A ratiometric nanoprobe consisting of up-conversion nanoparticles functionalized with cobalt oxyhydroxide for detecting and imaging ascorbic acid†
Received
6th October 2016
, Accepted 14th November 2016
First published on 15th November 2016
Abstract
The ability to rapidly, sensitively, and selectively detect ascorbic acid (AA) is important for medical assays and diagnoses. Here, a new cobalt oxyhydroxide (CoOOH)-functionalized up-conversion nanoparticle (UCNP) system was designed for the detection of AA. For the UCNPs, ligand-free β-NaYF4:Gd/Yb/Tm/Ho@NaYF4 offered simultaneous blue and red light emission, but CoOOH on the UCNP surface acted as a quencher that could effectively absorb the 475 nm-wavelength light produced by the luminescence of the nanoparticles. This quenching was found to be easily eliminated by the addition of AA through the FRET process. The ratio of the intensity of the up-conversion luminescence at 475 nm to that at 654 nm showed an excellent linear correlation with the concentration of AA. The nanoprobe also exhibited excellent accuracy in the quantitative analysis of AA in diluted fetal bovine serum. In addition, the probe showed an ability to be used for imaging AA in living cells, and may thus be considered as a good candidate for use in clinical diagnosis and drug screening.
Introduction
Small-molecule reactive species have in recent years attracted widespread attention due to their crucial roles in cell signaling.1–3 Ascorbic acid (AA) is a pivotal antioxidant and neuromodulator in the human body.4–7 Improper levels of AA are associated with diseases. For example, inadequate AA intake leads to the symptoms of scurvy, whereas its excess intake causes the formation of urinary stones, and also results in diarrhea and stomach convulsions.8,9 Thus, it is of great significance in physiology and pathology to detect AA effectively.4,10 So far, a few efficient methods for detecting AA have been developed, and they have involved chromatography,11 capillary electrophoresis,12 electrochemistry,13,14 spectrophotometry,15 and fluorometry.16,17 Fluorescent assays may be the most feasible technique for the detection of AA in living systems, due to the advantages, such as real-time spatial imaging, high sensitivity, and minimal harm to biological samples, they also displayed. Although many organic fluorescent probes have been deployed for detecting small biological molecules,18–20 fluorescent probes for determining the concentration of and imaging AA are still rare. The Yamada group has developed an AA probe by covalently coupling a nitroxide with a fluorophore.22 This probe, whose operation is based on an electron-exchange interaction, has been shown to sensitively and easily detect AA. However, these reported organic fluorescent probes have shown several drawbacks, including a relatively narrow range for which the relationship between the signal and AA concentration is linear, as well as poor photostability, difficult purification, and complex synthesis, which have all limited their biological applications.23–25 Furthermore, to the best of our knowledge, these probes have not yet been used to analyze extracellular AA levels. Compared with traditional organic probes, fluorescent nanoparticles turn out to be more useful because they are more stable in biological samples. Recently, cobalt oxyhydroxide (CoOOH)-modified persistent luminescence nanoparticles (Sr2MgSi2O7:Eu/Dy) were designed by the Tang group for determining the concentration of and imaging AA based on the specific reaction of CoOOH.26 However, these materials need to be prepared at a high temperature (>1000 °C) and in a hydrogen atmosphere, which are disadvantageous for practical applications. Even when in vitro excitation is carried out, the samples cannot be used until their afterglow strength becomes stable. Moreover, their signal becomes attenuated by about 90% compared with the initial intensity, which results in a great reduction of the signal-to-noise ratio. The Lin group presented a novel and efficient fluorescence probe for monitoring AA by using CoOOH modified with carbon dots.27 Using ultraviolet excitation, however, can be affected by the interference of background fluorescence in actual applications of complicated samples. Very recently, the Chu group developed an up-conversion nanoparticle (UCNP) nanosystem for sensing AA, but it did not use a ratio model.28 In particular, hardly any ratiometric fluorescent probes that detect AA have been reported.
