of European Cardiology Review Journal and the ISCP Pharmacotherapy series and Deputy Editor, International Journal of Cardiology. Prof. Kaski has published over 450 papers in peer-review journals, over 300 invited papers and book chapters and edited six books. |

Wenhui Song
| Wenhui Song received her PhD from the University of Cambridge in UK, BEng and MEng from Beihang University in China. She is currently Reader and Director of the Centre for Biomaterials in the Division of Surgery and Interventional Science, University College London. Her research is primarily focused on three main areas: polymeric biomaterials, nanomaterials and nanocomposites for drug delivery and regenerative medicines, scaffolds for tissue regeneration and artificial organs, and implantable sensors and devices. Her laboratory is also developing novel bio-manufacturing technologies, such as engineered self-assembling, 3D bioprinting, and electrospinning for production of nanomedicines, scaffolds, implants, sensors and devices. |
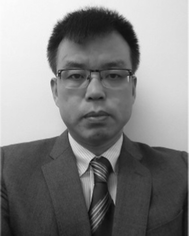
Taigang He
| Dr Taigang He is currently a senior lecturer of cardiovascular imaging at St George's, University of London. He also holds an honorary position at Imperial College London. He aims to help clinicians increase detection rates, reduce healthcare costs, and most importantly save lives by using novel imaging techniques and emerging big data analytics. His research interests include cardiovascular magnetic resonance, myocardial tissue characterization, medical image computing, and artificial intelligence in biomedical and health Informatics. |
1. Introduction
Cardiovascular diseases (CVDs) are a group of disorders of the heart and blood vessels and remain the biggest cause of deaths worldwide, representing 31% of all deaths.1 Cardiovascular imaging plays a pivotal role in modern health care and constitutes an essential component in the management of patients with cardiovascular conditions. Current imaging modalities for assessment of CVDs include ultrasonography, Positron Emission Tomography (PET), Computed Tomography (CT) and Magnetic Resonance Imaging (MRI). Since the pioneering work of Lauterbur and Mansfield in the 1970s,2 MRI has become a staple of imaging modality in medical science and clinical practice. For the heart and vascular system, the term CMR is often used. To note, although it is often routinely used for CVS imaging, CT is an X-ray based modality known for its radiation problems. By contrast, CMR does not use radiation and has no known side effects. CMR also affords superb soft tissue contrast, providing a comprehensive assessment of cardiac morphology, function, perfusion, viability, coronary artery stenosis and quantitative tissue characterisation.3 In view of these capabilities, CMR is often known as the “one-stop shop” for virtually any form of cardiovascular disease.
Because the endogenous differences between tissues in MRI can be small, a contrast agent (CA) is often used in MRI to provide additional contrast to distinguish a target tissue from its surroundings. In 1988, the first CA specifically designed for MRI, a complex of gadolinium ion (Gd3+) and 1,4,7,10-tetraazacyclododecane-1,4,7,10 carboxylic acid (Gd–DOTA), became available for clinical use.4 Since then, many and varied CAs have been developed to increase the sensitivity and specificity of detecting and evaluating various pathologies. In CMR, CAs have played a key role in a variety of applications such as perfusion,5 viability,6 tissue characterisation,7 angiography8–10 and more recently molecular imaging studies.11 Today, the CAs development continues to evolve, bringing exciting opportunities for more sensitive and targeted imaging to improve patient outcome, along with associated challenges.
Many CAs developed are however limited to proof-of-concept preclinical studies, and very few of them are currently approved for human use. In our opinion, the major obstacles include high dosages, toxicity, body clearance rate and long-term immunogenicity. In this review, instead of a detailed description of its history and clinical applications, we aim at critically assessing recent developments of CA in the field of CMR, highlighting both benefits and drawbacks. An insight into challenges facing the CAs' development may promote coordinated effort in producing improved CMR agents and accelerating translation of the development into clinical use.
2. Basics of tissue contrast in CMR
CMR scanners use strong magnetic fields, radiofrequency waves, and field gradients to generate images of the heart. The radiofrequency emission is tissue dependent which give rise to the unparalleled ability of CMR to distinguish subtle differences in the cardiovascular system. Briefly speaking, natural or intrinsic tissue contrast is due to difference in the measured signals which are majorly determined by four parameters in CMR: proton density, T1, T2, and flow. Proton density presents its concentration in tissue in the form of water and macromolecules (proteins, fat, etc.). The T1 and T2 relaxation define the way that the excited protons revert back to their equilibrium states. The effect of flow may be complex but the most common one is loss of signal from rapidly flowing blood. CMR can produce tailored contrast for a certain pathological condition by optimising these parameters in a pulse sequence. We herein refer to Manning and Pennell's monograph12 for physics principles and a comprehensive description of CMR.
Even though CMR has a high contrast sensitivity relative to most other imaging modalities, the intrinsic tissue characteristics can overlap for example between normal, reversibly and irreversibly damaged heart. In this scenario, CAs can be used to enhance the intrinsic characteristics within specific tissues or region of interest.
CAs for CMR can be classified according to various features like the presence of a metal ion centre (usually Gd3+), the ability to alter preferentially T1 or T2, their affinity for CVS, their effect on image, or their chemical structures. As these features are intimately related, a unique classification is unlikely.
The affinity of a CA for CVS is probably the most known property by the scientific community. We will use this feature to differentiate CAs between conventional and molecular. Conventional CAs are untargeted and passively absorbed in the damaged areas of CVS whilst molecular CAs target specific biomolecules expressed in the CVS during disease development.
Given the growing interest in CVDs at a molecular level, the field of molecular CAs is rapidly emerging. The synergistic combination of CMR and molecular imaging is expected to provide a much better contrast of diseased CVS, providing invaluable information on atherogenesis processes. Albeit on its infancy, research in this field has been enormous and already led to a plethora of targeted T1 and T2-based contrast reagents.
3. Developing efficient contrast agents: challenges and key factors
3.1 The balance between dosage and side effects
Generally speaking, a good CA needs to afford higher contrast of diseased CVS, but with a dosage at which virtually no short and long-term toxicity are encountered. Current CA dosage for CMR can be up to 0.3 mmol kg−1 M.13,14 Dosages over 0.3 mmol kg−1 may provide a further improvement, however, there is a great concern over increased toxicity. Serious acute and chronic effects have been reported even at clinically approved dosage, and a notorious example is the so-called Nephrogenic Systemic Fibrosis (NSF).15,16 Additionally, a recent concern is the possible adverse effect of Gd accumulation in patients' brains.17–19 The clinical significance of this remains unclear, nevertheless in the absence of sufficient evidence, the lowest possible dosage of Gd-based CAs is highly recommended.
3.2 The chemical challenge
From a chemist's perspective, the synthesis of more efficient CAs faces a great challenge: it must be simple, reliable, time saving, high yielding, and scalable at least to kilogram scale. Given the growing need of CAs for CMR, this challenge will become more and more prominent in the future.
The recent merging molecular CMR also leads to the development of the so-called targeted CAs. Compared to conventional CAs, these agents are more specific by binding key biomolecules expressed during CVDs to generate a better contrast. However, synthesis of these agents is usually laborious and time consuming, and low yields are usually encountered requiring novel protocols and optimization. If such challenges are addressed, molecular CMR can be implemented in a cost and time-effective fashion, representing a significant contribution alongside traditional CMR.
3.3 Key factors
When facing the aforementioned challenges, the researcher must consider several key properties of a promising CA: its ability to alter T1 and T2, body retention and clearance, side effects and the capability to accumulate preferentially in damaged CVS. A typical example is Late Gadolinium Enhancement (LGE), a well-known CMR protocol,20 which illustrates the importance of these factors. LGE heavily relies on a passive accumulation of the CA in damaged CVS, therefore a contrast medium with slow body clearance will have a great impact in lowering the dose, minimising side effects and addressing the challenges mentioned in the previous sections.21
3.4 The impact of molecular structure
The chemical structure of a CA is fundamental for understanding its mode of action. By using the current CAs as a lead and the Structure–Activity Relationship (SAR), synthetic and medicinal chemists can predict and design improved CAs by tuning their capability to alter T1 and T2, increase their stability22 in the bloodstream and improve specificity for damaged CVS. Chemists can also design novel synthetic routes of promising CAs in a cost-effective fashion. A clear example of SAR importance emerged in the 1980s with the advent of the first generation of Gd-based CAs, which will be described more in details in Section 4.1.1.
