DOI:
10.1039/C7RA13592K
(Paper)
RSC Adv., 2018,
8, 6727-6732
An efficient and sensitive chemosensor based on salicylhydrazide for naked-eye and fluorescent detection of Zn2+†
Received
22nd December 2017
, Accepted 2nd February 2018
First published on 12th February 2018
Abstract
We reported here the synthesis of a diarylethene with a 2,4-dihydroxybenzoyl hydrazine moiety (1O) for Zn2+ recognition. The compound is easy to prepare with a high yield up to 85%. Compound 1O can act as a highly selective and specific fluorescent sensor for Zn2+ without interference by other common metal ions. The LOD for Zn2+ detection was determined to be 1.28 × 10−6 mol L−1. Meanwhile, 1O can be used as a naked-eye detector for the Zn2+ ion with an obvious color change from colorless to olive. Based on the fluorescent properties of 1O, we constructed a logic circuit with four inputs of the combinational stimuli of UV/vis light and Zn2+/EDTA, and one output of fluorescence intensity.
Introduction
As we all know, zinc(II) is the second most abundant and essential element after iron ions (Fe2+ and Fe3+) in the human body and performs a variety of functions.1–3 It is an integral part of numerous enzymes and plays a critical role in various biological processes, such as protein metabolism, the immune system, gene transcription and regulation.4–7 The research on Zn2+ has drawn considerable attention among biologists, chemists, environmentalists and pharmacologists for its chemical and physical properties. The concentration of Zn2+ in the human body ranges from nanomolar (nM) to millimolar (mM)8 and it is indispensable to living organisms. Depletion of biological Zn2+ leads to a decrease in wound health strength as a result of impaired collagen synthesis.9 Zn2+ is a relatively non-toxic element, while its high level is cytotoxic. Unbalanced metabolism of Zn2+ may lead to a series of diseases, such as Alzheimer's disease,10 Parkinson's disease,11 diabetes,12 prostate cancer13 and immune dysfunction.14 It is important for us to maintain the balance of Zn2+ in human body. Therefore, monitoring the distribution and concentration of Zn2+ in environmental or biologic samples becomes important.
The demand for chemosensors that are selective and sensitive for specific target ion is continuously increasing. Numerous sensitive detection methods for metal ion recognition have been widely used in the field of analytical chemistry, biology and environmental processes,15–18 such as mass spectrometry, atomic absorption spectroscopy and high performance liquid chromatography. However, these methods are laborious and require the use of complex equipment. Fluorescence is a powerful tool to detect target ions for its simplicity, easy implementation, high sensitivity and low detection limit.19–21 When a specific fluorescent chemosensor is added to a solution of target metal ion, a color change can be observed accompanied by the changes of fluorescent characteristics. Based on this apparent phenomenon, we can design efficient chemosensors for the recognition of specific ions.
Over the years, many fluorescent chemosensors have been reported for the detection of Zn2+. Several Zn2+ sensors have been developed based on different fluorophores, such as quinoline,22–24 fluorescein,25–28 coumarin,29–32 peptide,33 and pyrene.34 However, most of them lack the smartness in Zn2+ selectivity, sensitivity and interference resistance from Cd2+ ion,35,36 or there is a low yield resulting from complex purification protocols.37–39 As shown in Table 1, most of these sensors can not distinguish Zn2+ from Cd2+.40–44 Zn2+ and Cd2+ are located in the same group of the periodic table and show similar photo-physical changes in these sensors.45 So, an active search of new analytic agents with higher sensitivity and selectivity for Zn2+ continues at the present time.
Table 1 Comparative study of the analytical performance of 1O with other reported sensors
Ref. |
Structure |
LOD (μM) |
Stokes shift (nm) |
Solvent concent |
Interferents |
This Work |
 |
1.28 |
65 |
THF |
None |
39 |
 |
99.1 |
128 |
CH3CN(5%) |
Cd2+ |
40 |
 |
— |
128 |
CH3CN |
Cd2+ |
41 |
 |
0.198 |
42 |
CH3CN(10%) |
Cd2+ |
42 |
 |
1.85 |
110 |
CH3CN(90%) |
Mg2+ |
43 |
 |
10 |
75 |
DMF |
Cd2+ |
Photo-stimuli responsive materials have attracted much attention due to their potential applications in optical devices, controlled release, clean energy, sensors, etc. Until now, plenty of materials based on different photoactive groups have been explored. Spiropyran, spirooxazine, diarylethene and fulgide derivatives are photochromic materials based on photo-induced isomerization involving ring-opening/closing reactions.46,47 Photochromism refers to a reversible change in the properties of a molecule in response to light. Among the various photochromic compounds, diarylethenes are gaining increasing attention in the field of photo-electronics, such as optical memory media and photo-switching devices, due to their high thermal stability, excellent fatigue resistance, and characteristic bistability.48,49
In the current work, we reported a Zn2+ chemosensor with a 2,4-dihydroxybenzoyl hydrazine unit. Salicylhydrazide is one of the important members in Schiff base family because it offers a number of possibilities for different modes of coordination with transition metal ions.50,51 On the other side, considering the advantages of fast response and excellent thermal stability for diarylethene derivatives, we designed and synthesized the compound 1O. The chemosensor detected Zn2+ with high selectivity and specificity accompanied by obvious color changes by stimuli of lights and metal ions. Besides, addition of Zn2+ into the compound 1O resulted in a change in the absorbance spectra, making 1O a naked-eye detector for Zn2+. The photochromic process of the diarylethene derivate was shown in Scheme 1.
