Optical transformation of a CdTe quantum dot-based paper sensor for a visual fluorescence immunoassay induced by dissolved silver ions
Received
23rd November 2016
, Accepted 28th December 2016
First published on 29th December 2016
Abstract
This work designs a simple low-cost visual fluorescence immunoassay for disease-related biomarkers (carcinoembryonic antigen, CEA, used as a model protein) in biological fluids, based on the structural and optical transformation of CdTe quantum dots (QDs) immobilized on paper induced by dissolved silver ions (Ag+) from silver nanoparticles (AgNPs) via a cation-exchange reaction. A sandwich-type immunoreaction was initially carried out in a removable polystyrene high-binding microplate coated with monoclonal anti-CEA capture antibody by using AgNP-labeled polyclonal anti-CEA antibody as the detection antibody. Thereafter, the carried AgNPs accompanying the sandwiched immunocomplexes were dissolved by acid to release numerous silver ions, which induced the ion-exchange reaction with the immobilized CdTe QDs on the paper (attached onto the microplate lid) for the Cd-to-Ag transformation, thus resulting in the quenching of the visual fluorescence from the CdTe QDs owing to Ag2Te formation. Under optimal conditions, the fluorescence intensity decreased with the increasing CEA concentration from 0.02 to 50 ng mL−1 with a detection limit of 5.6 pg mL−1. Further, a visual assay based on a CdTe QD-based paper sensor was developed for CEA detection, and 5.0 pg mL−1 CEA could be discriminated with the naked eye. In addition, our strategy displayed high specificity, good reproducibility and acceptable accuracy for analyzing human serum specimens with consistent results obtained using the commercialized enzyme-linked immunosorbent assay (ELISA) method.
Introduction
Molecular diagnostics, one of the most critical steps in healthcare and medical treatment, plays an important role in discovering and delivering insights and innovations that help to improve human health, since it is currently one of the predominant methods to diagnose the onset or progression of disease states.1,2 An advantageous strategy is the desire to realize the goals of personalized medicine and point-of-care (POC) testing, coupling with new concepts and applications of low-cost, portable, and field-based diagnostic technologies.3–5 Despite the high sensitivity of traditional immunoassays such as radioimmunoassays and enzyme-linked immunosorbent assays (ELISAs), the quantitation is usually performed in a centralized laboratory by technicians with a relatively long analysis time and complex operation for instrumentation.6–8 To keep pace with expectations in future POC testing, the next-generation diagnostic platforms should focus on the field of developing assays capable of providing rapidness, simplification and low cost while preserving essential benefits of sensitivity and efficient multiplexing performance for biomarker detection.9,10
Recently, paper-based sensing devices have drawn increasing attention in analytical and clinical chemistry because of their portability, high abundance and low cost.11,12 And more sophisticated analytical devices for paper-based assays, e.g., luminescence,13 electrochemiluminescence,14 visual fluorescence,15 electrochemistry16 and visual detection,17 have been studied on the basis of various signal-generation principles. One major advantage of using paper lies in its simplicity, rapidness, disposability, and visual detection with the naked eye.18,19 The assay is usually carried out on the basis of an enzymatic or a chemical reaction.20,21 Despite some reports on paper-based detection schemes, the development of nanomaterials for commercial use is rather limited besides gold nanoparticles.22–24 Semiconductor nanocrystals (known as quantum dots, QDs, that are several nanometers in size) exhibit unique properties, e.g., broad absorption spectra, narrow, symmetrical and size-tunable emission spectra as well as long photoluminescence lifetimes, and greater photostability and brightness than organic fluorophores, that are intermediate between those of bulk semiconductors and those of discrete molecules, which gives them fascinating applications in optics, bioimagings, labeling, solar cells, LEDs, diode lasers and second-harmonic generation.25–27 Meanwhile, the optoelectronic properties change as a function of both size and shape. These advantages make QDs an attractive fluorophore for the development of paper-based analytical devices.28–31 Significantly, the emergence of ion-exchange reactions with quantum dots (e.g., CdTe QDs) has opened another horizon for the synthesis of new ionic nanocrystals.32–34 Our group recently utilized a common synthesis route to Ag2Te nanocrystals via a simple Cd-to-Ag cation exchange reaction from CdTe QDs at room temperature (orders of magnitude faster than in the bulk).35 Unfavorably, fabrication of a CdTe QD-modified photosensitive electrode was relatively complex. Moreover, the electrochemical workstation and photoelectric conversion device were also necessary for the development of the ion-exchange reaction-based photoelectrochemical immunoassay. To overcome this shortcoming, our motivation in this work is to design a low-cost paper-based visual fluorescence sensor for the detection of biomarkers by coupling with a Cd-to-Ag ion-exchange reaction from immobilized CdTe QDs on paper.
