DOI:
10.1039/C7SC02688A
(Edge Article)
Chem. Sci., 2017,
8, 8078-8085
Bis(aminothiolato)nickel nanosheet as a redox switch for conductivity and an electrocatalyst for the hydrogen evolution reaction†
Received
16th June 2017
, Accepted 2nd October 2017
First published on 3rd October 2017
Abstract
A π-conjugated coordination nanosheet comprising bis(aminothiolato)nickel (NiAT) moieties was synthesized by the reaction of Ni(acac)2 with 1,3,5-triaminobenzene-2,4,6-trithiol at liquid–liquid and gas–liquid interfaces. The sheet thickness could be controlled down to a single layer (0.6 nm). Selected area electron diffraction and grazing incidence X-ray diffraction analyses indicated the formation of a flat crystalline sheet with a kagome lattice stacked in a staggered alignment. NiAT was reversibly interconverted to a bis(iminothiolato)nickel (NiIT) nanosheet by the chemical 2H+–2e− reaction, which was accompanied by a drastic change in electrical conductivity from 3 × 10−6 to 1 × 10−1 S cm−1. This change in conductivity was explained by the difference in band structures between NiAT and NiIT. NiAT acted as an efficient electrocatalyst for the hydrogen evolution reaction, showing strong acid durability and an onset overpotential of −0.15 V.
Introduction
Two-dimensional materials, such as graphene,1,2 metal oxides,3,4 chalcogenides,5–7 hydroxides,8–10 and covalent organic frameworks,11–14 have attracted much attention because of their unique properties. These materials have various potential applications, including transistors,15–17 sensors,18,19 luminescent devices,20–22 thermal interface materials,23,24 and piezo elements.25 Another type of intriguing 2D material is coordination nanosheets (CONASHs),26,27 which consist of metal complexes and can exhibit various unique physical and chemical features. They are easy to make by convenient bottom-up syntheses, because many coordination reactions proceed in solution under ambient conditions. The oil–water (liquid–liquid) interface can be used as the coordination reaction field for metal ions and ligands if they are soluble only in different phases. Initially, the characterization and structural analysis of CONASHs were the main focuses of research.28–41 Recently, various functionalities of CONASHs have been demonstrated.42–47
2D materials based on the bis(dithiolato)metal complex motif and its analogues comprise important series in CONASHs. Their square-planar geometry is ideal for constructing 2D frameworks. Our first report48 has stimulated their variations, in combination with metal ions (NiII, PdII, and CuI) with aromatic organic ligands (triphenylenehexaol,49,50 benzenehexathiol,48,51–53 triphenylenehexaamine,50,54–57 triphenylenehexathiol,58,59 and benzenehexaamine60). They exhibit high electrical conductivity due to strong charge delocalization in the plane,53–57,61 some of them been theoretically predicted to be 2D topological insulators.62 Moreover, these CONASHs can also act as electrocatalysts: for example, the hydrogen evolution reaction (HER) was catalysed by the bis(dithiolato)metal type of CONASHs.58,63,64
Herein, we add a new aspect in the bis(dithiolato)metal type of CONASHs, by creating a crystalline π-conjugated CONASH comprising bis(aminothiolato)nickel (NiAT, Fig. 1) synthesized by the interfacial reaction of 1,3,5-triaminobenzene-2,4,6-trithiol (L) in water and bis(2,4-pentanedionato)nickel(II) (Ni(acac)2) in CH2Cl2. We recently reported the synthesis of a bis(iminothiolato)nickel (NiIT) nanosheet (Fig. 1),65 although the reaction conditions were different. NiAT has composition and structure similar to those of NiIT, but different coordination modes with each other. The similarity allows NiAT and NiIT to be interconvertible reversibly via the chemical 2H+–2e− reaction per nickel complex unit, while the difference induces a drastic change in electrical conductivity. This change is explained by the obvious difference in band structures between NiAT and NiIT. We also find that NiAT exhibited high, durable catalytic activity for the HER.
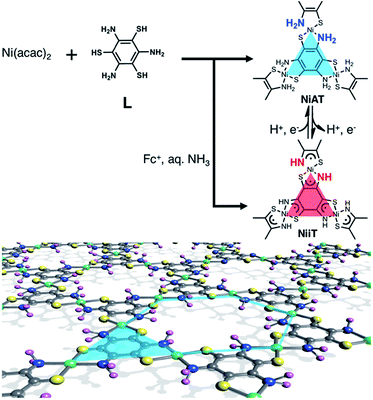 |
| Fig. 1 Schematics and chemical structures of NiAT and NiIT. Grey: C; yellow: S; blue: N; purple: H; green: Ni. | |
Results and discussion
Typical fabrication processes of multi-layered and single-layered NiATvia an interfacial reaction are shown in Fig. 2a and b, respectively. The multi-layered sheets were obtained at the liquid–liquid interface using an aqueous solution of L (1 μM) and a CH2Cl2 solution of Ni(acac)2 (0.5 mM). The bilayer solution was stood at room temperature under argon for 8 days, and a light brown-coloured thin film formed at the liquid/liquid interface. The film was cleaned by repeatedly replacing each solution with pure solvent. The film was vertically transferred onto a flat silicon(111) crystal plate chemically modified with 1,1,1,3,3,3-hexamethyldisilazane [HMDS/Si(111)].