Lanthanide-doped UCNPs have the unique ability to convert near infrared (NIR) excitations to UV or visible-light emissions. In recent years, UCNPs have been widely used as promising luminescent probes because of their intriguing properties, which include a large anti-Stokes shift, lack of auto-fluorescence, high penetration depth, and negligible photobleaching.29–34 Based on the fluorescence resonance energy transfer (FRET) process, a highly selective and sensitive sensing system has been assembled by integrating UCNPs and chromophores, with this system displaying a remarkable imaging capacity and outstanding recognition properties. Several groups have developed FRET techniques to detect biologically related ions,35,36 small molecules,37–39 proteins,40,41 nucleic acids,42–44 and other molecular species.45,46
Here, we pursued a new probe that detects AA based on the FRET process. We found that, in the absence of AA, the blue (475 nm wavelength) luminescence of UCNPs can be quenched efficiently by CoOOH, while red (654 nm) luminescence could only be slightly quenched. But in the presence of AA, the blue luminescence of the UCNPs was found to reappear, and this recovery was attributed to the AA-induced conversion of the cobalt species of CoOOH on the UCNP surface to Co2+. This probe for AA was made to be ratiometric by using the red luminescence as a reference signal. Compared with the existing fluorescent probe for AA detection, the presented upconversion nanosystem shows several outstanding features: (1) the CoOOH-assembled UCNPs rapidly detected AA with high selectivity over various other analytes; (2) the probe is available to achieve ratiometric fluorescence changes without the issue of ultraviolet excitation; and (3) auto fluorescence interference from scattered light and biological samples was greatly reduced. These features suggest that the UCNP nanosystem can be applied to directly survey the AA levels in a biological environment.41
Experimental
Synthesis of hydrophobic core–shell UCNPs
The β-NaYF4:Gd/Yb/Tm/Ho samples were prepared by using a Ostwald ripening process similar to those reported previously.47,48 First, cubic NaYF4 UCNPs were synthesized based on a previously reported procedure.49,50 To prepare core–shell UCNPs, Y2O3 (0.25 mmol), Yb2O3 (0.09 mmol), Gd2O3 (0.15 mmol), Tm2O3 (0.005 mmol) and Ho2O3 (0.005 mmol) were added to 2 mL CH3COOH to obtain the lanthanide acetate product. Then 15 mL 1-octadecence and 10 mL oleic acid were added to the lanthanide acetate product. The reaction system was held at 150 °C under a vacuum for 1 h and then cooled down to 50 °C. Subsequently, a solution of 2.5 mmol NaOH and 4.0 mmol NH4F in 10 mL methanol was added and stirred at 50 °C for 2 h. The temperature was increased to 100 °C to evaporate the methanol, and then further raised to 300 °C for 1.5 h under argon protection. Finally, 0.5 mmol cubic NaYF4 UCNPs in 2 mL 1-octadecence were injected into the reaction flask, which was maintained for another hour at 300 °C. When the system was returned to room temperature, the resulting nanoparticles were precipitated out by adding ethanol and carrying out centrifugation at 10
000 rpm. The products were suspended in cyclohexane for use.
Then, in order to convert these hydrophobic UCNPs to hydrophilic ones, an acid treatment was applied to remove the oleate ligands capped on the surface of nanoparticles, and the ligand-free β-NaYF4:Gd/Yb/Tm/Ho@NaYF4 core/shell UCNPs were then obtained.51,52
Preparation of CoOOH-modified UCNPs
A volume of 100 μL of a β-NaYF4:Gd/Yb/Tm/Ho@NaYF4 (10 mg mL−1) aqueous solution and different amounts of CoCl2 (10 mM, 0–200 μL) were added to a 2 mL microcentrifuge tube. A volume of 100 μL of an NaOH (0.8 M) solution and 100 μL of an NaClO (0.2 M) solution were then administered into the tube. The resulting mixture was successively subjected to 10 min ultrasound, centrifugation, three times washing, and re-dispersion in 1 mL of deionized water. The amorphous CoOOH was prepared in a manner similar to that used to prepare CoOOH-modified UCNPs, except that no hydrophilic UCNPs were introduced and that 100 μL of CoCl2 was used.
Luminescence spectroscopy
A volume of 1.0 mL of 1 mg mL−1 CoOOH-modified UCNPs and solutions of various concentrations (0–100 μM) of AA were added to respective quartz cells. To test the selectivity of the nanoprobe, aqueous solutions of NaCl, KCl, MgCl2, CaCl2, CoCl2, NiCl2, FeCl3, CuCl2, ZnCl2, Cd(NO3)2, Hg(ClO4)2, NaClO, H2O2, GSH, L-Cys, Tyr, L-Arg, Lys, Gly, Hcy, Trp, NaHSO3, NaH2PO2, H2C2O4, NaNO2, and Na2S2O3 were used to give for each case a final concentration of 0.1 mM. The fluorescence spectra resulted from excitation of the sample with a 980 nm-wavelength laser and up-conversion luminescence (UCL) spectra (in the wavelength range 325–700 nm) were recorded.