Carcinoembryonic antigen (CEA, a substance involved in cell adhesion) includes a set of highly related glycoproteins produced by cells of the gastrointestinal tract during embryonic development.36 The normal range for CEA in an adult non-smoker is <2.5 ng mL−1 and for a smoker is <5.0 ng mL−1. However, the serum levels are increased in some types of cancer, suggesting that it can be used as a tumor marker in a clinical test. An increasing CEA level indicates progression or recurrence of the cancer. Levels (>20 ng mL−1) before therapy may be associated with a cancer which has already spread (metastatic disease).37 Therefore, sensitive and specific detection of the CEA level in biological fluids would be very helpful for disease diagnostics. Herein, we report on the proof-of-concept of a simple, low-cost and powerful paper-based detection device for the visual fluorescence immunoassay of CEA on the basis of a silver nanolabel-assisted ion-exchange reaction with CdTe QDs immobilized on paper (Scheme 1). Upon addition of target CEA, the analyte is sandwiched between the coated capture antibody on the microplate and the labeled anti-CEA detection antibody on the silver nanoparticles (AgNPs). A subsequent ion-exchange reaction of silver ions released under acidic conditions from the carried AgNPs in the sandwich-type immunoassay format is readily executed with the CdTe QDs on the paper via the following reaction (Ag+ + CdTe → Ag2Te + Cd2+) (please see the reaction mechanism in our recent report35). As Ag2Te is formed, the (visual) fluorescence intensity of the CdTe QD-based paper sensor decreases or weakens with the increasing CEA level. The change in the color and/or fluorescence intensity can be utilized for qualitative or quantitative detection of the target analyte.
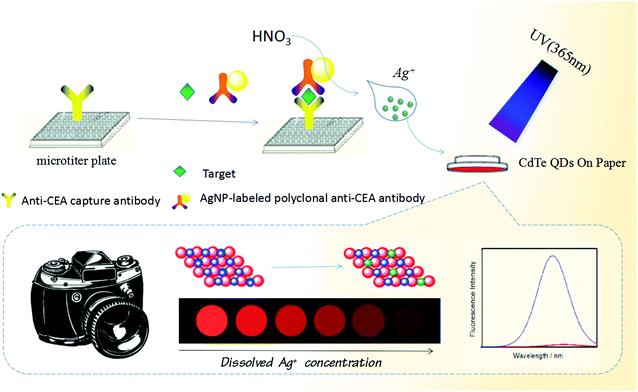 |
| Scheme 1 Schematic illustration of the CdTe quantum dot (QD)-coated paper sensor for visual fluorescence detection of cancer biomarker by using silver nanoparticle (AgNP)-labeled detection antibodies, based on the cation-exchange reaction between CdTe QDs and the dissolved silver ions from AgNPs. | |
Experimental
Materials and chemicals
CEA mouse anti-human monoclonal (unconjugated) (CB30) antibody (mAb1, 25 μg, PBS, pH 7.2, 0.09% sodium azide, RayBiotech Inc., Norcross, GA), rabbit polyclonal anti-human CEA antibody (pAb2, 0.1 mg, GenWay Biotech Inc., San Diego, CA) (note: 0.2 mL of distilled water will yield a concentration of 500 μg mL−1) and CEA standards (Biocell Biotechnol. Inc., Zhengzhou, China) were used in this work. All CEA ELISA kits including 96-well plastic microplates (each microplate consists of twelve removable strips of wells and a frame) were purchased from Biocell Biotechnol. Inc. (Zhengzhou, China). AgNO3, NaBH4, and bovine serum albumin (BSA) were acquired from Sinopharm Chem. Re. Co., Ltd. (Shanghai, China) for the preparation of colloidal silver particles. 3-Mercaptopropionic acid (MPA, Alfa Aesar), sodium tellurite (Na2TeO3, Aladdin, Shanghai, China) and Cd(NO3)2·4H2O (Sinopharm Chem. Re. Co., Ltd, Shanghai, China) were utilized for the synthesis of CdTe QDs. All other reagents were of analytical grade and used without further purification. Ultrapure water obtained from a Millipore water purification system (18.2 MΩ cm−1, Milli-Q) was used in all runs. Human serum specimens were gifted from local Provincial Hospital, Fujian, China.
A pH 9.6 carbonate buffer (1.69 g Na2CO3 and 2.86 g NaHCO3) and pH 7.4 phosphate-buffered saline (PBS, 2.9 g Na2HPO4·12H2O, 0.24 g KH2PO4, 0.2 g KCl and 8.0 g NaCl) were prepared by dissolving them into 1000 mL distilled water. The blocking buffer and washing buffer were obtained by adding 1.0 wt% BSA and 0.05% Tween 20 into pH 7.4 PBS, respectively.
Preparation and labeling of silver nanoparticles with pAb2 antibody
Colloidal silver nanoparticles (AgNPs) ∼15 nm in diameter were prepared by referring to the literature reports with minor modification.38,39 Initially, 10 mL of 1.0 mM AgNO3 aqueous solution was slowly dropped into 30 mL NaBH4 aqueous solution (2.0 mM) under vigorous stirring in an ice bath. Then, the mixture was continuously stirred until the color turned from bright yellow to brownish yellow, and the temperature of the solution rose to room temperature. Finally, the obtained silver colloids were stored at 4 °C in darkness for further use. The absorption spectrum of the resulting brownish yellow solution was characterized by a maximum at ∼15 nm. Also, it was found that the as-prepared silver colloids could be stable for at least 6 months at 4 °C in darkness. Herein, the concentration of AgNPs (C[AgNP]) could be estimated to be ∼1.627 × 10−10 M according to Beer’s law [A = εlc, where the extinction coefficient (ε) is calculated by the equation (ln
ε = 1.4418 × ln
D + 18.955), and D is the diameter in nm].40,41
Next, the as-prepared AgNPs were used for the labeling of anti-CEA pAb2 antibody.42,43 Prior to labeling, colloidal silver nanoparticles were first adjusted to pH 9.0–9.5 by using Na2CO3 aqueous resolution to avoid precipitation upon protein introduction (Importantly!!!). Following that, pAb2 detection antibody (50 μL, 500 μg mL−1) was added into 2.0 mL of the above-prepared silver colloids. The resulting suspension was slightly shaken overnight at 4 °C in darkness on a shaker (MS, IKA GmbH, Staufen, Germany). After that, polyethylene glycol (50 μL, 1.0 wt%) was injected into the suspension in order to enhance the dispersivity. Finally, the AgNP-labeled pAb2 antibody (denoted as pAb2–AgNP) was collected by centrifugation for 15 min at 14
000g, and dispersed into 2.0 mL of pH 7.4 PBS containing 1.0 wt% BSA and 0.1% (v/v) sodium azide for storage at 4 °C when not in use.