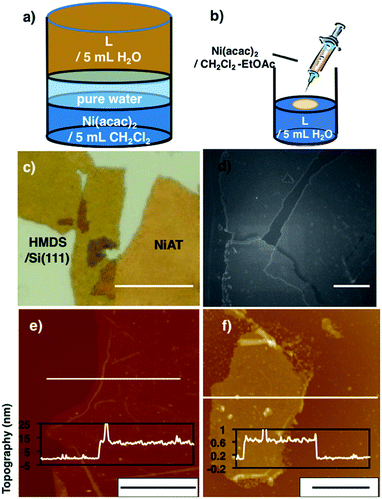 |
| Fig. 2 (a) Schematics of the liquid–liquid interfacial synthesis of multi-layer NiAT. (b) Schematics of the gas–liquid interfacial synthesis of single-layer NiAT. (c) Optical microscope images of multi-layer NiAT on HMDS/Si(111). Scale bars represent 50 μm. (d) FE-SEM images on HMDS/Si(111) of multi-layer NiAT. Scale bars represent 50 nm. (e) AFM image on HMDS/Si(111) and its cross-section analysis along the white line of the multi-layer. Scale bars represent 10 μm. (f) AFM image on HMDS/Si(111) and its cross-section analysis along the magenta line along the white line of the single-layer. Scale bars represent 2 μm. AFM images of (e) and (f) including height distribution bar with roughness analysis along magenta lines (Fig. S1†). | |
Optical microscopy revealed yellow colored film with lateral lengths of more than 50 μm (Fig. 1c) and field-emission scanning electron microscopy (FE-SEM) of the film revealed flat and layered film morphologies (Fig. 1d). Atomic force microscopy (AFM) showed a flat, thin film façade with a thickness of 12 nm (Fig. 2e), which contained ca. 30 layers, based on the thickness of a single layer on [HMDS/Si(111)] of 0.6 nm and the interlayer distance of NiAT of 0.42 nm. Wrinkles and folded sections were observed close to the edge of sheet, suggesting a uniform, pliable sheet material.
Single-layer NiAT was fabricated by a gas–liquid interfacial reaction (Fig. 2b). A tiny amount of Ni(acac)2 in CH2Cl2–EtOAc (10
:
1 v/v) was spread onto an aqueous solution of L (35 μM) under argon. Composition of highly extensible ethyl acetate let the organic solution spread on water surface immediately, leading to rapid evaporation of solvent. After evaporation of the organic solvents, the coordination reaction proceeded at the gas–liquid interface to form an ultrathin film, which was transferred onto HMDS/Si(111). Fig. 2f shows that the film was 0.6 nm thick, corresponding to a single layer.
The chemical structure of the metal complex unit in the film was identified by attenuated total reflectance (ATR)-IR spectroscopy. The multi-layered film showed no S–H stretching vibration signal around 2500 cm−1, whereas this signal was observed for L (Fig. S2†), indicating that all the thiol groups of L participated in coordinating to the Ni centre.48 The amino N–H showed two bands ascribed to antisymmetric and symmetric stretching vibrations, whereas the imino N–H exhibited only one N–H stretching vibration band. The spectrum of the film contained two peaks at 3270 and 3380 cm−1, confirming the presence of the –NH2–Ni motif in NiAT. The previously reported NiIT film showed a single peak at 3270 cm−1 (Fig. 3a).
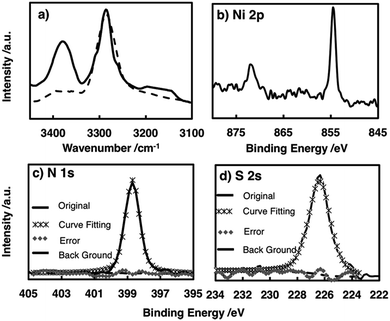 |
| Fig. 3 (a) IR spectra in the N–H stretching vibration region of NiAT (solid line) and NiIT (dashed line). Narrow-scan XPS focusing on (b) the Ni 2p region, (c) the N 1s region, and (d) the S 2s region. | |
X-ray photoelectron spectroscopy (XPS) of NiAT detected N, S, and Ni in a ratio of 1.98
:
2.07
:
1 which was consistent with the ideal ratio of 2
:
2
:
1 and indicated the quantitative formation of nickel complex units in the nanosheets (Fig. 3b–d). For reference, XPS spectra of L, the NiIT nanosheet, and the neutral mononuclear complexes, bis(1-aminobenzene-2-thiolato)nickel(II) (NiAT-M) and bis(1-iminobenzene-2-thiolato)nickel(II) (NiIT-M),66 were also obtained (Fig. S3–S6 and Table S1†). Two Ni 2p peaks at binding energies of 855 and 873 eV for NiAT were assigned to Ni 2p3/2 and Ni 2p1/2, respectively (Fig. 3b). The N 1s peak for NiAT was observed at 398.7 eV (Fig. 3c), slightly lower than that of NiIT at 399.9 eV (Fig. S6 and Table S1†), which was similar to the comparison of NiAT-M and NiIT-M. Only one S 2s peak appeared at 226.5 eV for NiAT (Fig. 3d), indicating that the nickel complex unit was in a neutral oxidation state. In conclusion, the NiAT film contained only one type of bis(aminothiolato)nickel moiety.
The crystal structure of multi-layered NiAT was analysed using diffraction techniques. High-resolution transmission electron microscopy (HR-TEM) showed its sheet-like morphology (Fig. 4a), and selected-area electron diffraction (SAED) found a hexagonal diffraction pattern (Fig. 4b and S6†), implying a hexagonal lattice with a cell length of 1.41 nm. Grazing incidence X-ray diffraction (GIXD) analysis using synchrotron radiation (λ = 1.00 Å) found prominent peaks (Fig. 4d) for NiAT that matched the diffraction pattern of the staggered stacking structure (P
, a = b = 1.405 nm and c = 0.84 nm) rather than that of the eclipsed stacking structure (absence of an in-plane intense peak at 2θ = 13.7°) (Fig. 4e and S7†). Additionally, multiple interlayer distance patterns were subjected to both staggered and eclipsed stacking structures to identify that the interlayer distance of 0.42 nm of staggered stacking structure agrees with the experimental observation (Fig. S7a,† examples of simulation using different interlayer distance). Moreover, slipped-parallel (AB) orientation pattern simulations were performed by fixing an interlayer separation of 0.42 nm with different stacking angels, which again supported the staggered stacking (Fig. S7b,† examples of simulation using different stacking angels). By comparing the observed GIXD profile with the simulated results, the sharp peaks at 2θ = 4.7°, 9.4°, 12.5°, and 21.7° were identified as the in-plane 1 0 0, 2 0 0, 3 1 0, and 5 1 0 diffractions, respectively. GIXD indicated that the present nanosheet had a crystalline hexagonal lattice with an in-plane distance of 1.41 nm and interlayer distance of 0.42 nm.