Fluorescence images of cells
Fluorescence images of probe-loaded HeLa cells were obtained by exciting the cells with a mode-locked titanium-sapphire laser source (Mai Tai DeepSee, 80 MHz, 90 fs) set at a wavelength of 980 nm with an Olympus FV1000 laser confocal microscope IX81 with a 40× objective, and a numerical aperture (NA) of 0.6. The image signals at the 450–490 nm and 635–675 nm wavelength ranges were collected by internal PMTs in 12 bit unsigned 1024 × 1024 pixels at a 40 Hz scan speed.
Results and discussion
Design principle of the ratiometric nanoprobe
The design used for the sensing response in CoOOH-modified UCNPs for AA detection was based on a FRET process (Scheme 1). In such a process, NaYF4:Gd/Yb/Tm/Ho@NaYF4 UCNPs with blue up-conversion luminescence (UCL) acted as an energy donor, while the AA-responsive CoOOH assembled on the UCNPs played the part of the energy acceptor. In the absence of AA, the UCNPs excited by the 980 nm-wavelength light exhibited blue light emission at a wavelength of 475 nm and red light emission at 654 nm. Due to the considerable overlap between the wavelengths of light absorbed by CoOOH and the wavelengths of the blue UCL, the blue UCL could be quenched by the CoOOH absorption, whose band was centered at 410 nm, while the wavelength of the red light emission of the UCNPs essentially remained unchanged at 654 nm. The presence of AA apparently caused the conversion of the cobalt species of CoOOH into Co2+ and accompanying collapse and decomposition of CoOOH from the UCNPs, leading to the disappearance of the absorption band at 410 nm. As a result, the FRET process from the blue UCL to CoOOH was suppressed. By using the emission of the red light as an internal reference, recording the changes in the emission ratio I475nm/I654nm allowed us to achieve a quantitative monitoring of AA.
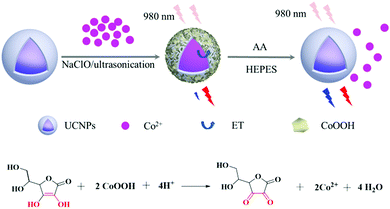 |
| Scheme 1 Proposed mechanism of the sensing system. | |
Synthesis and characterization of CoOOH-modified UCNPs
The core–shell UCNPs were prepared according to the method reported by the Veggel group to obtain effective UCL.47 Compact, cubic NaYF4 UCNPs were prepared as shell precursors, which were then injected into larger core UCNPs to yield core–shell structured UCNPs resulting from ripening-mediated epitaxial growth. Tm and Ho were co-doped into this structure to yield a multicolor emission.53,54 To assemble the CoOOH-modified UCNPs, the OA-UCNPs were treated with an acidic ethanol solution to achieve ligand-free nanoparticles, which were subsequently used to induce the growth of the CoOOH on its surface when CoCl2 solution was added to the system containing NaOH and NaClO. The TEM images of the prepared core–shell UCNPs indicated them to be uniform in size and shape, and their average diameters were measured to be approximately 23 nm (Fig. 1). The XRD analysis results are shown in Fig. S1 (ESI†). The XRD diffraction peaks of the core–shell UCNPs could be assigned to pure β-NaYF4 crystals (JCPDS, Card No. 16-0334). The high-resolution TEM image of the core–shell UCNPs revealed lattice fringes with observed d-spacings of 0.31 nm and 0.52 nm, corresponding to the d-spacing of the (11
0) and (10
0) planes of the hexagonal NaYF4 crystalline structure, respectively. The morphology of CoOOH-modified UCNPs was characterized by using TEM (Fig. 1B and D). EDXA revealed the presence of the elements Y, Yb, Gd, Tm, Ho, Na, F and Co in the CoOOH-modified UCNPs. To further confirm the change of the nanoparticle surface upon being modified with CoOOH, FTIR spectroscopy measurements were taken and the results are shown in Fig. S2 (ESI†). For the obtained CoOOH-modified UCNPs, the peaks at 1625 cm−1 and 571 cm−1 were related to the vibrations of Co–O double and single bonds, respectively. The band at 3437 cm−1 can be attributed to OH bond stretching. The weak peaks centered at 1568 cm−1 and 1412 cm−1 corresponded to characteristic peaks of residual oleic acid (OA) molecules on the UCNPs. The amount of CoOOH was determined using TGA to be approximately 3.79 wt%.