Synthesis of CdTe QDs and fabrication of CdTe QD-impregnated paper
The water-soluble CdTe QDs were synthesized similar with our previous report35 as follows. Prior to synthesis, a homogeneous mixture was initially prepared by adding Cd(NO3)2 (118 mg) and trisodium citrate (200 mg) into 50 mL distilled water containing 55 μL of MPA. Then, the resultant mixture was adjusted to pH 10.5 with the assistance of 1.0 M NaOH. After that, Na2TeO3 (22.2 mg) and KBH4 (50 mg) were thrown into the solution in turn. The resulting mixture was refluxed for 60 min at room temperature. Following that, the suspension was precipitated with n-propanol and centrifuged for 10 min at 8000g to remove the impurities. Finally, the water-soluble CdTe QDs were dispersed in 10 mL distilled water for use.
CdTe QD-impregnated paper was fabricated by consulting the literature.44 Initially, the chromatography paper (3 MM CHR, 20 × 20 cm, Cat No. 3030-861, Whatman, U.K.) was cut into many circular paper sheets with 50 mm diameters (note: the size was almost the same as that of the lid of the 96-well polystyrene microplate) by using a Deli hole puncher (No. 0102, Zhejiang, China). Thereafter, the circular paper was immersed into 0.5 mL of the above-prepared CdTe QD suspension for 30 min at room temperature (note: to achieve good reproducibility and precision, each circular piece of paper was incubated with CdTe QDs alone). Finally, the circular paper was taken out and dried in an oven at 40 °C, and then put into the plastic lid for subsequent usage.
The immunoreaction process and visual fluorescence measurements
Scheme 1 gives the schematic illustration of the CdTe QD-based paper sensor for visual fluorescence immunoassay induced by the dissolved silver ions from the AgNP-labeled detection antibody. Prior to the immunoreaction, a capture antibody-coated microplate was prepared by injecting 50 μL carbonate buffer (pH 9.6) containing 10 μg mL−1 anti-CEA mAb1 into a high-binding 96-well single-break strip plate and incubating overnight at 4 °C (note: the microplate was covered with an adhesive plastic plate sealing film in order to prevent evaporation). After washing with the washing buffer (twice), the microplate was blocked with the blocking buffer (60 min at 37 °C) to eliminate the nonspecific adsorption. Next, the sandwiched immunoreaction was carried out by adding different-level target CEA standards (50 μL) and the above-prepared pAb2–AgNP suspension (50 μL) into the well in turn. Each incubation process was executed for 35 min at room temperature under slight shaking on a shaker, with washing after each step. Following that, a 20 μL aliquot of 100 μM HNO3 solution was injected into each well to release silver ions from the captured AgNPs (8 min). Afterward, the microplate was placed upside down accompanying the lid attached with CdTe QD-impregnated paper after dilution to 10-fold with distilled water, and reacted for 60 s (fast) at room temperature. During this process, the ion-exchange reaction was carried out by the released silver ions with the immobilized CdTe QDs on paper via the reaction (Ag+ + CdTe → Ag2Te + Cd2+). Finally, the resulting circular paper was transferred to a UV test box to accomplish the visual qualitative detection under a 365 nm UV light. Also, the fluorescence intensity of the CdTe QD-impregnated paper was quantitatively determined on a Hitachi F-4600 fluorescence spectrofluorometer (Tokyo, Japan) via putting the paper in a solid sensing cell on a solid sample holder. The change in the fluorescence intensity (vs. background signal) was collected and registered as the sensor signal relative to the target CEA concentration by using the Stern–Volmer equation: I0/I = KSV [Q] + C (where I0 and I represent the fluorescence intensities of CdTe QD-modified paper before and after the ion-exchange reaction with the dissolved silver ions, respectively; KSV is the Stern–Volmer quenching constant; and Q is the concentration of target CEA). All measurements were performed at room temperature (25 ± 1.0 °C) unless specified otherwise.