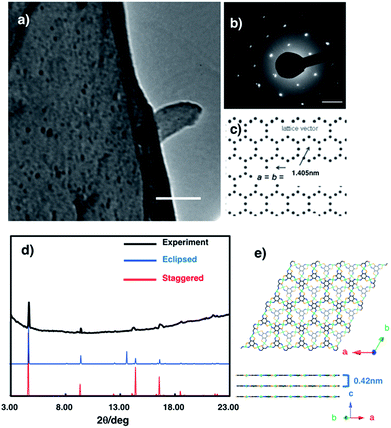 |
| Fig. 4 (a) HR-TEM images of NiAT. Scale bars represent 500 nm. (b) SAED pattern of NiAT. (c) Hexagonal two-dimensional lattice that gives the SAED pattern. Scale bar represents 2 nm−1. (d) Experimental and simulated GIXD patterns of NiAT. (e) Model structures of the staggered stacking structure. | |
The NiAT-M and NiIT-M complexes are reversibly interconverted by a 2H+–2e− reaction;66 therefore, interconversion of NiAT and NiIT was examined. The conversion of NiAT to NiIT was performed by treating NiAT suspended in water with a base (NEt3) followed by adding ferrocenium tetrafluoroborate as an oxidizing agent. The conversion was reversed by the addition of acetic acid followed by the reducing agent, decamethylcobaltocene. This was confirmed directly by monitoring the characteristic N–H stretching signals in the IR spectra (Fig. S8†). The double N–H stretching peaks changed to a single peak upon the chemical oxidation of NiAT, indicating the formation of NiIT, and the double peaks reappeared upon the chemical reduction of NiIT, forming NiAT. Overall, these results demonstrate that NiAT and NiIT are chemically interconvertible without decomposition or a change in shape.
We previously found that pelletized NiIT showed a semiconducting nature with the activation energy of 41 meV and the electrical conductivity of 1 × 10−1 S cm−1 at 298 K.65 Here, we measured the conductivity of pelletized NiAT, finding it to be close to an insulator with the activation energy of 113 meV and the conductivity of 3 × 10−6 S cm−1 at room temperature (Fig. S9†). This implies that NiAT functions as a redox switch of conductivity. The lower conductivity of NiAT was because there were no unpaired free electrons delocalized laterally in the sheet, in contrast to NiIT. The calculated band structures of NiIT (Fig. 5a) and NiAT (Fig. 5b) clearly show a typical kagome band, characterized by a set of Dirac bands and a flat band mostly derived from Ni-dx2−y2 orbitals. The band structure of NiIT indicates a metallic state with high electron density of states at the Fermi level, whereas that of NiAT shows an insulating state with the Fermi level lying inside a gap larger than 1.0 eV. The effect of adding one H atom per N atom to the NiAT sheet is to dope the system with electrons. There are six bands in NiIT between the Fermi level and the band gap above (shaded region in Fig. 5a), which are mainly ascribed to the pz orbitals of C, N, and S. NiAT has 12 more electrons (one contributed by every H atom) than NiIT per unit cell, leading to all the six empty bands being filled, shifting the Fermi level into the band gap. In addition to doping electrons, after adding H, the N valence orbitals are fully filled with a closed shell, so that electrons can hardly hop through the N sites. This effectively decreases the long-range hopping interaction between carbon rings, which in turn narrows the bandwidth and increases electron localization. Moreover, molecular orbitals by DFT calculation showed the frontier orbitals of NiIT to be greatly delocalized over the entire ring, whereas those of NiAT are strongly localized (Fig. S11†). This also indicates much larger charge delocalization in NiIT than NiAT, consistent with the band structure calculation results. These theoretical results suggest that NiIT has a much higher electrical conductivity than NiAT, supporting the experimental observation.