4. Conventional contrast reagents for CMR
Conventional CAs can alter preferentially T1 or T2 and will be described respectively in Sections 4.1 and 4.2. To note, CAs alter both T1 and T2 of the water protons; however, such effects are usually more pronounced for either T1 or T2, and it is their relative ratio that influence the categorization as either T1 or T2 based CAs.
4.1
T
1-Based contrast agents: general remarks
T
1-Based agents are the first class of CAs historically investigated for CMR. These agents shorten the relaxation time of surrounding water protons, and are called “positive” agents because they produce image brightening in T1-weighted imaging sequences. The paramagnetic Gd3+ has a high magnetic moment (μ2 = 63 BM2) and is currently the metal ion of choice. However, free Gd3+ is well-known to be cytotoxic and retained in liver, spleen and bone.23–25 Due to its similarity with Ca2+ in atomic radius, Gd3+ also binds ion channels and biomolecules like calmodulin which mediates many crucial processes in the body such as metabolism, apoptosis, smooth muscle contraction, short-long-term memory and the immune response. To decrease its toxicity, the Gd3+ needs to be held tightly by an organic ligand to form stable complexes or chelates. Recent preclinical studies showed that paramagnetic Mn2+-based CAs have also emerged as safer alternatives and these are, potentially more compatible with renally compromised patients.26
Conventional, T1-based CAs can be further divided into extracellular, blood pool, multinuclear CAs. These are all T1-based, but differ in chemical structure, size and different number of Gd3+ per molecule.
4.1.1 Extracellular contrast agents.
Extracellular CAs are so-called as they are not internalised by the body cells. Upon intravenous injection, these agents randomly distribute in intravascular and interstitial spaces and are excreted rapidly by the kidneys in their unchanged forms. Benefits include simple and inexpensive synthesis, relatively low toxicity and fast body clearance. The synthesis of these CAs involves chelation of the Gd3+ with acyclic or macrocyclic multidentate ligands containing multiple carboxylate anions (Fig. 1). The complexes are kinetically and thermodynamically stable, resulting in the minimal amount of free Gd3+ release in the body. The chemical structure of the ligand plays a crucial role in the overall toxicity.27 CAs based on macrocyclic ligands (Gd–DOTA, Gd-DO3A butrol and Gd-HP-DO3A) are more stable compared to their acyclic counterparts based on Diethylene Triamine Pentaacetic Acid (Gd-DTPA) because the so-called macrocyclic effect lead to a lower release of free Gd3+ in the body.
 |
| Fig. 1 First generation of T1-based contrast agents used for CMR. | |
Gd–DOTA showed enhancement on carotid vulnerable plaques related to inflammatory process28,29 as well as Gd-DTPA30,31 and Gd-DO3A-butrol,32 both at preclinical and clinical levels. Gd-DTPA also afforded enhanced contrast in the coronary artery wall of subjects after Acute Myocardium Infarction (AMI) compared to normal subjects six days after infarction.33 Since the introduction of Gd–DOTA in 1988, macrocyclic CAs have been extensively used to enhance the signal in CMR scans for the last three decades.
These agents also image non-cardiovascular related diseases like inflammatory edema and tumor angiogenesis.34 This may lead to false diagnosis of CVD, which prompts further development of CAs aimed specifically to damaged CVD.
4.1.2 Blood pool contrast agents.
Blood pool is often referred to as a blood deposit that occurs on walls and valves of veins when they work ineffectively, thereby making it difficult for blood to return to the heart. Blood pool CAs are a valuable solution compared to the first generation of CAs. These agents are still extracellular and are specifically used for cardiovascular applications. The T1-shortening capability in the damaged CVS was initially thought to be the main reason for the improved contrast. However, subsequent studies showed that such enhancement is also due to a higher molecular size which causes extended retention in the bloodstream; this results is a preferential absorption and passive accumulation of the CAs into atherosclerotic plaques via enhanced permeability and retention effect.35,36 This phenomenon is due to neovessels formation in the endothelial lesions during the atherosclerotic cascade. Given the growing importance of LGE in assessing damaged myocardium,37 research and development of such agents has been very intense. A recent example is gadofosveset (MS-325)8,38 which is currently used in patients39 with carotid artery stenosis for the detection of vulnerable plaque features. The superior capability of generating enhanced contrast was clearly demonstrated in a clinical trial, where LGE images of chronic myocardial infarction was compared using both MS-325 and Gd-DTPA. It was found that the accuracy of LGE was higher than MS-325 54 minutes after contrast injection, resulting in a sensitivity and specificity of 84% and 98% respectively.40 Another promising example is gadofluorine M,41 which affords enhanced images in aortic42,43 and femoral plaques of atherosclerotic rabbits.44 Independent studies45,46 demonstrated that upon injection in the bloodstream, gadofluorine M self-assembles in small micelles that bind the albumin present in the body, before penetrating into the atherosclerotic plaques; once there it passively accumulates in the fibrous cap.
Recent studies demonstrated that also gadofosveset,9 Gd-B-22956/147 and CB-Gd-DOTA-MA48 work via the same albumin-binding mechanism. In particular, Gd-B-22956/1 has a high affinity to serum albumin and generates high contrast enhancement in atherosclerotic plaques correlated with both neovessel and macrophages density.49 An improved novel blood pool CA, GdAAZTA-MADEC was recently developed and tested at a preclinical model,50 affording an enhanced contrast of damaged CVS compared to gadofosveset and B25716/1 at 1 T. Herein, the presence of a hydrophobic spacer between the deoxycholic acid moiety and the Gd-AAZTA unit results in a stronger binding with albumin, hence affording a better contrast. Another promising contrast agent, P792, has been used for aortic arch and carotid imaging and is on phase III clinical development.51,52 Gd-DTPA-BMA allowed enhanced imaging of both necrotic core, calcification and loose matrix, all key components in unstable plaques.53 A comparative study on the morphological characterization of the carotid plaques has been carried out with Gd-BOPTA54 and Gd-DTPA-BMA, and it was found that the choice of the contrast agent has little impact.55 Other agents for enhanced contrast of vascular tissues and plaques identification are Gd-EOB-DTPA,56,57 Gd-Motexafin6 and Gd-AAZTA-C17.7Fig. 2 shows some common blood pool CAs.
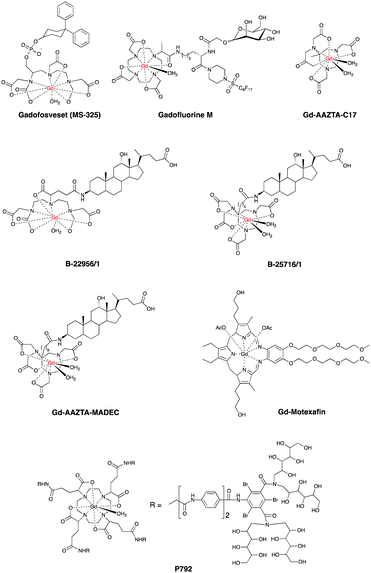 |
| Fig. 2 Most common blood pool contrast CAs for clinical and preclinical CMR. | |
These findings suggest that chemists, clinicians and toxicologists will need to use these CAs as a lead to develop novel systems. It is anticipated that ligand design and synthesis will play a key role to obtain the next generation of blood pool CAs by further altering T1 and increasing passive retention on damaged areas of CVS.58
So far, all CAs described herein have been designed to provide a contrast which is optimal for the vast majority of magnetic fields used in clinical CMR (up to 1.5 T). Higher magnetic fields severely compromise image contrast as T1 is reduced up to one-third compared to its maximum. This feature could be a long term limitation, given the recent advents of high field clinical scanners (3 T).