Results and discussion
The design of the CdTe QD-based paper sensor and characterization of pAb2–AgNPs
Methods based on molecular tags, e.g., fluorophores and quantum dots, have been developed for fluorescence immunoassays, but most have low sensitivity and are unsuitable for detection of low-abundance proteins. A possible speculation is that the traditional fluorescent chromophores (e.g., fluorescein isothiocyanate and rhodamine) tend to have poor photostability and brightness, and a short Stokes shift, thus causing significant problems when working with samples with naturally high background fluorescence. Ongoing effort has been recently made worldwide by using QDs due to their superior optical properties and lower susceptibility to photobleaching than the organic fluorophores. Unfavorably, the size of the generally prepared QDs are relatively small (<10 nm), thereby limiting the detectable fluorescence signal. In addition, the labeling of QDs with the biomolecules via the sophisticated surface-modification chemistry may decrease the fluorescence intensity. In this work, quantum dots alone were immobilized on paper without any modification, and the fluorescence signal was quenched by the dissolved silver ion from the nanolabels of the immunoassay accompanying the ion-exchange reaction. The split-type detection system is expected to enhance the sensitivity of the fluorescence immunoassay, whilst the use of the polystyrene microplate and chromatography paper helps to decrease the assay cost. Anti-CEA pAb2 detection antibody is conjugated to AgNPs through the dative binding between nanoparticles and free –SH on the proteins, while CdTe QDs are physically adsorbed on paper. Upon target CEA introduction, the analyte is sandwiched between the coated mAb1 capture antibody on the microplate and pAb2–AgNP. Under acidic conditions, the carried AgNPs accompanying the detection antibody are dissolved and release numerous silver ions. In this case, a large number of CdTe QDs on paper are changed into Ag2Te nanocrystals via the ion-exchange reaction, hence resulting in the quenching of the fluorescence signal. The change in the fluorescence intensity relative to the background signal can be used for quantitative monitoring of target CEA in the sample. Meanwhile, the CdTe QD-based paper can also be qualitatively determined on the basis of the change in the visual fluorescence signal under a 365 nm UV light.
To realize our design, we initially used high-resolution transmission electron microscopy (HRTEM, H-7650, Hitachi, Japan) to characterize the as-synthesized AgNPs. As shown in Fig. 1A, silver nanoparticles were homogeneously dispersed in the solution, and the average size was about 15 nm. Clearly, it can be attributed to the (111) lattice of AgNPs. The successful synthesis of silver nanoparticles provided a precondition for the labeling of the pAb2 antibody. To verify this issue, we employed UV-vis absorption spectroscopy (NanoQuant, Infinite 200 Pro, Tecan Austria GmbH, Austria) to investigate colloidal silver nanoparticles before and after incubation with pAb2 antibody. As seen from Fig. 1B, the spectrum of the newly prepared silver colloids exhibited a characteristic plasmon absorption peak at 402 nm (curve ‘a’). After the reaction of AgNPs with pAb2 antibody, the plasmon absorption peak shifted from 402 to 410 nm (curve ‘b’), indicating the formation of bioconjugates.45,46 Vaguely, we could observe the characteristic of pAb2 antibody (∼280 nm) referring to our previous work.47 Furthermore, dynamic light scattering (DLS, Zetasizer Nano S90, Malvern, London, U.K.) was utilized to monitor the size of AgNPs before and after modification with pAb2 antibody. As indicated in Fig. 1C, the size of the nanostructures increased from 15 ± 1.1 nm to 23 ± 1.7 nm after conjugation with pAb2 antibody. The increase in the size should be derived from the labeled antibody. These results revealed that pAb2–AgNPs could be successfully synthesized by our design.
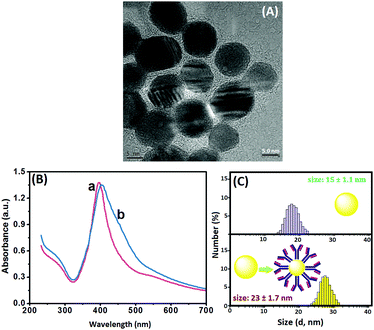 |
| Fig. 1 (A) HRTEM image of AgNPs, (B) UV-vis absorption spectra of (a) AgNPs and (b) pAb2–AgNPs, and (C) DLS data of AgNPs and pAb2–AgNPs. | |
Identification of the cation exchange reaction in CdTe QDs relative to the silver ion
As described above, the visual fluorescence intensity originates from the ion-exchange reaction in CdTe QDs with the dissolved silver ions from the labeled AgNPs on the detection antibody under acidic conditions. Prior to the identification of the ion-exchange reaction, we used HRTEM to monitor the as-synthesized CdTe QDs. As shown in Fig. 2A, the size of the water-soluble nanocrystals was ∼5.0 nm. Maybe, one puzzling question arises as to whether the nanocrystals could readily carry out the cation exchange reaction with a silver ion. To understand this issue intuitively, 100 μL of AgNO3 aqueous solution (0.1 mM) was directly injected into a CdTe QD solution at room temperature under ambient conditions. Immediately, the color of the CdTe QDs changed from light reddish-brown to black (Fig. 2B, top), and the fluorescence was also quenched under a 365 nm UV light (Fig. 2B, bottom). Readily, the cation exchange reaction was very fast, which was in accordance with previous reports.44 The reason might be attributed to a low activation barrier, large surface area and small volume.48–50 To further elucidate the ion-exchange reaction, we also used density functional theory (DFT) to calculate the binding affinity between the CdTe QDs and Ag+ ions by using Cd33Te33 nanoclusters as a model, and HRTEM, X-ray diffraction (XRD), X-ray photoelectron spectroscopy (XPS), UV-vis absorption spectroscopy and fluorescence spectroscopy to investigate CdTe QDs before and after the reaction with Ag+ ions [please see the detailed results and discussion in our recent work]35 (note: similar figures and description were not placed in this study to avoid overlapping). Despite these studies proving that the ion-exchange reaction in CdTe QDs with Ag+ ions could occur completely at room temperature with a fast reaction rate, we used another characterization method (i.e., an anodic stripping voltammetric method) to further verify the above-mentioned conclusion. The assay was carried out in 2.0 mL acetate buffer (0.2 M, pH 5.6) containing CdTe QDs in the absence and presence of Ag+ ions on a CHI 620D Electrochemical Workstation (Chenhua, Shanghai, China) with a conventional three-electrode system comprising a platinum wire counter electrode, a saturated calomel electrode (SCE) reference electrode and an in situ formed mercury drop electrode (Fig. 2C). As seen from curve ‘a’, a relatively weak voltammetric peak was observed in the absence of silver ions. Upon addition of Ag+ ions, however, the characteristic peak current for Cd2+ ions largely increased (curve ‘b’), indicating that some of the Cd2+ ions in the CdTe QDs were released out on the basis of the reaction: Ag+ + CdTe → Ag2Te + Cd2+. Referring to our previous reports and the additional studies, we may make a definite conclusion that the ion-exchange reaction in CdTe QDs could be smoothly executed by the dissolved Ag+ ions.