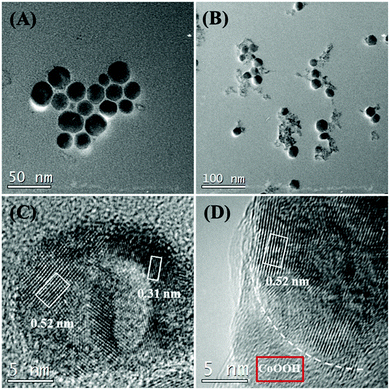 |
| Fig. 1 (A) TEM image of β-NaYF4:Gd/Yb/Tm/Ho@NaYF4. (B) TEM image of CoOOH-modified UCNPs. (C) HRTEM image of β-β-NaYF4:Gd/Yb/Tm/Ho@NaYF4. (D) HRTEM image of part of a CoOOH-modified UCNP. | |
As shown in Fig. S3 (ESI†), the weak absorption peak of CoCl2 was centered at 510 nm, which underwent a hypsochromic shift to about 410 nm when CoOOH was formed. The ET of the nanoprobe was confirmed by the quenching effect of the UCL with various relative amounts of CoOOH. When the concentration of CoCl2 was increased in the presence of NaClO and NaOH, the UCL intensity of the prepared nanoparticles was observed to decrease (Fig. 2). As shown in Fig. S4, (ESI†), the presence of CoOOH on the surface of UCNPs led to a decrease of Tm3+ luminescence lifetime (λ = 475 nm), which further confirms the FRET process from UCNPs to CoOOH. Furthermore, in contrast to the case for the free UCNPs, the physical mixture of CoOOH and UCNPs did not show any marked change in the UCL intensity during the FRET process, while the CoOOH-modified UCNPs did show an obvious decrease in the UCL intensity for this process (Fig. S5, ESI†). The above results showed that the modification of CoOOH on the UCNPs was successful. Since the CoOOH-modified UCNPs tend to sedimentate over time due to the heavy density of CoOOH, the addition of 100 μM CoCl2 were used to prepare CoOOH modified UCNPs for AA determination. The UCL intensity did not show an obvious difference between pH 6.5 and pH 8.5 conditions (Fig. S6, ESI†), which suggested our nanoprobe to be unaffected by small changes in pH under nearly neutral conditions. In particular, no sedimentation was seen in the solution of the CoOOH-modified UCNPs over a period of three hours. This observation mainly resulted from the small size of the prepared nano-probe (Fig. S7, ESI†). In addition, when the as-prepared nanoprobe was dispersed in HEPES (10 mM, pH = 7.4), the UCL ratio (I475/I654) remained essentially constant, clearly suggesting the feasibility of using the developed CoOOH-modified UCNPs for applications in biological settings (Fig. S8, ESI†).
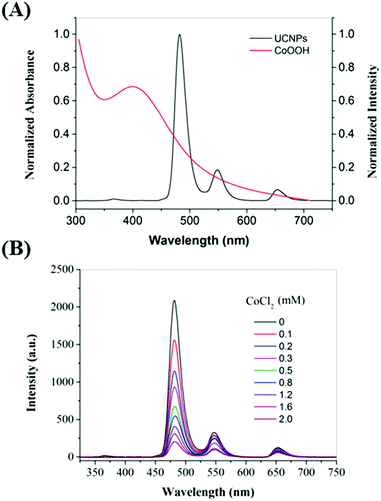 |
| Fig. 2 (A) UV-Vis spectrum of CoOOH (red line) and UCL spectrum of UCNPs (black line). (B) UCL spectra of UCNPs modified with various amounts of CoCl2 (0–2.0 mM). | |
Spectral study and response for AA
The absorption and emission spectra of our probe were then investigated under simulated physiological conditions (10 mM HEPES, pH = 7.4). The absorption of CoOOH-modified UCNPs showed a broad absorption band centered at 410 nm. After the addition of AA, the intensity of the band dramatically decreased (Fig. 3A). Interestingly, the absorbance decreased as the AA concentration was increased from 0 to 100 μM, (Fig. 3B). The decrease of absorbance could be attributed to the reduction of CoOOH, in which the cobalt species of CoOOH was converted to Co2+, in the presence of AA. Corresponding to the changes of UV-vis spectra, the emission intensity at 475 nm increased gradually in the presence of increasing amounts of AA (Fig. 3C). Meanwhile, the UCL emission intensity at 654 nm was almost unchanged. In order to achieve a lower detection limit and improve the signal stability, the ratiometric luminescence signals between blue and red UCL emissions were used to assess the as-prepared probe (Fig. 3D).