On the other hand, higher field MRI introduces serious safety considerations: higher power radiofrequency pulses, potential tissue heating, coil burns and, most prominently, the dangers associated with a stronger magnetic field, such as ferromagnetic materials and implanted medical devices in the patients, many of which have not been evaluated at fields above 1.5 T.
Given these limitations there is still an enormous effort to develop alternative CAs to provide enhanced contrast with 1.5 T-based clinical scanners.
4.1.3 Multinuclear contrast agents.
A recent and promising solution are the so-called multinuclear CAs. Different from the aforementioned CAs, these agents contain more than one Gd3+ centre per molecule and have some advantages compared to their mononuclear counterparts. Their higher molecular weight allows a better retention in atherosclerotic plaques,59 whereas the presence of multiple Gd3+ centers will be more efficient in altering T1.
One of the most advanced CAs in clinical development is Gadomer 1760 (Fig. 3, page 6), which is a dendritic chelate carrying 24 Gd3+ centers.10,61,62
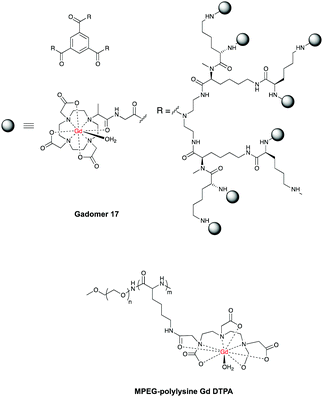 |
| Fig. 3 Structures of Gadomer 17 and MPEG polylysine DTPA polymers. | |
After intravenous injection Gadomer-17 distributes almost exclusively within the intravascular space, providing enhanced contrast of coronary arteries in patients with Coronary Artery Disease (CAD)63 and in a swine model of myocardial perfusion.5
Another example is MPEG-polylysine-DTPA-Gd3 for an enhanced vessel-muscle contrast where the half-life was shown to be 14 h with a dose of 20 μmol of Gd per kg in preclinical trials.64
Similar to blood pool CAs, the molecular size of multinuclear CAs is too large for capillary extravasation, yet it is small enough for rapid renal elimination, allowing improved images of vessels and decreased toxicity compared to their mononuclear counterparts. Polynuclear micelles containing Gd-DOTAC16, Gd-DTPA-BSA or Gd-DO3A-OA (Fig. 4) have also been prepared and gave enhanced images of macrophages in plaques compared to standard mononuclear agents such as Gd-DTPA.65,66 In another study high density lipoproteins (HDL) containing Gd-DTPA-DMPE showed enhanced imaging of atherosclerotic lesions.67–69
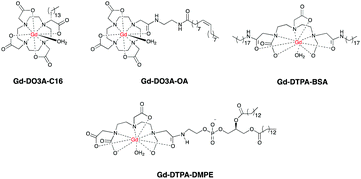 |
| Fig. 4 Multinuclear contrast agents currently developed for CMR. | |
Whilst multinuclear CAs may allow enhanced images of damaged CVS, they also pose serious issues due to potential release of more free Gd3+ in liver, spleen and bones,70 requiring longer and more expensive toxicology tests before their translation into clinical uses. The use of biodegradable multinuclear CAs can be a safer alternative. Preclinical studies showed that after providing enhanced contrast of heart and blood vessels, endogenous enzymes in the body will degrade these CAs into lower molecular weight fragments, which are more easily excreted by the kidneys.71–74
4.2.
T
2-Based contrast agents
4.2.1 General remarks.
T
2-Based CAs mainly shorten the transverse relaxation times of the surrounding water protons and are termed “negative” agents because they produce darker images in T2-weighted imaging sequences.
The ability of iron oxide nanoparticles to alter T2 relaxation times in water was first discovered in 1978.75 Since then iron oxide became the most common T2-based CA currently used. Super Paramagnetic Iron Oxide Nanoparticles (SPIONS, diameter size 50 to 300 nm) and Ultra-small SPIONS (USPIONS, diameter size 15 to 30 nm) are iron oxide nanoparticles that can be coated with dextran, silicates, or other non-immunogenic polymers for preclinical and clinical applications. An advantage of SPIONS over T1-based CAs is their transverse relaxivity which increases at higher fields. This property suggests that SPIONS can be a promising tool for the future, especially with the advent of high field MRI. Additionally, unlike Gd-based CAs, SPIONS have proven safe and are cleared as endogenous iron by the reticulo-endothelial system.
There are many different system containing SPIONS for CVDs.76–79 The next sections will describe them separately, along with recent advances, current challenges and future directions.
4.2.2 Non-specific contrast reagents: SPIONS.
Non-specific SPIONS are primarily addressed to macrophages which accumulate in atherosclerotic lesions and promote the late stage formation of atheroma and atherosclerotic plaques rupture.80
To date, ferumoxtran is one of the most extensively studied CAs for imaging atherosclerotic plaques, both at preclinical and clinical levels.81,82 Ferumoxtran offers a number of advantages: inexpensive synthesis, very good biocompatibility, high affinity for plaque macrophages and a low toxicity. It has been successfully applied to enhance images in stenotic carotid or atheromatous plaques.81–83 In another study84 ferumoxtran has been administered for CMR imaging of symptomatic patients scheduled for carotid endarterectomy, showing enhanced contrast (up to 25%) and high SPION content (up to 75%) in rupture-prone lesions, as confirmed by histology. The optimal post-injection time for imaging symptomatic plaques was found to be between 24 and 36 h.81 The high reliability of ferumoxtran has been used for therapy monitoring in patients treated with different doses of atorvastatin.85,86 Another SPION based agent, Sinerem, also proved very promising in providing enhanced images of macrophages in atherosclerotic plaques of rabbits.87
Despite providing enhanced images of damaged CVS, these CAs lack of tissue specificity. Ferumoxtran is a polysaccharide-coated SPION and can be easily internalized by the macrophages present in other tissues of the body, in particular by the Kupffer cells in the liver.88 Synthetic challenges also arise from controlling the size of the SPIONs, a key factor. Studies showed that smaller nanoparticles (up to 5 nm) would be more advantageous for easier body clearance and decreased toxicity.89–91 Phagocytic cells internalize large particles more effectively, whereas nonphagocytic T cells internalize intermediate-sized particles more efficiently.92–94
To increase the CVS specificity, many research groups investigated different sizes of SPIONS and polymer-coating chemicals. Mannan95 coated SPIONS allowed enhanced images of atherosclerotic walls in rabbits,96 whereas citrate97 and Dextran Sulphate (DS) coated SPIONS provided enhanced contrast of atherosclerotic plaques both in preclinical and clinical studies.98
5. Molecular imaging of CVDs: the emerging role of molecular contrast reagents
5.1 General remarks
Molecular imaging is able to visualize specific biomolecules expressed during a broad range of pathologies. This technique has been increasingly applied to detect CVDs as many biomolecules are expressed de novo during inflammation and endothelial dysfunction. The most important biomolecules known so far are E and P-selectins, integrins, Vascular Cell Adhesion Molecule 1 (VCAM-1), Intercellular Adhesion Molecule 1 (ICAM-1), peroxidases and Matrix Metalloproteinases (MMPs). These molecules promote leukocyte rolling and adhesion on the wall of the inflamed endothelium and their subsequent transmigration into the sub-endothelial space, all important events in the development of CVDs (Fig. 5).99–101
 |
| Fig. 5 The beginning of atherosclerosis. Following an inflammatory response, E and P selectins are expressed and translocated on the endothelial surface, causing leucocytes in the blood stream (blue) to tether, roll and accumulate on the endothelium, triggering the formation of atherosclerotic plaques. | |
Integrin αvβ3 promotes leucocyte extravasation through the extracellular matrix and is heavily involved in atherosclerosis progression and in restenosis. MMPs are a broad family of endopeptidases involved in rupture-prone plaques and play a key role in the degradation and remodelling of the extracellular matrix. Myeloperoxidases (MPO) promote oxidation of low-density lipoprotein (LDL).102 Fibrin is also an important component of atherosclerotic plaques and is present in advanced lesions, whereas elastin is important in vascular remodelling. Low-density Lipoprotein Receptor-1 (LOX-1) triggers inflammation in the endothelium and is expressed in vulnerable plaques.