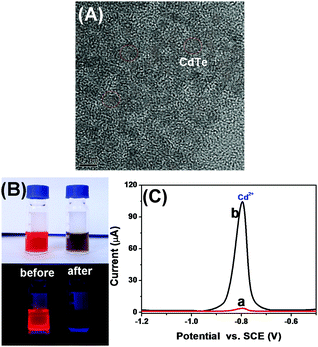 |
| Fig. 2 (A) HRTEM image of CdTe QDs, (B) photographs of CdTe QDs before and after reaction with Ag+ ions under (top) natural light and (bottom) 365 nm UV light, respectively, and (C) anodic stripping voltamogramms of the in situ formed mercury drop electrode in 2.0 mL acetate buffer (0.2 M, pH 5.6) containing CdTe QDs in the (a) absence and (b) presence of Ag+ ions. | |
Optimization of experimental conditions
In this work, silver ions were released from AgNPs upon addition of HNO3, and the ion-exchange reaction was implemented with CdTe QDs, thus resulting in the transformation between CdTe and Ag2Te. So, the concentration of the added HNO3 would greatly affect the visual fluorescence signal. To investigate this issue, different concentrations of HNO3 were incubated with the CdTe QD-modified paper. As shown in Fig. 3A, the fluorescence intensity increased with a decreasing HNO3 amount, and the signal when using ≤10 μM HNO3 was almost the same as that of the background signal. The reason might be the fact that some of the CdTe QDs were dissolved at a high concentration of HNO3. Thus, 100 μM HNO3 was diluted (at least) 10-fold with distilled water during the ion-exchange reaction.
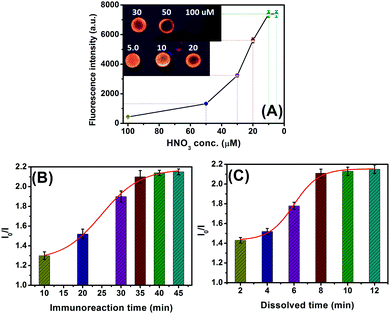 |
| Fig. 3 The effects of (A) HNO3 concentration for the ion-exchange reaction (Inset: The corresponding fluorescence images), (B) immunoreaction time for the antigen–antibody reaction, and (C) the dissolution time of AgNPs under 100 μM HNO3 on the fluorescence of the CdTe QD-based visual fluorescence immunoassay (1.0 ng mL−1 CEA was used in this case). | |
As mentioned above, the released silver ions are derived from the labeled AgNPs on the detection antibody accompanying the sandwiched immunoreaction. Generally speaking, it takes some time to implement the antigen–antibody reaction. A short incubation time is unfavorable for the formation of the sandwiched immunocomplexes. As seen from Fig. 3B, the change in the fluorescence (I0/I) increased with the increment of target CEA (1.0 ng mL−1 used as an example), and tended to level off after 35 min (note: the reaction time of mAb1-CEA was the same as that of the pAb2–AgNP to avoid confusion). To save assay time, 35 min was used for the antigen–antibody reaction in this work.
Since the ion-exchange reaction between CdTe QDs and Ag+ ions was very fast, the fluorescence was recorded as the exchange reaction proceeded after 60 s to avoid possible errors resulting from different additions of samples. Under these conditions, we monitored the effect of the dissolution time of AgNPs under acidic conditions on the fluorescence intensity of the CdTe QD-based paper relative to 1.0 ng mL−1 CEA. As indicated in Fig. 3C, the fluorescence (I0/I) increased with longer dissolution time, and reached an equilibrium state after 8 min. A longer dissolution time did not cause a significant change in the signal. Therefore, 8 min was selected for the releasing of silver ions from AgNPs upon addition of 10 μM HNO3.