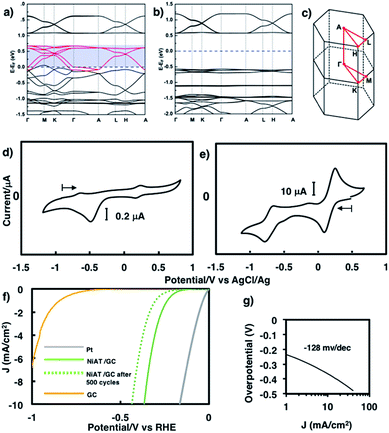 |
| Fig. 5 First-principles band structures of (a) NiAT and (b) NiAT. (c) First Brillouin zone and high-symmetry k-points of NiIT and NiAT. (d) Cyclic voltammogram of (d) NiAT and (e) NiIT in 0.1 M KCl aq. at a scan rate of 50 mV s−1. (f) Linear sweep voltammograms for HER reaction with Pt, NiAT/GC, and GC in 0.05 M H2SO4 and (g) its Tafel slope. | |
Cyclic voltammograms of NiAT and NiIT in 0.1 M KCl are shown in Fig. 5d and e, respectively. NiIT had two quasi-reversible pairs at 0.24 and −0.66 V (Fig. 5e), suggesting that it underwent two successive, reversible one-electron reductions and two successive, one-electron oxidations. NiAT exhibited an irreversible peak at −0.48 V, probably caused by hydrogen migration from the amino group to the nickel centre to form a Ni–H bond.66 The utilization of nanostructures as electrocatalysts for energy conversion technologies are intensively studied because their structures allowed for the sufficient exposure of well-defined active sites.55,58,63,64,67,68 The electrocatalytic activity of NiAT for the HER was examined because the NiAT-M complex exhibits higher catalytic ability for the HER than bis(diamino)nickel or bis(dithiolato)nickel.66NiAT on glassy carbon (GC) protected with Nafion® was used as an electrocatalyst for the HER in acidic and neutral aqueous solutions. As the pH of the solution decreased, the catalytic efficiency increased (Fig. S12†). At pH = 1.3, the onset potential for hydrogen evolution was −0.15 V vs. a reversible hydrogen electrode (RHE) with an operating potential of −0.37 V at 10 mA cm−2 (Fig. 5f) and a Tafel slope of 128 mV dec−1 (Fig. 5g). The HER exchange current density (j0) for the NiAT catalysts obtained from the Tafel plots using the extrapolation method was 0.04 mA cm−2 (Fig. S13†). The Tafel slope in the plots is 128 mV dec−1, which was further confirmed by electrochemical impedance spectroscopy (EIS, Fig. S14†). The charge-transfer Tafel slope derived from the linear fit of the plot of log
Rctversus overpotential was 131 mV dec−1 close to the value obtained by the Tafel plots, indicating not only a charge transfer rate defining step but also a swift electron transfer characteristic of the NiAT catalyst in the HER.69 The turnover frequency (TOF), indicating the number of H2 molecules generated per second per active site, was calculated to be 0.1 s−1 at overpotential of 300 mV, using electrochemical surface area (ECSA, Fig. S15†) of the NiAT sheet by testing the electrochemical double layer capacitance (Cdl) (Fig. S16†). Compared with similar materials such as Co-benzenehexathiol and Co-triphenylenehexathiol complex nanosheets for HER catalysts,64 bis(aminothiolato)nickel motif outperformed bis(dithiolato)cobalt motif in many aspects. Moreover, NiAT exhibited relatively stable performance during 500 HER cycles (Fig. S17,† potential cycling in the range of 0.27 to −0.93 V vs. RHE), XPS of NiAT showed that the component ratios and valence state remained unchanged after 500 HER cycles demonstrating the durability in acidic conditions (Fig. S18†). These results show that NiAT is an efficient, durable electrocatalytic cathode material for hydrogen generation from water.
Conclusions
In conclusion, we synthesized a crystalline CONASH containing NiAT moieties using the liquid–liquid and gas–liquid interfacial reactions. The morphology, composition, and crystal structure of NiAT were characterized by AFM, FE-SEM, XPS, ATR-IR, HR-TEM, and GIXD. NiAT is an insulator and interconvertible to the conducting NiIT nanosheet by a proton-coupled redox reaction. These drastic changes in electrical conductivity were explained by the theoretical calculation of band structures. NiAT showed remarkable performance as an electrochemical HER catalyst. These results demonstrate a rare example of a CONASH acting as a redox switch for conductivity and as an electrocatalyst. Utilization for FET and other catalytic activities and energy storage abilities of this dual CONASH are currently being investigated.
Methods
Materials
All starting material and solvents were purchased from Tokyo Chemical Industry Co., Ltd. Dichloromethane was purified with a glass contour solvent dispensing system (Nikko Hansen & Co., Ltd.). Water was purified using the Milli-Q purification system (Merck). 1,3,5-Triaminobenzene-2,4,6-trithiol (L), mononuclear complex bis(1-aminobenzene-2-thiolato)nickel(II) [NiAT-M], mononuclear complex bis(1-iminobenzene-2-thiolato)nickel(II) [NiIT-M] and were prepared according to the literature.66,70
Substrate preparation
HOPG was purchased from Alliance Biosystems, Inc. (Grade SPI-1 10 × 10 × 2 mm) and cleaved with adhesive tape prior to use. Silicon wafers (p-doped with a carrier concentration of 3 × 1018 cm−3 and resistivity of 1–10 ohm cm) with thermally-grown 100 nm-thick SiO2 were purchased from Yamanaka Semiconductor and cut into squares (10 × 10 mm). 1,1,1,3,3,3-Hexamethyldisilanaze (HMDS)-modified SiO2/Si substrates were prepared by depositing HMDS on SiO2/Si substrates, keeping them under vacuum for 10 min, and then placing them in a flow of Ar. The HMDS-modified SiO2/Si substrates were kept in anhydrous ethanol.
Preparation of multi-layer NiAT
Under an Ar atmosphere, a degassed aqueous solution (10 mL) containing 2.4 × 10−5 mol L−1 of L, and organic layer of a dichloromethane solution of 0.5 mM Ni(acac)2 (10 mL). The organic layer was overlaid slowly with the aqueous L solution. After standing in an Ar atmosphere for 10 days, a yellow nanosheet was obtained at the aqueous/organic interface. After the removal of the aqueous and organic phases, multi-layer NiAT was washed thoroughly with water, ethanol, and dichloromethane, and dried in vacuo at 120 °C. NiAT could be oxidized under long time explosion into air, thus all characterizations and measurements took suitable oxygen segregation treatments. Elemental analysis for NiC4H4N2S2: calcd: C: 23.66%; H: 2.02%; N: 13.81%. Found: C: 23.36%; H: 2.32%; N: 14.12%.
Preparation of single-layer NiAT
A dilute dichloromethane (10 μL)-ethyl acetate (1 μL) solution of Ni(acac)2 (0.5 mM) was gently dropped onto an aqueous solution of L (35 μM) at ambient temperature using a micro syringe. After spontaneous evaporation of the organic solvent, the reaction system was left undisturbed, so that single-layer NiAT was produced at the air–liquid interface. The nanosheet was then transferred onto substrates using the Langmuir–Schaefer method.