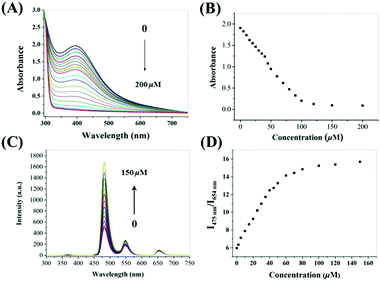 |
| Fig. 3 (A) Absorption spectra of CoOOH-modified UCNPs (1 mg mL−1) with the titration of AA in HEPES (10 mM, pH = 7.4). (B) Absorption at 410 nm vs. AA concentration. (C) UCL spectra of CoOOH-modified UCNPs (1 mg mL−1) with the titration of AA in HEPES (10 mM, pH = 7.4). (D) UCL intensity ratio I475/I654 of CoOOH-modified UCNPs vs. AA concentration. | |
Sensitivity and selectivity to AA
Upon addition of AA to the CoOOH-modified UCNP solution, the ratio of the UCL at 475 to that at 654 nm (I475/I654) increased. As shown in Fig. S9 (ESI†), the reaction of CoOOH-modified UCNPs and AA finished rapidly, in less than one minute, which indicated that the probe can quickly respond to AA. Interestingly, the I475/I654 UCL ratio increased linearly with the concentration of AA in the range 0–40 μM (Fig. S10, ESI†), and then plateaued with the addition of 50 μM AA. The limit of detection for AA was estimated to be 0.63 μM according to the determined UCL ratios. To evaluate the selectivity of CoOOH-modified UCNPs for AA, the UNCPs were also incubated with various biologically relevant amino acids, redox species and important metal ions, and the effects of these added agents on the UCL were measured. As shown in Fig. 4, no significant changes in the ratiometric UCL emission were observed when the system was treated with any of these potentially interfering agents. To understand the high selectivity derived from the specific reaction of CoOOH with AA, cyclic voltammograms of common reductive species (1 mM) were acquired in HEPES (10 mM, pH = 7.4). AA showed a high reductive ability even when using an oxidation potential as low as 0.2 V (Fig. S11, ESI†). The additional reaction specificity may have originated from the relative rapidity of the reaction between CoOOH and AA.21
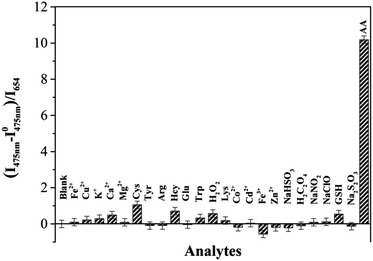 |
| Fig. 4 UCL I475/I468 intensity ratio responses of CoOOH-modified UCNPs to various amino acids, metal ions and redox species (100 μM) in HEPES solutions (10 mM, pH = 7.40). | |
Detection of AA in serum
Due to the advantageous properties shown above for the nanoprobe, such as its low detection limit, high sensitivity, and excellent selectivity, it was tested for how well it can determine the concentration of AA in diluted (25%, v/v) fetal bovine serum. First, the spectra of CoOOH and CoOOH-modified UCNPs in diluted bovine serum were acquired and compared (Fig. 5). There was almost no background fluorescence from serum sample and serum sample with the addition of CoOOH only while a strong UCL was observed in the serum sample with nanoprobe, indicates 980 nm excitation has effectively improved ratio of signal/noise compared with UV excitation, for example, at 380 nm (Fig. S12, ESI†). Then, the diluted serum solution was spiked with different concentrations of AA, and the UCL spectra were recorded. The I475/I654 UCL ratio was observed to increase as the concentration of AA was increased from 0 to 50 μM, and the relationship between the ratio and the AA concentration was observed to be quite linear. Since the concentrations of AA in the sera of men have been measured to range from 20 to 100 μM,55 this CoOOH-modified UCNP nanosystem, if further developed, should be of practical use for the detection of AA.