5.2 Molecular T1-based contrast agents
Targeted T1-based CAs are made by linking three components: the first one is a targeting vector which binds the biomolecule of interest, the second one is a clinical T1 based CA which provides the contrast, and the third one is a linker which holds the first two components together.103 The chemical process aimed to link these components together is called bioconjugation, which generally results in the formation of biologically stable molecular moieties like amides, ethers or thioesters. The enhanced contrast of these CAs derives from both T1 alteration and the specificity of the CA for the biomolecule of interest (Fig. 6). The mode of action is different from the conventional CAs (described in Section 4) which are not targeted to any molecule but accumulate passively in the damaged CVS.
 |
| Fig. 6 Some T1-based targeted contrast agents currently in preclinical development. | |
Compared to conventional CAs, molecular CAs offer many advantages: better specificity, improved contrast and a better understanding of molecular processes behind CVDs. Clinicians and medicinal chemists will use these advantages to develop better drugs and improved therapies. Long term advantages are also detection of CVDs at subclinical levels, identification of in vivo markers for stable and unstable plaques and patient-individualised risk assessment.
Despite the great promise, many challenges still hamper the translation of molecular CAs into clinical use: complex multistep syntheses and optimisation processes are often needed. To obtain the best contrast, bioconjugation must not alter the affinity of the vector for the targeted biomolecule or decrease the probe capability to alter T1. In case of Gd-based molecular CAs, bioconjugation may also lead to the release of free Gd in the body, requiring longer and more expensive toxicology studies.
Given these challenges, research in this field has been very intense and there is a plethora of systems already reported, examples include E and P-selectin imaging with Gd-F-P717, Gd-P717104–107 and Gd-DTPA-BsLexA, respectively.108,109 A targeted Gd-based contrast agent was used to image MMPs activation110 as well as fibrin111–113 with Gd EP-2104R114 and EP-1242.115 Gd-LMI1174116 and BMS753951117 allowed enhanced images of plaques by targeting elastin and has been used to quantify the changes in elastin content in plaques regression in a mouse model of atherosclerosis. Targeted liposomes containing Gd-DTPA successfully imaged low density lipoprotein receptors in ApoE(−/−) mice.118,119
Gd-DTPA-G-R826 is a conjugate containing the peptide sequence LIKKPF: this agent binds the phosphatidylserine receptor of apoptotic macrophages, allowing enhanced images of atherosclerotic plaques.120 A similar peptide-based agent has been reported to image plaque progression.121
Another group developed a Gd-DTPA-mim-RGD agent that allowed enhanced images of inflamed blood vessels by targeting integrin αvβ3 in vulnerable atherosclerotic plaques. The authors also reported a decreased contrast when the analogue, non-targeted Gd-DTPA was used in the same model.122
Contrast agents containing amphiphilic fullerenes C60,123 C70124 have also been recently developed, providing enhanced visualization of specific receptors on foam cells in atherosclerotic plaques compared to mononuclear Gd–DOTA.125
In a similar fashion, Gd-DTPA-MPO provided enhanced contrast by targeting myeloperoxidase activity in mice.126 Finally, P947 is a Gd–DOTA derived agent that afforded enhanced images by targeting the MMPs that accumulate in atherosclerotic lesions.127,128Fig. 6 shows some promising CAs for targeted CMR.
5.3 Molecular T2-based contrast agents
Similar to what has been discussed for T1-based molecular CAs, SPIONS can be targeted to specific biomolecules involved in CVDs. This can be achieved by synthetically modifying the SPIONS coating to allow attachment of targeting vectors. The resulting CAs offer enhanced images of CVS, improved bioavailability and lower toxicity. All these features can facilitate the translation of these systems into clinical use.
The synthetic challenges described in Section 5.2 also apply for molecular T2-based CAs. For these CAs, bioconjugation and formulation constitute even more critical steps as they may cause self-aggregation and precipitation of insoluble CAs both in vitro and in vivo. As nanoparticle-based CAs translate into the clinic, one additional challenge is to maximize the value-to-cost ratio for high volume product scale-up.
Research in this field has been intense, recent examples (Table 1) are contrast agent VINP-28, a peptide-bound nanoparticle with high affinity for VCAM-1 that afforded enhanced contrast in the aortic root of ApoE−/− mice 48 hours after injection.129 The contrast obtained with VINP-28 has been also used for therapy monitoring where the enhanced signal in atherosclerotic plaques strongly decreased after an 8 week treatment with Statin. Further examples of VCAM-1 targeted imaging with SPIONS have been published.130,131
Table 1 Targeted SPIONS currently investigated in preclinical studies
Name |
Ref. |
Molecular target |
VINP-28 |
129
|
VCAM-1 |
SPIONS-antibodies |
133
|
E-selectin, CD44 |
SPIONS-SLeX mimic |
134
|
E-selectin, CD44 |
SPIONS-fumagillin |
136 and 137
|
Integrin |
SPIONS-annexin |
132
|
Phosphatidyl serine |
SPIONS have also been conjugated with antibodies and with a SLex mimics133,134 to detect E-selectins and CD44, both cell-adhesion molecules involved in the early stages of atherosclerosis and in the formation of atherosclerotic plaques.135 In another study, SPIONS were conjugated with fumagillin, an endothelium selective anti-angiogenic drug. The developed agent offers enhanced contrast via targeting integrin αvβ3 in early endothelial inflammation,136 with promising therapeutic applications.137
6. Conclusions
Since the introduction of Gd–DOTA in 1988 there has been a large repertoire of CAs currently available for both preclinical and clinical CMR. However, they offer both advantages and disadvantages.
The first generation of T1-based CAs affords enhanced images of CVS and is straightforward to synthesize. However higher dosages may be required in order to increase CMR sensitivity, which also increases the toxicity due to the release of more free Gd3+ into the body. Blood pool and multinuclear CAs recently emerged as a better option to obtain enhanced contrast of damaged CVS, however their synthesis is less straightforward especially in the case of multinuclear CAs. Nanoparticles hold a promising future in CMR. However, a major limitation is the long post-injection time (24 to 48 hours) which is sometimes needed to obtain an enhanced contrast of CVS. To date, unaddressed challenges include method reproducibility upon scale up syntheses and size control.
Targeted T1 and T2-based CAs hold a great promise for the future of CMR. Distinct from conventional non-targeted CAs, these agents are invaluable in enhancing our understanding of CVDs at molecular level, and helping clinicians to detect cardiovascular abnormalities at subclinical stages. However, both design and synthesis of such agents can be much more complex and expensive.
Last but not the least, it is anticipated that the role of medicinal and synthetic chemists in this field will become more and more important in the future, as the developers have to face all the challenges encountered with design, synthesis, analysis, preclinical and clinical evaluations of these chemical agents.
Conflicts of interest
The authors declare no conflicts of interest.
Acknowledgements
This work is supported by the British Heart Foundation [FS/15/17/31411 to M. M. M.].
References
-
S. Mendis, P. Puska and B. Norrving, Global atlas on cardiovascular disease prevention and control, World Health Organization, 2011 Search PubMed.
- P. Mansfield and A. A. Maudsley, Br. J. Radiol., 1977, 50, 188–194 CrossRef CAS PubMed.
- C. Lang and M. K. Atalay, R. I. Med. J., 2014, 97, 28–34 Search PubMed.
- J. C. Bousquet, S. Saini, D. D. Stark, P. F. Hahn, M. Nigam, J. Wittenberg and J. T. Ferrucci, Jr., Radiology, 1988, 166, 693–698 CrossRef CAS PubMed.