Characterization
Optical microscope images were taken using VHX-100 (Keyence Corporation). FE-SEM images were collected using a scanning electron microscope (JSM-7400 FNT, JEOL). The samples were prepared by depositing an ethanol suspension of NiAT on HMDS-modified SiO2/Si substrates. TEM images were recorded with a transmission electron microscope (HF-2000, Hitachi) equipped with a AMT-CCD camera (HISCO, AMT552) at 75 kV. The TEM samples were prepared by depositing an ethanol suspension of NiAT on a carbon film supported by a copper grid. GIXD data were obtained using synchrotron radiation (λ = 1.00 Å) at BL45XU in SPring-8, Japan. Diffractions from a sample were detected using a Pilatus3X 2M detector. Recorded diffraction images were integrated along the Debye–Scherrer ring using a FIT2D software, affording a one-dimensional intensity profile. To prepare the samples, NiAT was deposited on HMDS-modified SiO2/Si substrates. ATR-IR spectra were recorded using an IR spectrometer (FT/IR-6100, JASCO) at room temperature under vacuum. The samples were prepared by depositing NiAT on HMDS-modified SiO2/Si substrates. XPS data were obtained using an XPS microprobe (PHI 5000 VersaProbe, ULVAC-PHI, Inc.). Al Kα (15 kV, 25 W) was used as the X-ray source, and the beam was focused on a 100 μm2 area. The spectra were analyzed with MultiPak (MultiPak Version 9.2.0.5 ULVAC-PHI, Inc.), and standardized using the C (1s) peak at 284.8 eV. Atomic force microscopy measurements were carried out using a scanning probe microscope (5500, Agilent Technologies) under ambient conditions high-amplitude mode (tapping mode) with a silicon cantilever probe (PPP-NCL, Nano World).
SAED and GIXD simulation
To reproduce the obtained SAED and 2D WAXS pattern (Fig. 3d), two types of 3D lattices comprising piles of single-layer NiAT were considered. The two lattices include eclipsed (AA) and staggered (AA−1) stack models, because 6-fold symmetry is required to reproduce the hexagonal diffraction pattern in SAED. First, the atomic arrangement of single-layer NiAT was optimized using density-functional theory implemented in performed on Gaussian 09:71 all the optimized structures were obtained at the B3LYP level of theory. As the basis sets, 6-31G* is used. The optimized cell was then subjected so as to reproduce the observed diffraction data. The eclipsed and staggered stack models were then constructed by using space group of P3 and P
, respectively. Slipped-parallel (AB) orientations pattern simulations were performed using eclipsed (AA) stack models fixing an interlayer separation of 0.42 nm with different stacking angles β. The SAED and GIXD patterns were simulated by implementing CrystalMaker 2.6.3, SingleCrystal 2.3, and CrystalDiffract 6.5.5 (CrystalMaker Software Ltd).
Electrical properties
Electrical conductivity data were collected using the van der Pauw method.72NiAT (2.1 mg) prepared using conditions of described in Preparation of multi-layer NiAT were grinded then pressed with a pressure of 500 kg cm−2 to form self-standing films. The pelletized films were placed on fluoropolymer boards and attached to gold wires using carbon paste (Fujikura Kasei Co., Ltd.). The O2 prevention procedures are applied to every subjectable step.
Band structure calculation
The first-principles band structure calculations based on density-functional-theory method were performed in the framework of the projector augmented wave approach and the generalized gradient approximation of Perdew–Burke–Ernzerhof for the exchange–correlation potentials, as implemented in Vienna ab initio simulation package.73,74 Self-consistent calculations were carried out with an energy cut off of 400 eV on a 5 × 5 × 7 Γ-centered K-point mesh. All atoms were fully relaxed in the structural optimizations until the atomic forces were smaller than 0.01 eV Å−1. Electron spin polarization was also considered. The experimental lattice constants of NiAT and NiIT with AB-stacking pattern were adopted for the band structure calculations.
Electrochemical methods
Electrochemistry experiments were carried out using an electrochemical analyzer (650DT, ALS). A platinum wire served as the auxiliary electrode, and the reference electrode was an Ag/AgCl electrode (silver wire immersed in 0.1 M Bu4NClO4/0.01 M AgClO4/CH3CN), calibrated with a ferrocenium/ferrocene redox potential. To make the working electrode, NiAT (1.7 mg) was placed on 10 mm ϕ aluminum foil to cover entire surface, another 10 mm ϕ carbon paper was put on top to make a “sandwich”, then pressed with a pressure of 500 kg cm−2. For the HER, a 3 mm ϕ GC working electrode was used. Before use, the GC electrodes were polished using aqueous alumina suspensions on felt polishing pads. The potentials for the HER in this study refer to those of the reversible hydrogen electrode (RHE) obtained by adding E(SCE) + 0.059 pH. The aqueous solutions used in the electrochemical experiments were prepared as follows. For cyclic voltammograms of NIAT, KCl (0.1493 g) was dissolved in water (20 mL) to make 0.1 M KCl aqueous solution. For the pH 1.3 solution, H2SO4 (0.0534 mL, c = 98%) was dissolved in water (20 mL). The solutions were deoxygenated with pure Ar before measurement. The catalyst ink was prepared by suspending NiAT (0.2 mg) in ethanol (4 mL). The catalyst ink was pipetted onto the GC surface to achieve the desired catalyst loading. Then, 7 μL of 5% Nafion® solution in ethanol was drop-cast on top to protect the NiAT film. The HER was measured by cyclic voltammetry with a sweep rate of 50 mV s−1. For comparison, the same GC electrode was re-polished after drop casting the catalyst, redrop the same amount of Nafion without catalyst and measured in the same aqueous solution to give a naked GC HER result. Moreover, a 2 mm ϕ Pt electrode was also measured for comparison.