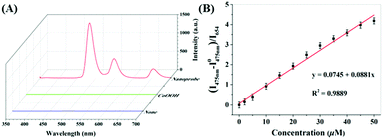 |
| Fig. 5 (A) Background (blue line) and emission spectra of CoOOH (green line) and CoOOH-modified UCNPs (red line) in diluted (25%) fetal bovine serum. (B) Changes in the UCL intensity ratio I475/I654 of CoOOH-modified UCNPs as a function of AA concentration in diluted fetal bovine serum. | |
UCL imaging in the cell
Inspired by the favorable properties of the CoOOH-modified UCNPs nanosystem in vitro, we investigated the ability of the probe to be applied in UCL imaging applications by using a HeLa cell model. The microscopy images showed that the cells not treated with the probe displayed no fluorescence in both the blue (450–490 nm) and red (635–675 nm) channels when they were excited at 980 nm (Fig. 6A). However, when the cells were treated with 1 mM N-ethylmaleimide (an RSS blocking agent that could eliminate interference from GSH, Hcy, Cys and H2S) and then incubated with CoOOH-modified UCNPs, UCL fluorescence was seen in the blue channel as well as the red channel (Fig. 6B). In the control group, the cells were incubated with AA for another 30 min after the probe was loaded (Fig. 6C). In this case, the UCL fluorescence of the blue channel was much brighter than that for the cells not treated with AA. In contrast, the UCL intensity achieved at the red channel hardly changed at all. These results demonstrated the potential of CoOOH-modified UCNPs for imaging AA in living cells.
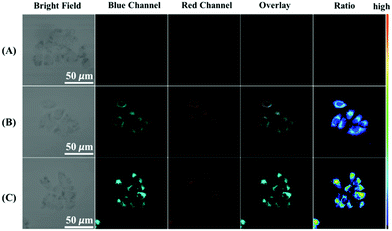 |
| Fig. 6 UCL images of HeLa cells. (A) Cells incubated without CoOOH-modified UCNPs. (B) Cells incubated with NEM (1 mM) for 30 min at 37 °C and then further incubated with 1 mg mL−1 CoOOH-modified UCNPs for 30 min. (C) Cells in this image were treated with AA (100 μM) for 0.5 h after being incubated with NEM and then CoOOH-modified UCNPs. Images were obtained with a 980 nm excitation, and the emission was measured at the blue channel (475 ± 20 nm) and the red channel (655 ± 20 nm). The ratios of the UCL values at the blue channel to those at the red channel are also shown. | |
Conclusions
In summary, a novel core–shell UCL nanoprobe for AA detection has been prepared by assembling cobalt oxyhydroxide onto the surfaces of Tm3+/Ho3+ co-doped up-conversion nanoparticles. By combining the specific reaction between CoOOH and AA with the excellent optical properties of UCNPs, a new AA detection method was developed and was shown to be able to monitor AA levels in diluted fetal bovine serum with high sensitivity, selectivity and cost-efficiency. Furthermore, this probe could be applied to detect intracellular AA. This work represents, to the best of our knowledge, the first time that a UCL probe has been used for ratiometrically sensing AA. The proposed strategy may offer a new approach for developing low-cost and sensitive methods for applications in biology and clinical diagnostics.
Acknowledgements
This study was supported by the NSFC (Grant 21431002) and the Fundamental Research Funds for the Central Universities (lzujbky-2015-k04).
Notes and references
- T. Finkel and N. J. Holbrook, Nature, 2000, 408, 239–247 CrossRef CAS PubMed.
- H. K. Seitz and F. Stickel, Nat. Rev. Cancer, 2007, 7, 599–612 CrossRef CAS PubMed.
- W. R. Wilson and M. P. Hay, Nat. Rev. Cancer, 2011, 11, 393–410 CrossRef CAS PubMed.
- J. R. Smythies, J. R. Soc. Med., 1996, 89, 241 CAS.
- M. Zhang, K. Liu, K. Gong, L. Su, Y. Chen and L. Mao, Anal. Chem., 2005, 77, 6234–6242 CrossRef CAS PubMed.
- R. Oliveira, F. Bento, C. Sella, L. Thouin and C. Amatore, Anal. Chem., 2013, 85, 9057–9063 CrossRef CAS PubMed.
- M. R. Deakin, P. M. Kovach, K. J. Stutts and R. M. Wightman, Anal. Chem., 1986, 58, 1474–1480 CrossRef CAS PubMed.
- S. J. Padayatty, A. Katz, Y. Wang, P. Eck, O. Kwon, J. H. Lee, S. Chen, C. Corpe, A. Dutta, S. K. Dutta and M. Levine, J. Am. Coll. Nutr., 2003, 22, 18–35 CrossRef CAS PubMed.
- M. Sonmez, G. Turk and A. Yuce, Theriogenology, 2005, 63, 2063–2072 CrossRef PubMed.