Dose–response curve of CdTe QD-based visual fluorescence immunoassay toward target CEA
Under the optimal experimental conditions, a CdTe QD-based visual fluorescence immunoassay was used for the qualitative/quantitative detection of target CEA based on the change in the visible color and the shift in the fluorescence intensity of the CdTe QD-modified paper after the antigen–antibody reaction. Firstly, we evaluated the analytical performance of the CdTe QD-based visual immunoassay under 365 nm UV irradiation after pAb2–AgNPs had reacted with various concentrations of CEA standards on mAb1-coated microplates. As shown in Fig. 4A, the fluorescence of the CdTe QD-coated paper was gradually quenched with the increasing target CEA level, even at a low concentration of 5.0 pg mL−1 relative to the background signal. Moreover, the quantitative analysis of the CdTe QD-based fluorescence immunoassay could be acquired on a commercial fluorospectrometer by using the above-mentioned Stern–Volmer equation (Fig. 4B). The corresponding fluorescence increased with the increasing target CEA, and a good linear correlation between I0/I and CEA concentration was obtained within the dynamic range from 0.02 ng mL−1 to 50 ng mL−1 with a correlation coefficient (r) of 0.9975 (n = 8). The linear regression equation could be fitted to I0/I = 0.3689C + 1.4222 (ng mL−1) (Fig. 4B, inset). Each datum represents the average value obtained from three different measurements, and the maximum relative standard deviation (RSD) was 8.9%, indicating a good reproducibility. The limit of detection, defined as the CEA concentration equivalent to 3s (standard deviation) of 11 repeated measurements of a blank solution, was calculated to be 5.6 pg mL−1, which was obviously lower than those of commercially available human CEA ELISA kits from different companies e.g., BioVision, Inc. (LOD: 0.2 ng mL−1, Cat# K4805-100), Diagnostic Automation, Inc. (LOD: 1.0 ng mL−1, Cat# 5201-16), Boster Biolog. Technol. (LOD: <10 pg mL−1, Cat# EK0904), RayBiotech, Inc. (LOD: 0.2 ng mL−1, Cat# P06731), and Sigma-Aldrich (LOD: 0.05 ng mL−1, Cat# RAB0411). Since the threshold value is 2.5 ng mL−1 CEA for adult non-smokers and 5.0 ng mL−1 CEA for smokers, our strategy could meet the needs of CEA detection.
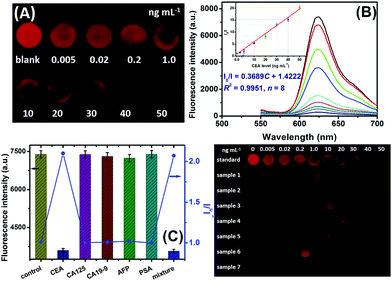 |
| Fig. 4 (A) Fluorescence images of the CdTe QD-based visual immunoassay relative to different concentrations of CEA standards under 365 nm UV-lamp excitation using a digital camera, (B) the corresponding photoluminescence emission spectra by using a commercial fluorospectrometer (inset: the corresponding calibration curve), (C) the specificity of this system against target CEA, CA 125, CA 19-9, AFP and PSA (note: the mixture contained the above-mentioned analytes), and (D) fluorescence images of our strategy toward seven human serum samples. | |
Specificity and stability of CdTe QD-based immunoassay
The specificity of the CdTe QD-based fluorescence immunoassay were evaluated by challenging our system against other proteins or biomarkers, e.g., cancer antigen 125 (CA 125), cancer antigen CA 19-9 (CA 19-9), alpha-fetoprotein (AFP), and prostate-specific antigen (PSA). The evaluation was carried out on the basis of a low concentration of target CEA (1.0 ng mL−1) and a high level of the non-target analyte (100 ng mL−1 or 100 U mL−1). As shown in Fig. 4C, the fluorescence intensities toward the non-target analytes alone were almost the same as that of the background signal, while a change in the fluorescence could be observed toward target CEA. More importantly, the co-existence of non-target analytes with target CEA did not interfere with the detectable signal of CdTe QD-based paper relative to the analyte alone. These results indicated that our strategy had a good selectivity toward target CEA.
The stability of pAb2–AgNPs and CdTe QDs was also studied during a 60-day period. When not in use, they were stored at 4 °C in darkness, and measured intermittently (every 3–5 days) toward the same concentration of CEA standard. Almost no apparent change in the fluorescence was found after storage for 20 days toward 1.0 ng mL−1 CEA, but a ∼6.5% decrease in their initial signal was noticed after 60 days. A possible reason might be attributed to the gradual denaturation of the labelled antibodies on the AgNPs and the variability of CdTe QDs during the storage. Hence, the stability of the CdTe QD-based visual fluorescence immunoassay is acceptable.
Preliminary applications in real samples
To further elucidate the accuracy of our method, seven human serum specimens gifted from local Fujian Provincial Hospital of China were detected by using CdTe QD-based visual fluorescence immunoassay. Prior to measurements, these samples were gently shaken at room temperature (note: all handling and processing were performed carefully, and all tools in contact with patient specimens and immunoreagents were disinfected after use), and then evaluated by our strategy. Fig. 4D gives the visual results under a 365 nm UV light. Further, these samples were evaluated by using a commercial human CEA ELISA kit (LOD: 0.235 ng mL−1, Cat#: ab 183365, Abcam) and our system, respectively (Table 1). Statistical comparison between two methods was performed by using a t-test relative to these data. Almost no significant differences were encountered between the two methods at the 0.05 significance level because all of the texp values were less than tcrit (tcrit[4,0.05] = 2.77). Further, we also investigated the recovery experiments of our system by spiking CEA standards (including 0.5, 5.0 and 20 ng mL−1) in blank new-born cattle serum (Dingguo Biotechnol. Co. Ltd, Beijing, China). The content measured by using CdTe QD-based fluorescence immunoassay was 0.57, 4.68 and 24.3 ng mL−1 for the above-mentioned analytes, respectively. The recoveries were 114%, 93.6% and 121.5%, respectively. So, our system could be considered as an optional scheme for the detection of CEA in clinical diagnostics.