- P. Kellman, M. S. Hansen, S. Nielles-Vallespin, J. Nickander, R. Themudo, M. Ugander and H. Xue, J. Cardiovasc. Magn. Reson., 2017, 19, 43 CrossRef PubMed.
- K. Ramani, R. M. Judd, T. A. Holly, T. B. Parrish, V. H. Rigolin, M. A. Parker, C. Callahan, S. W. Fitzgerald, R. O. Bonow and F. J. Klocke, Circulation, 1998, 98, 2687–2694 CrossRef CAS PubMed.
- T. A. Treibel, S. K. White and J. C. Moon, Curr. Cardiovasc. Imaging Rep., 2014, 7, 9254 CrossRef CAS PubMed.
- S. Kelle, T. Thouet, T. Tangcharoen, K. Nassenstein, A. Chiribiri, I. Paetsch, B. Schnackenburg, J. Barkhausen, E. Fleck and E. Nagel, Med. Sci. Monit., 2007, 13, 469–474 Search PubMed.
- R. B. Lauffer, D. J. Parmelee, S. U. Dunham, H. S. Ouellet, R. P. Dolan, S. Witte, T. J. McMurry and R. C. Walovitch, Radiology, 1998, 207, 529–538 CrossRef CAS PubMed.
- M. Brechbiel, K. Jaspers, B. Versluis, T. Leiner, P. Dijkstra, M. Oostendorp, J. M. van Golde, M. J. Post and W. H. Backes, PLoS One, 2011, 6, e16159 Search PubMed.
- P. U. Atukorale, G. Covarrubias, L. Bauer and E. Karathanasis, Adv. Drug Delivery Rev., 2016 DOI:10.1016/j.addr.2016.09.006.
-
W. J. Manning and D. J. Pennell, in Basic principles of cardiovascular magnetic resonance. Cardiovascular magnetic resonance, Saunders, Philadelphia, 2010, vol. 2, pp. 3–18 Search PubMed.
- T. F. Hany, M. Schmidt, P. R. Hilfiker, P. Steiner, U. Bachmann and J. F. Debatin, J. Magn. Reson. Imaging, 1998, 8, 901–906 CrossRef.
- M. F. Bellin and A. J. Van Der Molen, Eur. J. Radiol., 2008, 66, 160–167 CrossRef PubMed.
- T. Frenzel, P. Lengsfeld, H. Schirmer, J. Hutter and H. J. Weinmann, Invest. Radiol., 2008, 43, 817–828 CrossRef CAS PubMed.
- F. G. Shellock and E. Kanal, J. Magn. Reson. Imaging, 1999, 10, 477–484 CrossRef CAS PubMed.
- C. Olchowy, K. Cebulski, M. Lasecki, R. Chaber, A. Olchowy, K. Kalwak and U. Zaleska-Dorobisz, PLoS One, 2017, 12, e0171704 Search PubMed.
- T. Kanda, K. Ishii, H. Kawaguchi, K. Kitajima and D. Takenaka, Radiology, 2013, 270, 834–841 CrossRef PubMed.
- T. Kanda, Y. Nakai, H. Oba, K. Toyoda, K. Kitajima and S. Furui, Magn. Reson. Imaging, 2016, 34, 1346–1350 CrossRef CAS PubMed.
- O. P. Simonetti, R. J. Ki, D. S. Fien, H. B. Hillenbrand, E. Wu, J. M. Bundy, J. P. Finn and R. M. Judd, Radiology, 2001, 218, 215–223 CrossRef CAS PubMed.
- A. Doltra, B. H. Amundsen, R. Gebker, E. Fleck and S. Kelle, Curr. Cardiol. Rev., 2013, 3, 185–190 CrossRef.
- F. Dioury, A. Duprat, G. Dreyfus, C. Ferroud and J. Cossy, J. Chem. Inf. Model., 2014, 54, 2718–2731 CrossRef CAS PubMed.
- K. N. Christensen, C. U. Lee, M. M. Hanley, N. Leung, T. P. Moyer and M. R. Pittelkow, J. Am. Acad. Dermatol., 2011, 64, 91–96 CrossRef CAS PubMed.
- World Health Organization. Pharmaceuticals: Restrictions in Use and Availability, WHO/EMP/QSM/2010.3, Geneva, Switzerland, 2010, p. 14.
- C. D. Wiginton, B. Kelly, A. Oto, M. Jesse, P. Aristimuno, R. Ernst and G. Chaljub, Am. J. Roentgenol., 2008, 190, 1060–1068 CrossRef PubMed.
- E. M. Gale, I. P. Atanasova, F. Blasi, I. Ay and P. Caravan, J. Am. Chem. Soc., 2015, 137, 15548–15557 CrossRef CAS PubMed.
- A. K. Tiwari, H. Ojha, A. Kaul, A. Dutta, P. Srivastava, G. Shukla, R. Srivastava and A. K. Mishra, Chem. Biol. Drug Des., 2009, 74, 87–91 CAS.
- A. Millon, L. Boussel, M. Brevet, J. L. Mathevet, E. Canet-Soulas, C. Mory, J. Y. Scoazec and P. Douek, Stroke, 2012, 43, 3023–3028 CrossRef PubMed.
- L. Boussel, G. Herigault, M. Sigovan, R. Loffroy, E. Canet-Soulas and P. C. Douek, J. Magn. Reson. Imaging, 2008, 28, 533–537 CrossRef PubMed.
- A. Phinikaridou, F. L. Ruberg, K. J. Hallock, Y. Qiao, N. Hua, J. Viereck and J. A. Hamilton, Circ. Cardiovasc. Imaging, 2010, 3, 323–332 CrossRef PubMed.
- J. A. Ronald, Y. Chen, A. J. L. Belisle, A. M. Hamilton, K. A. Rogers, R. A. Hegele, B. Misselwitz and B. K. Rutt, Circ. Cardiovasc. Imaging, 2009, 2, 226–234 CrossRef PubMed.
- J. M. Grimm, K. Nikolaou, A. Schindler, R. Hettich, F. Heigl, C. C. Cyran, F. Schwarz, R. Klingel, A. Karpinska, C. Yuan, M. Dichgans, M. F. Reiser and T. Saam, J. Cardiovasc. Magn. Reson., 2012, 14, 80 CrossRef PubMed.
- T. Ibrahim, M. R. Makowski, A. Jankauskas, D. Maintz, M. Karch, S. Schachoff, W. J. Manning, A. Schömig, M. Schwaiger and R. M. Botnar, JACC: Cardiovasc. Imaging, 2009, 2, 580–588 CrossRef PubMed.
- H. Hawighorst, P. G. Knapstein, M. V. Knopp, P. Vaupel and G. van Kaick, MAGMA, 1999, 8, 55–62 CAS.
- E. Lobatto, V. Fuster, Z. A. Fayad and W. J. M. Mulder, Nat. Rev. Drug Discovery, 2011, 10, 835–852 CrossRef PubMed.
- J. Fang, H. Nakamura and H. Maeda, Adv. Drug Delivery Rev., 2011, 63, 136–151 CrossRef CAS PubMed.
- A. Varga-Szemes, R. J. van der Geest, B. S. Spottiswoode, P. Suranyi, B. Ruzsics, C. N. De Cecco, G. Muscogiuri, P. M. Cannaò, M. A. Fox, J. L. Wichmann, R. Vliegenthart and U. I. Schoepf, Radiology, 2015, 278, 374–382 CrossRef PubMed.
- P. Caravan, J. J. Ellison, T. J. McMurry and R. B. Lauffer, Chem. Rev., 1999, 99, 2293–2352 CrossRef CAS PubMed.
- S. Tartari, R. Rizzati, R. Righi, A. Deledda, K. Capello, R. Soverini and G. Benea, Am. J. Roentgenol., 2011, 196, 1164–1171 CrossRef PubMed.
- T. Thouet, B. Schnackenburg, T. Kokocinski, E. Fleck, E. Nagel and S. Kelle, Sci. World J., 2012, 2012, 1–6 CrossRef PubMed.