Conflicts of interest
There are no conflicts of interest to declare.
Acknowledgements
This work is supported by JST-CREST “Development of Atomic or Molecular Two-Dimensional Functional Films and Creation of Fundamental Technologies for Their Applications” (JPMJCR15F2) and JSPS KAKENHI Grant Numbers JP15H00862, JP15J06673, JP15K13654, JP16H00900, JP16H00957, JP26220801, JP26708005, JP17H05354, JP17H03028. Work at Utah is supported by US Department of Energy (Grant No. DE FG02-04ER46148). The synchrotron radiation experiments were performed at BL45XU in SPring-8 with the approval of RIKEN (Proposal No. 20160041). XPS measurements were conducted at Advanced Characterization Nanotechnology Platform of the University of Tokyo, supported by “Nanotechnology Platform” of the Ministry of Education, Culture, Sports, Science and Technology (MEXT), Japan. We acknowledge NERSC and CHPC at the University of Utah for providing the computing resources.
References
- K. S. Novoselov, A. K. Geim, S. V. Morozov, D. Jiang, Y. Zhang, S. V. Dubonos, I. V. Grigorieva and A. A. Firsov, Science, 2004, 306, 666 CrossRef CAS PubMed
.
- A. C. Ferrari, F. Bonaccorso, V. Fal'ko, K. S. Novoselov, S. Roche, P. Boggild, S. Borini, F. H. L. Koppens, V. Palermo, N. Pugno, J. A. Garrido, R. Sordan, A. Bianco, L. Ballerini, M. Prato, E. Lidorikis, J. Kivioja, C. Marinelli, T. Ryhanen, A. Morpurgo, J. N. Coleman, V. Nicolosi, L. Colombo, A. Fert, M. Garcia-Hernandez, A. Bachtold, G. F. Schneider, F. Guinea, C. Dekker, M. Barbone, Z. Sun, C. Galiotis, A. N. Grigorenko, G. Konstantatos, A. Kis, M. Katsnelson, L. Vandersypen, A. Loiseau, V. Morandi, D. Neumaier, E. Treossi, V. Pellegrini, M. Polini, A. Tredicucci, G. M. Williams, B. Hee Hong, J.-H. Ahn, J. Min Kim, H. Zirath, B. J. van Wees, H. van der Zant, L. Occhipinti, A. Di Matteo, I. A. Kinloch, T. Seyller, E. Quesnel, X. Feng, K. Teo, N. Rupesinghe, P. Hakonen, S. R. T. Neil, Q. Tannock, T. Lofwander and J. Kinaret, Nanoscale, 2015, 7, 4598 RSC
.
- L. Wang and T. Sasaki, Chem. Rev., 2014, 114, 9455 CrossRef CAS PubMed
.
- R. Ma and T. Sasaki, Acc. Chem. Res., 2015, 48, 136 CrossRef CAS PubMed
.
- S.-L. Li, K. Tsukagoshi, E. Orgiu and P. Samori, Chem. Soc. Rev., 2016, 45, 118 RSC
.
- R. Lv, J. A. Robinson, R. E. Schaak, D. Sun, Y. Sun, T. E. Mallouk and M. Terrones, Acc. Chem. Res., 2015, 48, 56 CrossRef CAS PubMed
.
- M. Bosi, RSC Adv., 2015, 5, 75500 RSC
.
- G. Abellań, C. Marti-Gastaldo, A. Ribera and E. Coronado, Acc. Chem. Res., 2015, 48, 160 CrossRef PubMed
.
- Q. Wang and D. O'Hare, Chem. Rev., 2012, 112, 4124 CrossRef CAS PubMed
.
- R. Ma, Z. Liu, L. Li, N. Iyi and T. Sasaki, J. Mater. Chem., 2006, 16, 3809 RSC
.
- P. J. Waller, F. Gandara and O. M. Yaghi, Acc. Chem. Res., 2015, 48, 3053 CrossRef CAS PubMed
.
- K. Sakaushi and M. Antoniettie, Acc. Chem. Res., 2015, 48, 1591 CrossRef CAS PubMed
.
- M. Dogru and T. Bein, Chem. Commun., 2014, 50, 5531 RSC
.
- X.-H. Liu, C.-Z. Guan, W. Dong and L.-J. Wan, Adv. Mater., 2014, 26, 6912 CrossRef CAS PubMed
.
- Q. H. Wang, K. Kalantar-Zadeh, A. Kis, J. N. Coleman and M. S. Strano, Nat. Nanotechnol., 2012, 7, 699 CrossRef CAS PubMed
.
- R. Cheng, S. Jiang, Y. Chen, Y. Liu, N. Weiss, H.-C. Cheng, H. Wu, Y. Huang and X. Duan, Nat. Commun., 2014, 5, 5143 CrossRef CAS PubMed
.
- M. C. Lemme, T. J. Echtermeyer, M. Baus and H. Kurz, IEEE Electron Device Lett., 2007, 28, 282 CrossRef CAS
.
- F. Schedin, A. K. Geim, S. V. Morzov, E. W. Hill, P. Blake, M. I. Katsnelson and K. S. Novoselov, Nat. Mater., 2007, 6, 652 CrossRef CAS PubMed
.
- J. Yan, M.-H. Kim, J. A. Elie, A. B. Sushkov, G. S. Jenkins, H. M. Milchberg, M. S. Fuhrer and H. D. Drew, Nat. Nanotechnol., 2012, 7, 472 CrossRef CAS PubMed
.
- Y. D. Kim, H. Kim, Y. Cho, J. H. Ryoo, C.-H. Park, P. Kim, Y. S. Kim, S. Lee, Y. Li, S.-N. Park, Y. S. Yoo, D. Yoon, V. E. Dorgan, E. Pop, T. F. Heinz, J. Hone, S.-H. Chun, H. Cheong, S. W. Lee, M.-H. Bae and Y. D. Park, Nat. Nanotechnol., 2015, 10, 676 CrossRef CAS PubMed
.