- M. Zhuang, C. Ding, A. Zhu and Y. Tian, Anal. Chem., 2014, 86, 1829–1836 CrossRef CAS PubMed.
- R. Shakya and D. A. Navarre, J. Agric. Food Chem., 2006, 54, 5253–5260 CrossRef CAS PubMed.
- W.-S. Kim, R. L. Dahlgren, L. L. Moroz and J. V. Sweedler, Anal. Chem., 2002, 74, 5614–5620 CrossRef CAS PubMed.
- M. Zhang, K. Liu, L. Xiang, Y. Lin, L. Su and L. Mao, Anal. Chem., 2007, 79, 6559–6565 CrossRef CAS PubMed.
- X.-Q. Wu, J.-G. Ma, H. Li, D.-M. Chen, W. Gu, G.-M. Yang and P. Cheng, Chem. Commun., 2015, 51, 9161–9164 RSC.
- B. Tang, Y. Wang, M. Du, J.-C. Ge and Z.-Z. Chen, J. Agric. Food Chem., 2003, 51, 4198–4201 CrossRef CAS PubMed.
- L. Hu, L. Deng, S. Alsaiari, D. Zhang and N. M. Khashab, Anal. Chem., 2014, 86, 4989–4994 CrossRef CAS PubMed.
- W. Zhai, C. Wang, P. Yu, Y. Wang and L. Mao, Anal. Chem., 2014, 86, 12206–12213 CrossRef CAS PubMed.
- K. Liu, Y. Lin, P. Yu and L. Mao, Brain Res., 2009, 1253, 161–168 CrossRef CAS PubMed.
- T. Ueno and T. Nagano, Nat. Methods, 2011, 8, 642–645 CrossRef CAS PubMed.
- J. Chan, S. C. Dodani and C. J. Chang, Nat. Chem., 2012, 4, 973–984 CrossRef CAS PubMed.
- T. Maki, N. Soh, K. Nakano and T. Imato, Talanta, 2011, 85, 1730–1733 CrossRef CAS PubMed.
- Y. Matsuoka, M. Yamato, T. Yamasaki, F. Mito and K.-I. Yamada, Free Radical Biol. Med., 2012, 53, 2112–2118 CrossRef CAS PubMed.
- Y. Yang, Q. Zhao, W. Feng and F. Li, Chem. Rev., 2013, 113, 192–270 CrossRef CAS PubMed.
- U. Resch-Genger, M. Grabolle, S. Cavaliere-Jaricot, R. Nitschke and T. Nann, Nat. Methods, 2008, 5, 763–775 CrossRef CAS PubMed.
- L. D. Lavis and R. T. Raines, ACS Chem. Biol., 2008, 3, 142–155 CrossRef CAS PubMed.
- N. Li, Y. Li, Y. Han, W. Pan, T. Zhang and B. Tang, Anal. Chem., 2014, 86, 3924–3930 CrossRef CAS PubMed.
- L. Li, C. Wang, K. Liu, Y. Wang, K. Liu and Y. Lin, Anal. Chem., 2015, 87, 3404–3411 CrossRef CAS PubMed.
- Y. Cen, J. Tang, X. J. Kong, S. Wu, J. Yuan, R. Q. Yu and X. Chu, Nanoscale, 2015, 7, 13951–13957 RSC.
- J. V. Frangioni, Curr. Opin. Chem. Biol., 2003, 7, 626–634 CrossRef CAS PubMed.
- S. Wu, G. Han, D. J. Milliron, S. Aloni, V. Altoe, D. V. Talapin, B. E. Cohen and P. J. Schuck, Proc. Natl. Acad. Sci. U. S. A., 2009, 106, 10917–10921 CrossRef CAS PubMed.
- D. K. Chatterjee, A. J. Rufaihah and Y. Zhang, Biomaterials, 2008, 29, 937–943 CrossRef CAS PubMed.
- G. Chen, T. Y. Ohulchanskyy, A. Kachynski, H. Ågren and P. N. Prasad, ACS Nano, 2011, 5, 4981–4986 CrossRef CAS PubMed.
- D. J. Gargas, E. M. Chan, A. D. Ostrowski, S. Aloni, M. V. P. Altoe, E. S. Barnard, B. Sanii, J. J. Urban, D. J. Milliron, B. E. Cohen and P. J. Schuck, Nat. Nanotechnol., 2014, 9, 300–305 CrossRef CAS PubMed.