- M. Sirol, P. R. Moreno, K. R. Purushothaman, E. Vucic, V. Amirbekian, H.-J. Weinmann, P. Muntner, V. Fuster and Z. A. Fayad, Circ. Cardiovasc. Imaging, 2009, 2, 391 CrossRef PubMed.
- M. Sirol, V. V. Itskovich, V. Mani, J. G. S. Aguinaldo, J. T. M. Fallon, B. Misselwitz, H. J. Weinmann, V. Fuster, J. F. Toussaint and Z. A. Fayad, Circulation, 2004, 109, 2890–2896 CrossRef CAS PubMed.
- J. Barkhausen, W. Ebert, C. Heyer, J. F. Debatin and H.-J. Weinmann, Circulation, 2003, 108, 605–609 CrossRef CAS PubMed.
- J. Zheng, E. Ochoa, B. Misselwitz, D. Yang, I. El Naqa, P. K. Woodard and D. Abendschein, Invest. Radiol., 2008, 43, 49–52 CrossRef PubMed.
- J. Meding, M. Urich, K. Licha, M. Reinhardt, B. Misselwitz, Z. A. Fayad and H. J. Weinmann, Contrast Media Mol. Imaging, 2007, 2, 120–129 CrossRef CAS PubMed.
- R. Uppal and P. Caravan, Future Med. Chem., 2010, 2, 451–470 CrossRef CAS PubMed.
- C. Parolini, M. Busnelli, G. S. Ganzetti, F. Dellera, S. Manzini, E. Scanziani, J. L. Johnson, C. R. Sirtori and G. Chiesa, Mol. Imaging, 2014, 13, 1–9 CAS.
- L. N. Goswami, Q. Cai, L. Ma, S. S. Jalisatgi and M. F. Hawthorne, Org. Biomol. Chem., 2015, 13, 8912–8918 CAS.
- J. C. Cornily, F. Hyafil, C. Calcagno, K. C. Briley-Saebo, J. Tunstead, J. G. S. Aguinaldo, V. Mani, V. Lorusso, F. M. Cavagna and Z. A. Fayad, J. Magn. Reson. Imaging, 2008, 27, 1406–1411 CrossRef PubMed.
- D. L. Longo, F. Arena, L. Consolino, P. Minazzi, S. Geninatti-Crich, G. B. Giovenzana and S. Aime, Biomaterials, 2016, 75, 47–57 CrossRef CAS PubMed.
- L. Vander Elst, I. Raynal, M. Port, P. Tisnès and R. N. Muller, Eur. J. Inorg. Chem., 2005, 1142–1148 CrossRef CAS.
- H. Alsaid, M. Sabbah, Z. Bendahmane, O. Fokapu, J. Felblinger, C. Desbleds-Mansard, C. Corot, A. Briguet, Y. Crémillieux and E. Canet-Soulas, Magn. Reson. Med., 2007, 58, 1157–1163 CrossRef PubMed.
- W. Liu, N. Balu, J. Sun, X. Zhao, H. Chen, C. Yuan, H. Zhao, J. Xu, G. Wang and W. S. Kerwin, J. Magn. Reson. Imaging, 2012, 35, 812–819 CrossRef PubMed.
- F. Uggeri, S. Aime, P. L. Anelli, M. Botta, M. Brocchetta, C. de Haeen, G. Ermondi, M. Grandi and P. Paoli, Inorg. Chem., 1995, 34, 633–643 CrossRef CAS.
- W. S. Kerwin, X. Zhao, C. Yuan, T. S. Hatsukami, K. R. Maravilla, H. R. Underhill and X. Zhao, J. Magn. Reson. Imaging, 2009, 30, 35–40 CrossRef PubMed.
- G. Schuhmann-Giampieri, H. Schmitt-Willich, W. R. Press, C. Negishi, H. J. Weinmann and U. Speck, Radiology, 1992, 183, 59–64 CrossRef CAS PubMed.
- L. V. Elst, F. Maton, S. Laurent, F. Seghi, F. Chapelle and R. N. Muller, Magn. Reson. Med., 1997, 38, 604–614 CrossRef.
- G. H. Lee, Y. Chang and T. J. Kim, Eur. J. Inorg. Chem., 2012, 1924–1933 CrossRef CAS.
- A. N. Oksendal and P.-A. Hals, J. Magn. Reson. Imaging, 1993, 3, 157–165 CrossRef CAS PubMed.
- B. Misselwitz, H. Schmitt-Willich, W. Ebert, T. Frenzel and H. J. Weinmann, MAGMA, 2001, 128–134 CrossRef CAS.
- J. Tang, Y. Sheng, H. Hu and Y. Shen, Prog. Polym. Sci., 2013, 38, 462–502 CrossRef CAS.
- S. Langereis, A. Dirksen, T. M. Hackeng, M. H. P. van Genderen and E. W. Meijer, New J. Chem., 2007, 31, 1152–1160 RSC.
- G. A. Krombach, C. B. Higgins, M. Chujo and M. Saeed, Radiology, 2005, 236, 510–518 CrossRef PubMed.
- A. A. Bogdanov, R. Weissleder, H. W. Frank, A. V. Bogdanova, N. Nossif, B. K. Schaffer, E. Tsai, M. I. Papisov and T. J. Brady, Radiology, 1993, 187, 701–706 CrossRef CAS PubMed.
- M. J. Lipinski, V. Amirbekian, J. C. Frias, J. G. S. Aguinaldo, V. Mani, K. C. Briley-Saebo, V. Fuster, J. T. Fallon, E. A. Fisher and Z. A. Fayad, Magn. Reson. Med., 2006, 56, 601–610 CrossRef PubMed.
- W. Chen, D. P. Cormode, Y. Vengrenyuk, B. Herranz, J. E. Feig, A. Klink, W. J. M. Mulder, E. A. Fisher and Z. A. Fayad, JACC: Cardiovasc. Imaging, 2013, 6, 373–384 CrossRef PubMed.
- K. C. Briley-Saebo, S. Geninatti-Crich, D. P. Cormode, A. Barazza, W. J. M. Mulder, W. Chen, G. B. Giovenzana, E. A. Fisher, S. Aime and Z. A. Fayad, J. Phys. Chem. B, 2009, 113, 6283–6289 CrossRef CAS PubMed.
- K. C. Briley-Saebo, V. Amirbekian, V. Mani, J. G. S. Aguinaldo, E. Vucic, D. Carpenter, S. Amirbekian and Z. A. Fayad, Magn. Reson. Med., 2006, 56, 1336–1346 CrossRef CAS PubMed.
- J. C. Frias, K. J. Williams, E. A. Fisher and Z. A. Fayad, J. Am. Chem. Soc., 2004, 126, 16316–16317 CrossRef CAS PubMed.
- S. Aime and P. Caravan, J. Magn. Reson. Imaging, 2009, 30, 1259–1267 CrossRef PubMed.
- Z. R. Lu, A. M. Mohs, Y. Zong and Y. Feng, Int. J. Nanomed., 2006, 1, 31–40 CrossRef CAS PubMed.
- A. M. Mohs, Y. Zong, J. Guo, D. L. Parker and Z.-R. Lu, Biomacromolecules, 2005, 6, 2305–2311 CrossRef CAS PubMed.
- A. M. Mohs, X. Wang, K. C. Goodrich, Y. Zong, D. L. Parker and Z. R. Lu, Bioconjugate Chem., 2004, 15, 1424–1430 CrossRef CAS PubMed.
- T. D. Nguyen, P. Spincemaille, A. Vaidya, M. R. Prince, Z.-R. Lu and Y. Wang, Mol. Pharmaceutics, 2006, 3, 558–565 CrossRef CAS PubMed.
- M. Ohgushi, K. Nagayama and A. Wada, J. Magn. Reson., 1978, 29, 599–601 CAS.
- E. A. Schellenberger, A. Bogdanov, Jr, D. Hogemann, J. Tait, R. Weissleder and L. Josephson, Mol. Imaging, 2002, 1, 102–107 CrossRef CAS PubMed.