Table 1 Comparison of the assayed results for seven human serum samples with an appropriate dilution ratio obtained by the CdTe QD-based fluorescence immunoassay and a commercial human CEA ELISA kit
Sample no. |
Method; concentration (mean ± SD, ng mL−1, n = 3) |
t
exp
|
Fluorescence immunoassay |
CEA ELISA kit |
1 |
2.3 ± 0.3 |
2.1 ± 0.4 |
0.69 |
2 |
43 ± 1.6 |
46 ± 1.1 |
2.68 |
3 |
13 ± 0.9 |
14 ± 1.3 |
1.09 |
4 |
27 ± 3.2 |
25 ± 2.7 |
0.83 |
5 |
18 ± 2.1 |
16 ± 2.6 |
1.04 |
6 |
0.92 ± 0.2 |
0.89 ± 0.1 |
0.24 |
7 |
49 ± 1.9 |
52 ± 1.6 |
2.09 |
Conclusions
In summary, we demonstrated the development of an advanced visual fluorescence immunoassay by coupling a low-cost polystyrene microplate and highly efficient CdTe QD-modified paper. Relative to conventional enzyme or fluorophore-labeled immunoassays, the use of AgNPs can be used to enhance the detectable signal because numerous silver ions are released from individual nanoparticles under acidic conditions. The dissolved silver ions can quench the fluorescence of the immobilized CdTe QDs on the paper. Meanwhile, a large number of CdTe QDs are readily attached onto the paper without the need for any cross-linkage agents. The target analyte is detected qualitatively or quantitatively by using 365 nm UV light irradiation and a commercial fluorospectrometer. Significantly, our system can be further extended for the detection of other biomarkers by changing the corresponding antibodies, thereby representing a versatile detection mode. Nevertheless, one disadvantage in this study is the need to control the pH value of the detection solution since it would greatly affect the fluorescence of the immobilized CdTe QDs on the paper. Further studies should focus on improvement of the detection system.
Acknowledgements
This work was financially supported by the National Natural Science Foundation of China (Grant no. 21675029 and 21475025), the National Science Foundation of Fujian Province (Grant no. 2014J07001), and the Program for Changjiang Scholars and Innovative Research Team in University (Grant no. IRT15R11).
References
- J. Hu, T. Wang, J. Kim, C. Shannon and C. Easley, J. Am. Chem. Soc., 2012, 134, 7066–7072 CrossRef CAS PubMed.
- M. Haider, B. Ji, T. Haselgrubler, A. Sonnleitner, F. Aberger and J. Hesse, Biosens. Bioelectron., 2016, 86, 20–26 CrossRef CAS PubMed.
- J. Vermeesch, T. Voet and K. Devriendt, Nat. Rev. Genet., 2016, 17, 643–656 CrossRef CAS PubMed.
- S. Shiao, G. Chu and L. Chung, Cancer Lett., 2016, 380, 340–348 CrossRef CAS PubMed.
- K. Levrie, K. Jans, R. Vos, N. Ardakanian, N. Verellen, C. Van Hoof, L. Lagae and T. Stakenborg, Bioelectrochemistry, 2016, 112, 61–66 CrossRef CAS PubMed.
- G. Catananta, A. Rhouati, A. Hayat and J. Marty, Electroanalysis, 2016, 28, 1750–1763 CrossRef.
- R. Liu, S. Zhang, C. Wei, Z. Xing, S. Zhang and X. Zhang, Acc. Chem. Res., 2016, 49, 775–783 CrossRef CAS PubMed.
- L. Chang, J. Li and L. Wang, Anal. Chim. Acta, 2016, 910, 12–24 CrossRef CAS PubMed.
- J. Hu, X. Cui, Y. Gong, X. Xu, B. Gao, T. Wen, T. Lu and F. Xu, Biotechnol. Adv., 2016, 24, 305–320 CrossRef PubMed.
- T. Lan, J. Zhang and Y. Lu, Biotechnol. Adv., 2016, 34, 331–341 CrossRef CAS PubMed.
- J. Cunnigham, P. Degregory and R. Crooks, Annu. Rev. Anal. Chem., 2016, 9, 183–202 CrossRef PubMed.
- Q. Ju, M. Noor and U. Krull, Analyst, 2016, 141, 2838–2860 RSC.
- M. He, Z. Li, Y. Ge and Z. Liu, Anal. Chem., 2016, 88, 1530–1534 CrossRef CAS PubMed.
- L. Wu, Y. Zhang, Y. Wang, S. Ge, H. Liu, M. Yan and J. Yu, Microchim. Acta, 2016, 183, 1873–1880 CrossRef CAS.
- K. Huang, K. Xu, W. Zhu, L. Yang, X. Hou and C. Zheng, Anal. Chem., 2016, 88, 789–795 CrossRef PubMed.
- H. Li, W. Wang, Q. Lv, G. Xu, H. Bai and Q. Zhang, Electrochem. Commun., 2016, 68, 104–107 CrossRef CAS.
- S. Rosolina, T. Carpenter and Z. Xue, Anal. Chem., 2016, 88, 1553–1558 CrossRef CAS PubMed.
- S. Burrs, M. Bhargava, R. Sidhu, J. Kiernan-Lewis, C. Gomes, J. Claussen and E. McLamore, Biosens. Bioelectron., 2016, 85, 479–487 CrossRef CAS PubMed.