- Y. J. Zhang, T. Oka, R. Suzuki, J. T. Ye and Y. Iwasa, Science, 2014, 344, 725 CrossRef CAS PubMed
.
- S. Mouri, Y. Miyauchi and K. Matsuda, Nano Lett., 2013, 13, 5944 CrossRef CAS PubMed
.
- Q. Liang, X. Yao, W. Wang, Y. Liu and C. P. Wong, ACS Nano, 2011, 5, 2392 CrossRef CAS PubMed
.
- K. M. F. Shahil and A. A. Balandin, Nano Lett., 2012, 12, 861 CrossRef CAS PubMed
.
- G. C. Rodrigues, P. Zelenovskiy, K. Romanyuk, S. Luchkin, Y. Kopelevich and A. Kholkin, Nat. Commun., 2014, 6, 7572 CrossRef PubMed
.
- M. T. Ong and E. J. Reed, ACS Nano, 2012, 6, 1387 CrossRef CAS PubMed
.
- W. Wu, L. Wang, Y. Li, F. Zhang, L. Lin, S. Niu, D. Chenet, X. Zhang, Y. Hao, T. F. Heinz, J. Hone and Z. L. Wang, Nature, 2014, 514, 470 CrossRef CAS PubMed
.
- A. Dmitriev, H. Spillmann, N. Lin, J. V. Barth and K. Kern, Angew. Chem., Int. Ed., 2003, 42, 2670 CrossRef CAS PubMed
.
- W. Xu, J.-G. Wang, M. Yu, E. Laegsgaard, I. Stensgaard, T. R. Linderoth, B. Hammer, C. Wang and F. Besenbacher, J. Am. Chem. Soc., 2010, 132, 15927 CrossRef CAS PubMed
.
- Y. Li, J. Xiao, T. E. Shubina, M. Chen, Z. Shi, M. Schmid, H.-P. Steinrück, M. Gottfried and N. Lin, J. Am. Chem. Soc., 2012, 134, 6401 CrossRef CAS PubMed
.
- C. S. Kley, J. Čechal, T. Kumagai, F. Schramm, M. Ruben, S. Stepanow and K. Kern, J. Am. Chem. Soc., 2012, 134, 6072 CrossRef CAS PubMed
.
- Z. Shi and N. Lin, J. Am. Chem. Soc., 2009, 131, 5376 CrossRef CAS PubMed
.
- J. Liu, T. Lin, Z. Shi, F. Xia, L. Dong, P. N. Liu and N. Lin, J. Am. Chem. Soc., 2011, 133, 18760 CrossRef CAS PubMed
.
- H. Walch, J. Dienstmaier, G. Eder, R. Gutzler, S. Schlögl, T. Sirtl, K. Das, M. Schmittel and M. Lackinger, J. Am. Chem. Soc., 2011, 133, 7909 CrossRef CAS PubMed
.
- T.-C. Tseng, N. Abdurakhmanova, S. Stepanow and K. Kern, J. Phys. Chem. C, 2011, 115, 10211 CAS
.
- Z. Shi and N. Lin, J. Am. Chem. Soc., 2010, 132, 10756 CrossRef CAS PubMed
.
- M. Matena, M. Stöhr, T. Riehm, J. Björk, S. Martens, M. S. Dyer, M. Persson, J. Lobo-Checa, K. Müller, M. Enache, H. Wadepohl, J. Zegenhagen, T. A. Jung and L. H. Gade, Chem.–Eur. J., 2010, 16, 2079 CrossRef CAS PubMed
.
- J. A. A. W. Elemans, S. Lei and S. D. Feyter, Angew. Chem., Int. Ed., 2009, 48, 7298 CrossRef CAS PubMed
.
- S. Motoyama, R. Makiura, O. Sakata and H. Kitagawa, J. Am. Chem. Soc., 2011, 133, 5640 CrossRef CAS PubMed
.
- R. Makiura, S. Motoyama, Y. Umemura, H. Yamanaka, O. Sakata and H. Kitagawa, Nat. Mater., 2010, 9, 565 CrossRef CAS PubMed
.
- S. Stepanow, N. Lin, D. Payer, U. Schlickum, F. Klappenberger, G. Zoppellaro, M. Ruben, H. Brune, J. V. Barth and K. Kern, Angew. Chem., Int. Ed., 2007, 46, 710 CrossRef CAS PubMed
.
- T. Kambe, T. Kusamoto, R. Sakamoto and H. Nishihara, Macromol. Symp., 2015, 351, 78 CrossRef CAS
.
- K. Takada, R. Sakamoto, S.-T. Yi, S. Katagiri, T. Kambe and H. Nishihara, J. Am. Chem. Soc., 2015, 137, 4681 CrossRef CAS PubMed
.
- R. Sakamoto, K. Hoshiko, Q. Liu, T. Yagi, T. Nagayama, S. Kusaka, M. Tsuchiya, Y. Kitagawa, W.-Y. Wong and H. Nishihara, Nat. Commun., 2015, 6, 6713 CrossRef CAS PubMed
.
- R. Sakamoto, T. Iwashima, M. Tsuchiya, R. Toyoda, R. Matsuoka, J. F. Kögel, S. Kusaka, K. Hoshiko, T. Yagi, T. Nagayama and H. Nishihara, J. Mater. Chem. A, 2015, 3, 15357 CAS
.
- R. Sakamoto, K. Takada, X. Sun, T. Pal, T. Tsukamoto, E. J. H. Phua, A. Rapakousiou, K. Hoshiko and H. Nishihara, Coord. Chem. Rev., 2016, 320–321, 118 CrossRef CAS
.