- P. Bannas, O. Graumann, P. Balcerak, K. Peldschus, M. G. Kaul, H. Hohenberg, F. Haag, G. Adam, H. Ittrich and F. Koch-Nolte, Mol. Imaging, 2010, 9, 211–222 CAS.
- H. B. Na, I. C. Song and T. Hyeon, Adv. Mater., 2009, 21, 2133–2148 CrossRef CAS.
- T. C. Yeh, W. Zhang, S. T. Ildstad and C. Ho, Magn. Reson. Med., 1993, 30, 617–625 CrossRef CAS PubMed.
- P. Libby, Circulation, 2001, 104, 365–372 CrossRef CAS PubMed.
- R. A. Trivedi, J. M. U-King-Im, M. J. Graves, P. J. Kirkpatrick and J. H. Gillard, Neurology, 2004, 63, 187–188 CrossRef CAS PubMed.
- S. A. Schmitz, M. Taupitz, S. Wagner, K. J. Wolf, D. Beyersdorff and B. Hamm, J. Magn. Reson. Imaging, 2001, 14, 355–361 CrossRef CAS PubMed.
- R. A. Trivedi, J. M. U-King-Im, M. J. Graves, J. J. Cross, J. Horsley, M. J. Goddard, J. N. Skepper, G. Quartey, E. Warburton, I. Joubert, L. Wang, P. J. Kirkpatrick, J. Brown and J. H. Gillard, Stroke, 2004, 35, 1631–1635 CrossRef PubMed.
- M. E. Kooi, V. C. Cappendijk, K. B. J. M. Cleutjens, A. G. H. Kessels, P. J. E. H. M. Kitslaar, M. Borgers, P. M. Frederik, M. J. A. P. Daemen and J. M. A. van Engelshoven, Circulation, 2003, 107, 2453–2458 CrossRef CAS PubMed.
- T. Y. Tang, S. P. S. Howarth, S. R. Miller, M. J. Graves, A. J. Patterson, J. M. U-King-Im, Z. Y. Li, S. R. Walsh, A. P. Brown, P. J. Kirkpatrick, E. A. Warburton, P. D. Hayes, K. Varty, J. R. Boyle, M. E. Gaun, A. Zalewski and J. H. Gillard, J. Am. Coll. Cardiol., 2009, 53, 2039–2050 CrossRef CAS PubMed.
- J. H. F. Rudd, F. Hyafil and Z. A. Fayad, Arterioscler., Thromb., Vasc. Biol., 2009, 29, 1009–1016 CrossRef CAS PubMed.
- S. G. Ruehm, C. Corot, P. Vogt, S. Kolb and J. F. Debatin, Circulation, 2001, 103, 415–422 CrossRef CAS PubMed.
- Z. Zhang, N. Mascheri, R. Dharmakumar and D. Li, Cytotherapy, 2008, 10, 575–586 CrossRef CAS PubMed.
- H. S. Choi, W. Liu, P. Misra, E. Tanaka, J. P. Zimmer, B. Itty Ipe, M. G. Bawendi and J. V. Frangioni, Nat. Biotechnol., 2007, 25, 1165–1170 CrossRef CAS PubMed.
- P. C. Naha, K. C. Lau, J. C. Hsu, M. Hajfathalian, S. Mian, P. Chhour, L. Uppuluri, E. S. McDonald, A. D. Maidment and D. P. Cormode, Nanoscale, 2016, 8, 13740–13754 RSC.
- P. C. Naha, A. A. Zaki, E. Hecht, M. Chorny, P. Chhour, E. Blankemeyer, D. M. Yates, W. R. Witschey, H. I. Litt, A. Tsourkas and D. P. Cormode, J. Mater. Chem. B, 2014, 2, 8239–8248 RSC.
- R. D. Oude Engberink, S. M. A. van der Pol, E. A. Döpp, H. E. de Vries and E. L. A. Blezer, Radiology, 2007, 243, 467–474 CrossRef PubMed.
- Z. Zhang, E. J. van de Bosv, P. Wielopolski, M. de Jong-Popijus, M. Bernsen, D. Duncker and G. Krestin, MAGMA, 2005, 18, 175–185 CrossRef CAS PubMed.
- D. L. J. Thorek and A. Tsourkas, Biomaterials, 2008, 29, 3583–3590 CrossRef CAS PubMed.
- K. Tsuchiya, N. Nitta, A. Sonoda, H. Otani, M. Takahashi, K. Murata, M. Shiomi, Y. Tabata and S. Nohara, Eur. J. Radiol., 2013, 82, 1919–1925 CrossRef PubMed.
- Y. M. Kyong, P. I. Young, I. Y. Kim, I. K. Park, K. J. S. Kwon, H. J. Jeong, Y. Y. Jeong and C. C. Su, J. Nanosci. Nanotechnol., 2008, 8, 5196–5202 CrossRef.
- S. Wagner, J. Schnorr, A. Ludwig, V. Stangl, M. Ebert, B. Hamm and M. Taupitz, Int. J. Nanomed., 2013, 767–779 Search PubMed.
- D. G. You, G. Saravanakumar, S. Son, H. S. Han, R. Heo, K. Kim, I. C. Kwon, J. Y. Lee and J. H. Park, Carbohydr. Polym., 2014, 101, 1225–1233 CrossRef CAS PubMed.
- Z. M. Dong, S. M. Chapman, A. A. Brown, P. S. Frenette, R. O. Hynes and D. D. Wagner, J. Clin. Invest., 1998, 102, 145–152 CrossRef CAS PubMed.
- K. Wenzel, F. X. Felix, S. Kleber, R. Brachold, T. Menke, S. Schattke, K. L. Schulte, C. Glaser, K. Rohde, G. Baumann and A. Speer, Hum. Mol. Genet., 1994, 3, 1935–1937 CrossRef CAS PubMed.
- P. C. Burger and D. D. Wagner, Blood, 2002, 101, 2661–2666 CrossRef PubMed.
- J. G. Park and G.-T. Oh, BMB Rep., 2011, 44, 497–505 CrossRef CAS PubMed.
- A. M. Morawski, G. A. Lanza and S. A. Wickline, Curr. Opin. Biotechnol., 2005, 16, 89–92 CrossRef CAS PubMed.
- F. Chaubet, I. Bertholon, J. M. Serfaty, R. Bazeli, H. Alsaid, M. Jandrot-Perrus, C. Zahir, P. Even, L. Bachelet, Z. Touat, E. Lancelot, C. Corot, E. Canet-Soulas and D. Letourneur, Contrast Media Mol. Imaging, 2007, 2, 178–188 CrossRef CAS PubMed.
- A. Hasan, G. D. Souza, B. M. Calude, C. Frederic, S. Abdulrazzaq, D.-M. Catherine, C. Linda, Z. Charaf, L. Eric, R. Olivier, C. Claire, P. Douek, A. Briguet, L. Didier and C. S. Emmanuelle, Invest. Radiol., 2009, 44, 151–158 CrossRef PubMed.
- L. Chaabane, N. Pellet, M. C. Bourdillon, C. D. Mansard, A. Sulaiman, G. Hadour, F. Thivolet-Béjui, P. Roy, A. Briguet, P. Douek and E. C. Soulas, MAGMA, 2004, 17, 188–195 CrossRef CAS PubMed.
-
E. A. Waters and T. J. Meade, Magnetic Resonance Angiography: Principles and Applications, Springer, New York, 2012, pp. 199–210 Search PubMed.
- S. Laurent, L. Vander Elst, Y. Fu and R. N. Muller, Bioconjugate Chem., 2004, 15, 99–103 CrossRef CAS PubMed.
- S. Boutry, C. Burtea, S. Laurent, G. Toubeau, L. Vander Elst and R. N. Muller, Magn. Reson. Med., 2005, 53, 800–807 CrossRef CAS PubMed.