- D. Yang, P. a. Ma, Z. Hou, Z. Cheng, C. Li and J. Lin, Chem. Soc. Rev., 2015, 44, 1416–1448 RSC.
- Q. Liu, J. Peng, L. Sun and F. Li, ACS Nano, 2011, 5, 8040–8048 CrossRef CAS PubMed.
- Z. Li, S. Lv, Y. Wang, S. Chen and Z. Liu, J. Am. Chem. Soc., 2015, 137, 3421–3427 CrossRef CAS PubMed.
- D. E. Achatz, R. J. Meier, L. H. Fischer and O. S. Wolfbeis, Angew. Chem., Int. Ed., 2011, 50, 260–263 CrossRef CAS PubMed.
- C. Zhang, Y. Yuan, S. Zhang, Y. Wang and Z. Liu, Angew. Chem., Int. Ed., 2011, 50, 6851–6854 CrossRef CAS PubMed.
- Y. Xiao, L. Zeng, T. Xia, Z. Wu and Z. Liu, Angew. Chem., Int. Ed., 2015, 54, 5323–5327 CrossRef CAS PubMed.
- Y. Wang, L. Bao, Z. Liu and D.-W. Pang, Anal. Chem., 2011, 83, 8130–8137 CrossRef CAS PubMed.
- Y. Wang, P. Shen, C. Li, Y. Wang and Z. Liu, Anal. Chem., 2012, 84, 1466–1473 CrossRef CAS PubMed.
- J. Yuan, Y. Cen, X.-J. Kong, S. Wu, C.-L. Liu, R.-Q. Yu and X. Chu, ACS Appl. Mater. Interfaces, 2015, 7, 10548–10555 CAS.
- C. Lin, M. T. Berry, R. Anderson, S. Smith and P. S. May, Chem. Mater., 2009, 21, 3406–3413 CrossRef CAS.
- X. Hu, Y. Wang, H. Liu, J. Wang, Y. Tan, F. Wang, Q. Yuan and W. Tan, Chem. Sci., 2017 10.1039/C6SC03401B.
- J. Wang, T. Wei, X. Li, B. Zhang, J. Wang, C. Huang and Q. Yuan, Angew. Chem., Int. Ed., 2014, 53, 1616–1620 CrossRef CAS PubMed.
- X. Hu, T. Wei, J. Wang, Z.-E. Liu, X. Li, B. Zhang, Z. Li, L. Li and Q. Yuan, Anal. Chem., 2014, 86, 10484–10491 CrossRef CAS PubMed.
- N. J. J. Johnson, A. Korinek, C. Dong and F. C. J. M. van Veggel, J. Am. Chem. Soc., 2012, 134, 11068–11071 CrossRef CAS PubMed.
- S. Dühnen and M. Haase, Chem. Mater., 2015, 27, 8375–8386 CrossRef.
- H.-X. Mai, Y.-W. Zhang, L.-D. Sun and C.-H. Yan, J. Phys. Chem. C, 2007, 111, 13730–13739 CAS.
- H. Dong, S.-R. Du, X.-Y. Zheng, G.-M. Lyu, L.-D. Sun, L.-D. Li, P.-Z. Zhang, C. Zhang and C.-H. Yan, Chem. Rev., 2015, 115, 10725–10815 CrossRef CAS PubMed.
- P. Huang, W. Zheng, S. Zhou, D. Tu, Z. Chen, H. Zhu, R. Li, E. Ma, M. Huang and X. Chen, Angew. Chem., Int. Ed., 2014, 53, 1252–1257 CrossRef CAS PubMed.
- M. Wang, Z. Chen, W. Zheng, H. Zhu, S. Lu, E. Ma, D. Tu, S. Zhou, M. Huang and X. Chen, Nanoscale, 2014, 6, 8274–8282 RSC.
- X. Xie, N. Gao, R. Deng, Q. Sun, Q.-H. Xu and X. Liu, J. Am. Chem. Soc., 2013, 135, 12608–12611 CrossRef CAS PubMed.
- G. Chen, H. Qiu, P. N. Prasad and X. Chen, Chem. Rev., 2014, 114, 5161–5214 CrossRef CAS PubMed.
- K. R. Dhariwal, W. O. Hartzell and M. Levine, Am. J. Clin. Nutr., 1991, 54, 712–716 CAS.
Footnote |
† Electronic supplementary information (ESI) available: Additional characterization details. See DOI: 10.1039/c6tb02606k |
|
This journal is © The Royal Society of Chemistry 2017 |