- H. Maeda, R. Sakamoto and H. Nishihara, Langmuir, 2016, 32, 2527 CrossRef CAS PubMed
.
- T. Kambe, R. Sakamoto, K. Hoshiko, K. Takada, M. Miyachi, J. H. Ryu, S. Sasaki, J. Kim, K. Nakazato, M. Takata and H. Nishihara, J. Am. Chem. Soc., 2013, 135, 2462 CrossRef CAS PubMed
.
- M. Hmadeh, Z. Lu, Z. Liu, F. Gándara, H. Furukawa, S. Wan, V. Augustyn, R. Chang, L. Liao, F. Zhou, E. Perre, V. Ozolins, K. Suenaga, X. Duan, B. Dunn, Y. Yamamto, O. Terasaki and O. M. Yaghi, Chem. Mater., 2012, 24, 3511 CrossRef CAS
.
- M. G. Campbell, S. F. Liu, T. M. Swager and M. Dincă, J. Am. Chem. Soc., 2015, 137, 13780 CrossRef CAS PubMed
.
- K. Hoshiko, T. Kambe, R. Sakamoto, K. Takada and H. Nishihara, Chem. Lett., 2014, 43, 252 CrossRef CAS
.
- T. Pal, T. Kambe, T. Kusamoto, M. L. Foo, R. Matsuoka, R. Sakamoto and H. Nishihara, ChemPlusChem, 2015, 80, 1255 CrossRef CAS
.
- X. Huang, P. Sheng, Z. Tu, F. Zhang, J. Wang, H. Geng, Y. Zou, C.-a. Di, Y. Yi, Y. Sun, W. Xu and D. Zhu, Nat. Commun., 2015, 6, 7408 CrossRef CAS PubMed
.
- D. Sheberla, L. Sun, M. Blood-Forsythe, S. Er, C. R. Wade, C. K Brozek, A. Aspuru-Guzik and M. Dincă, J. Am. Chem. Soc., 2014, 136, 8859 CrossRef CAS PubMed
.
- E. M. Miner, T. Fukushima, D. Sheberla, L. Sun, Y. Surendranath and M. Dincă, Nat. Commun., 2015, 7, 10942 CrossRef PubMed
.
- D. Sheberla, J. C. Bachman, J. S. Elias, C.-J. Sun, Y. Shao-Hornand and M. Dincă, Nat. Mater., 2016, 16, 220 CrossRef PubMed
.
- M. G. Campbell, D. Sheberla, S. F. Liu, T. M. Swager and M. Dincă, Angew. Chem., Int. Ed., 2015, 54, 4349 CrossRef CAS PubMed
.
- R. Dong, M. Pfeffermann, H. Liang, Z. Zheng, X. Zhu, J. Zhang and X. Feng, Angew. Chem., Int. Ed., 2015, 54, 12058 CrossRef CAS PubMed
.
- J. Cui and Z. Xu, Chem. Commun., 2014, 50, 3986 RSC
.
- N. Lahiri, N. Lotfizadeh, R. Tsuchikawa, V. Deshpande and J. Louie, J. Am. Chem. Soc., 2017, 139, 19 CrossRef CAS PubMed
.
- T. Kambe, R. Sakamoto, T. Kusamoto, T. Pal, N. Fukui, K. Hoshiko, T. Shimojima, Z. Wang, T. Hirahara, K. Ishizaka, S. Hasegawa, F. Liu and H. Nishihara, J. Am. Chem. Soc., 2014, 136, 14357 CrossRef CAS PubMed
.
- Z. F. Wang, N. Su and F. Liu, Nano Lett., 2013, 13, 2842 CrossRef CAS PubMed
.
- R. Dong, Z. Zheng, D. C. Tranca, J. Zhang, N. Chandrasekhar, S. Liu, X. Zhuang, G. Seifert and X. Feng, Chem.–Eur. J., 2016, 23, 2255 CrossRef PubMed
.
- A. J. Clough, J. W. Yoo, M. H. Mecklenburg and S. C. Marinescu, J. Am. Chem. Soc., 2015, 137, 118 CrossRef CAS PubMed
.
- X. Sun, K.-H. Wu, R. Sakamoto, T. Kusamoto, H. Maeda and H. Nishihara, Chem. Lett., 2017, 46, 1072 CrossRef
.
- A. Das, Z. Han, W. W. Brennessel, P. L. Holland and R. Eisenberg, ACS Catal., 2015, 5, 1397 CrossRef CAS
.
- J. Hu, C. Zhang, X. Meng, H. Lin, C. Hu, X. Long and S. Yang, J. Mater. Chem. A, 2017, 5, 5995 CAS
.
- Y. An, B. Huang, Z. Wang, X. Long, Y. Qiu, J. Hu, D. Zhou, H. Lin and S. Yang, Dalton Trans., 2017, 46, 10700 RSC
.
- J. Hu, B. Huang, C. Zhang, Z. Wang, Y. An, D. Zhou, H. Lin, M. K. H. Leung and S. Yang, Energy Environ. Sci., 2017, 10, 593 CAS
.
- G. Grandolini and A. Martani, Gazz. Chim. Ital., 1962, 92, 1150 CAS
.
-
M. J. Frisch, et al., Gaussian 09, Revision B.01, Gaussian Inc., Wallingford CT, 2010 Search PubMed
.
- L. J. van der Pauw, Technol. Rev., 1958, 20, 220 Search PubMed
.
- P. E. Blöchl, Phys. Rev. B, 1994, 50, 17953 CrossRef
.
- J. P. Perdew, K. Burke and M. Ernzerhof, Phys. Rev. Lett., 1996, 77, 3865 CrossRef CAS PubMed
.
Footnote |
† Electronic supplementary information (ESI) available. See DOI: 10.1039/c7sc02688a |
|
This journal is © The Royal Society of Chemistry 2017 |