- F. Hyafil, E. Vucic, J. C. Cornily, R. Sharma, V. Amirbekian, F. Blackwell, E. Lancelot, C. Corot, V. Fuster, Z. S. Galis, L. J. Feldman and Z. A. Fayad, Eur. Heart J., 2010, 32, 1561–1571 CrossRef PubMed.
- M. R. Makowski, S. C. Forbes, U. Blume, A. Warley, C. H. P. Jansen, A. Schuster, A. J. Wiethoff and R. M. Botnar, Atherosclerosis, 2012, 222, 43–49 CrossRef CAS PubMed.
- M. E. Andia, P. Saha, J. Jenkins, B. Modarai, A. J. Wiethoff, A. Phinikaridou, S. P. Grover, A. S. Patel, T. Schaeffter, A. Smith and R. M. Botnar, Arterioscler., Thromb., Vasc. Biol., 2014, 34, 1193–1198 CrossRef CAS PubMed.
- G. S. Loving and P. Caravan, Angew. Chem., Int. Ed., 2014, 53, 1140–1143 CrossRef CAS PubMed.
- M. Sirol, V. Fuster, J. J. Badimon, J. T. Fallon, P. R. Moreno, J.-F. Toussaint and Z. A. Fayad, Circulation, 2005, 112, 1594–1600 CrossRef PubMed.
- M. Sirol, J. G. S. Aguinaldo, P. B. Graham, R. Weisskoff, R. Lauffer, G. Mizsei, I. Chereshnev, J. T. Fallon, E. Reis, V. Fuster, J.-F. Toussaint and Z. A. Fayad, Atherosclerosis, 2005, 182, 79–85 CrossRef CAS PubMed.
- H. Okamura, L. J. Pisani, A. R. Dalal, F. Emrich, B. A. Dake, M. Arakawa, D. C. Onthank, R. R. Cesati, S. P. Robinson, M. Milanesi, G. Kotek, H. Smit, A. J. Connolly, H. Adachi, M. V. McConnell and M. P. Fischbein, Circ. Cardiovasc. Imaging, 2014, 7, 690–696 CrossRef PubMed.
- M. R. Makowski, A. J. Wiethoff, U. Blume, F. Cuello, A. Warley, C. H. P. Jansen, E. Nagel, R. Razavi, D. C. Onthank, R. R. Cesati, M. S. Marber, T. Schaeffter, A. Smith, S. P. Robinson and R. M. Botnar, Nat. Med., 2011, 17, 383–388 CrossRef CAS PubMed.
- D. Li, A. R. Patel, A. L. Klibanov, C. M. Kramer, M. Ruiz, B. Y. Kang, J. L. Mehta, G. A. Beller, D. K. Glover and C. H. Meyer, Circ. Cardiovasc. Imaging, 2010, 3, 464–472 CrossRef CAS PubMed.
- B. den Adel, L. M. van der Graaf, I. Que, G. J. Strijkers, C. W. Löwik, R. E. Poelmann and L. van der Weerd, Contrast Media Mol. Imaging, 2013, 8, 63–71 CrossRef CAS PubMed.
- C. Burtea, S. Ballet, S. Laurent, O. Rousseaux, A. Dencausse, W. Gonzalez, M. Port, C. Corot, L. Vander Elst and R. N. Muller, Arterioscler., Thromb., Vasc. Biol., 2012, 32, e36–e38 CrossRef CAS PubMed.
- X. Wu, N. Balu, W. Li, Y. Chen, X. Shi, C. M. Kummitha, X. Yu, C. Yuan and Z. R. Lu, Am. J. Nucl. Med. Mol. Imaging, 2013, 3, 446–455 Search PubMed.
- C. Burtea, S. Laurent, O. Murariu, D. Rattat, G. Toubeau, A. Verbruggen, D. Vansthertem, L. Vander Elst and R. N. Muller, Cardiovasc. Res., 2008, 78, 148–157 CrossRef CAS PubMed.
- A. Dellinger, J. Olson, K. Link, S. Vance, M. G. Sandros, J. Yang, Z. Zhou and C. L. Kepley, J. Cardiovasc. Magn. Reson., 2013, 15, 7 CrossRef PubMed.
- Z. Zhou, R. P. Lenk, A. Dellinger, S. R. Wilson, R. Sadler and C. L. Kepley, Bioconjugate Chem., 2010, 21, 1656–1661 CrossRef CAS PubMed.
- L. Wang, X. Zhu, X. Tang, C. Wu, Z. Zhou, C. Sun, S.-L. Deng, H. Ai and J. Gao, Chem. Commun., 2015, 51, 4390–4393 RSC.
- J. Querol, W. Chen, R. Weissleder and A. Bogdanov, Org. Lett., 2005, 7, 1719–1722 CrossRef PubMed.
- R. Golestani, J. J. Jung and M. M. Sadeghi, J. Clin. Med., 2016, 5 DOI:10.3390/jcm5060057.
- E. Lancelot, V. Amirbekian, I. Brigger, J. S. Raynaud, S. Ballet, C. David, O. Rousseaux, S. Le Greneur, M. Port, H. R. Lijnen, P. Bruneval, J. B. Michel, T. Ouimet, B. Roques, A. Hyafil, E. Vucic, J. C. S. Aguinaldo, C. Corot and Z. A. Fayad, Arterioscler., Thromb., Vasc. Biol., 2008, 28, 425–432 CrossRef CAS PubMed.
- M. Nahrendorf, F. A. Jaffer, K. A. Kelly, D. E. Sosnovik, E. Aikawa, P. Libby and R. Weissleder, Circulation, 2006, 114, 1504–1511 CrossRef CAS PubMed.
- A. Tsourkas, V. R. Shinde-Patil, K. A. Kelly, P. Patel, A. Wolley, J. R. Allport and R. Weissleder, Bioconjugate Chem., 2005, 16, 576–581 CrossRef CAS PubMed.
- M. Michalska, L. Machtoub, H. D. Manthey, E. Bauer, V. Herold, G. Krohne, G. Lykowsky, M. Hildenbrand, T. Kampf, P. Jakob, A. Zernecke and W. R. Bauer, Arterioscler., Thromb., Vasc. Biol., 2012, 32, 2350–2357 CrossRef CAS PubMed.
- G. A. F. van Tilborg, E. Vucic, G. J. Strijkers, D. P. Cormode, V. Mani, T. Skajaa, C. P. M. Reutelingsperger, Z. A. Fayad, W. J. M. Mulder and K. Nicolay, Bioconjugate Chem., 2010, 21, 1794–1803 CrossRef CAS PubMed.
- S. Boutry, S. Laurent, L. V. Elst and R. N. Muller, Contrast Media Mol. Imaging, 2006, 1, 15–22 CrossRef CAS PubMed.
- K. A. Radermacher, N. Beghein, S. Boutry, S. Laurent, L. Vander Elst, R. N. Muller, B. F. Jordan and B. Gallez, Invest. Radiol., 2009, 44, 398–404 CrossRef CAS PubMed.
- M. H. El-Dakdouki, K. El-Boubbou, M. Kamat, R. Huang, G. S. Abela, M. Kiupel, D. C. Zhu and X. Huang, Pharm. Res., 2013, 31, 1426–1437 CrossRef PubMed.
- P. M. Winter, A. M. Neubauer, S. D. Caruthers, T. D. Harris, J. D. Robertson, T. A. Williams, A. H. Schmieder, G. Hu, J. S. Allen, E. K. Lacy, H. Zhang, S. A. Wickline and G. M. Lanza, Arterioscler., Thromb., Vasc. Biol., 2006, 26, 2103–2109 CrossRef CAS PubMed.
- P. M. Winter, A. M. Morawski, S. D. Caruthers, R. W. Fuhrhop, H. Zhang, T. A. Williams, J. S. Allen, E. K. Lacy, J. D. Robertson, G. M. Lanza and S. A. Wickline, Circulation, 2003, 108, 2270–2274 CrossRef CAS PubMed.
|
This journal is © The Royal Society of Chemistry 2017 |
Click here to see how this site uses Cookies. View our privacy policy here.