- R. Pelton, TrAC, Trends Anal. Chem., 2009, 28, 925–942 CrossRef CAS.
- D. Liana, B. Raguse and J. Gooding, Sensors, 2012, 12, 11505–11526 CrossRef CAS PubMed.
- E. Nery and L. Kubota, Anal. Bioanal. Chem., 2013, 405, 7573–7595 CrossRef CAS PubMed.
- M. Santhiago, E. Nery, G. Santos and L. Kubota, Bioanalysis, 2014, 6, 89–106 CrossRef CAS PubMed.
- J. Hu, S. Wang, L. Wang, F. Li, B. Pingguan-Murphy, T. Lu and F. Xu, Biosens. Bioelectron., 2014, 54, 585–597 CrossRef CAS PubMed.
- X. Ge, A. Asiri, D. Du, W. Wen, S. Wang and Y. Lin, TrAC, Trends Anal. Chem., 2014, 58, 31–39 CrossRef CAS.
- D. Gomez, K. Vernon, P. Mulvaney and T. Davis, Nano Lett., 2010, 10, 274–278 CrossRef CAS PubMed.
- Z. Chen, S. Berciaud, C. Nuckolls, T. Heinz and L. Brus, ACS Nano, 2010, 4, 2964–2968 CrossRef CAS PubMed.
- W. Liu, K. Whitaker, K. Kittilstved and D. Gamelin, J. Am. Chem. Soc., 2006, 128, 3910–3911 CrossRef CAS PubMed.
- L. Page, X. Zhang, A. Jawaid and P. Snee, Chem. Commun., 2011, 47, 7773–7775 RSC.
- G. Zou and H. Ju, Anal. Chem., 2004, 76, 6871–6876 CrossRef CAS PubMed.
- W. Algar, K. Susumu, J. Delehanty and I. Medintz, Anal. Chem., 2011, 83, 8826–8837 CrossRef CAS PubMed.
- P. Dai, T. Yu, H. Shi, J. Xu and H. Chen, Anal. Chem., 2015, 87, 12372–12379 CrossRef CAS PubMed.
- Y. Yu, X. Yin, A. Kvit and X. Wang, Nano Lett., 2014, 14, 2528–2535 CrossRef CAS PubMed.
- A. Smith, L. Lane and S. Nie, Nat. Commun., 2014, 5, 4506 CAS.
- D. Lee, W. Kim, S. Lee, W. Bae, L. Lee and D. Lee, Chem. Mater., 2015, 27, 5295–5304 CrossRef CAS.
- Y. Lin, Q. Zhou, D. Tang, R. Niessner, H. Yang and D. Knopp, Anal. Chem., 2016, 88, 7858–7866 CrossRef CAS PubMed.
- X. Li, M. Yu, Z. Chen, X. Lin and Q. Wu, Sens. Actuators, B, 2017, 239, 874–882 CrossRef CAS.
- H. Miao, L. Wang, Y. Zhuo, Z. Zhou and X. Yang, Biosens. Bioelectron., 2016, 86, 83–89 CrossRef CAS PubMed.
- C. Munro, W. Smith, M. Garner, J. Clarkson and P. White, Langmuir, 1995, 11, 3712–3720 CrossRef CAS.
- H. Li, Z. Sun, W. Zhong, N. Hao, D. Xu and H. Chen, Anal. Chem., 2010, 82, 5477–5483 CrossRef CAS PubMed.
- J. Yguerabide and E. Yguerabide, Anal. Biochem., 1998, 262, 137–156 CrossRef CAS PubMed.
- R. Kanjanawarut and X. Su, Anal. Chem., 2009, 81, 6122–6129 CrossRef CAS PubMed.
- N. Tung, M. Chikae, Y. Ukita, P. Viet and Y. Takamura, Anal. Chem., 2012, 84, 1210–1213 CrossRef CAS PubMed.
- J. Ling, Y. Li and C. Huang, Anal. Chem., 2009, 81, 1707–1714 CrossRef CAS PubMed.
- K. Huang, K. Xu, J. Tang, L. Yang, X. Hou and C. Zheng, Anal. Chem., 2015, 87, 6587–6591 Search PubMed.
- X. Liu, Q. Dai, L. Austin, J. Coutts, G. Knowles, J. Zou, H. Chen and Q. Huo, J. Am. Chem. Soc., 2008, 130, 2780–2782 CrossRef CAS PubMed.
- J. Zheng, A. Jiao, R. Yang, H. Li, J. Li, M. Shi, C. Ma, Y. Jiang, L. Deng and W. Tan, J. Am. Chem. Soc., 2012, 134, 19957–19960 CrossRef CAS PubMed.
- D. Tang, R. Yuan and Y. Chai, Anal. Chem., 2008, 80, 1582–1588 CrossRef CAS PubMed.
- D. Son, S. Hughes, Y. Yin and A. Alivisatos, Science, 2004, 306, 1009–1012 CrossRef CAS PubMed.
- S. Wark, C. Hsia and D. Son, J. Am. Chem. Soc., 2008, 130, 9550–9555 CrossRef CAS PubMed.
- J. Luther, H. Zheng, B. Sadtler and A. Alivistos, J. Am. Chem. Soc., 2009, 131, 16851–16857 CrossRef CAS PubMed.
|
This journal is © The Royal Society of Chemistry 2017 |
Click here to see how this site uses Cookies. View our privacy policy here.