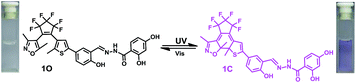 |
| Scheme 1 Synthesis route to diarylethene 1O. | |
Experimental
General methods
All solvents were of analytical purity and were purified by distillation before use. Other reagents were used without further purification. Mass spectra were measured with a Bruker amazon SL Ion Trap Mass spectrometer. 1H NMR and 13C NMR spectra were recorded on a Bruker AV400 (400 MHz) spectrometer with tetramethylsilane as an internal standard. Infrared spectra (IR) were recorded on a Bruker Vertex-70 spectrometer. Melting point was measured on a WRS-1B melting point apparatus. Fluorescence spectra were measured using a Hitachi F-4600 spectrophotometer. The fluorescence quantum yield was measured with an Absolute PL Quantum Yield Spectrometer QY C11347-11. Absorption spectra were measured using an Agilent 8453 UV/vis spectrophotometer with an MUL-165 UV lamp and a MVL-210 visible lamp as equipments of photoirradiation. The solutions of metal ions (0.1 mol L−1) were prepared by the dissolution of their respective metal nitrates in distilled water, except for K+, Ba2+, Mn2+, and Hg2+ (all of their counter ions were chloride ions). Necessary dilutions were made according to each experimental set up. All of the measurements were conducted at room temperature unless otherwise stated.
Synthesis of 1O
Diarylethene 1O was synthesized as presented in Scheme 2. Compound 2 was synthesized according to the previous reported similar method.58 Compound 2 (0.25 g, 0.5 mmol) and 2,4-dihydroxybenzoyl hydrazine (0.1 g, 0.6 mmol) were dissolved in a round-bottom flask with ethanol (20 mL). After refluxed for 4 h until no compound 2 was detected by the TLC silica gel plate. The mixture was cooled to room temperature and concentrated under vacuum. The crude product was purified by recrystallization with ethanol to give compound 1O (0.29 g, 0.46 mmol) as a white solid in 83% yield. Mp 458–459 K; 1H NMR (400 MHz, DMSO-d6, TMS), δ (ppm): 2.00 (s, 3H), 2.06 (s, 3H), 2.28 (s, 3H), 6.35 (s, 1H), 6.40 (d, 1H, J = 8.0 Hz), 7.01 (d, 1H, J = 8.0 Hz), 7.39 (s, 1H), 7.57–7.60 (m, 1H), 7.82 (d, 2H, J = 8.0 Hz), 8.69 (s, 1H), 10.33 (s, 1H), 11.45 (s, 1H), 11.96 (s, 1H), 12.19 (s, 1H). (Fig. S1, ESI†) 13C NMR (DMSO-d6, 100 MHz): 10.13, 11.69, 13.98, 102.70, 102.87, 103.93, 107.06, 107.62, 117.29, 119.40, 121.33, 124.05, 124.12, 125.75, 128.57, 129.87, 140.42, 141.81, 147.25, 157.44, 157.06, 162.04, 162.87, 170.16. (Fig. S2, ESI†) IR (KBr, ν, cm−1): 601, 982, 1064, 1136, 1279, 1612, 3255. (Fig. S3, ESI†). HR-MS (ESI, m/z): [M − H]− calcd for (C29H20F6N3O5S)−, 636.1028; found 636.1034 (Fig. S4, ESI†).
 |
| Scheme 2 Synthesis route to diarylethene 1O. | |
Results and discussion
Photochromic and fluorescent behaviors
The absorption spectrum and fluorescence changes of 1O were measured in tetrahydrofuran (2.0 × 10−5 mol L−1) at room temperature. As shown in Fig. 1, the absorption maximum of 1O was observed at 312 nm (ε = 4.30 × 104 mol−1 L cm−1), which was resulted from π–π* transition.52 On irradiation with 297 nm light, a new absorption band centered at 565 nm (ε = 3.29 × 103 mol−1 L cm−1) appeared and the color of 1O solution turned purple due to the formation of closed-ring isomer 1C. At the same time, the absorption band peaked at 312 nm decreased gradually. Reversely, the purple solution could be completely bleached upon irradiation with visible light (λ > 500 nm) and its absorption spectrum recovered to that of the open-ring isomer 1O. Fig. S5† showed the emission spectral changes of 1O by alternating irradiation with UV and visible light. When excited with 365 nm light, an emission peak of 1O was observed at 580 nm. The fluorescence quantum yield of 1O was determined to be 0.006. On irradiation with 297 nm light, the photocyclization happened and its emission intensity decreased slightly due to the formation of non-fluorescent closed-ring isomer 1C. The fluorescence quantum yield of 1C was determined to be 0.004. The emission intensity of 1O was quenched to ca. 80% at the photostationary state. This phenomenon indicated that the diarylethene unit exhibited relatively low fluorescent modulation efficiency in tetrahydrofuran and it would provide a low background when act as a Zn2+ sensor. The residual fluorescence in the photostationary state might be attributed to the incomplete cyclization reaction and existence of parallel conformations.53,54 Back irradiation with appropriate wavelength visible light regenerated its opening isomer and recovered the original emission intensity.
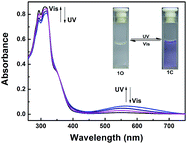 |
| Fig. 1 Absorption spectra and color changes of 1O by photoirradiation. | |
Absorption changes induced by Zn2+/EDTA and UV/vis light
The absorption property of 1O was investigated in tetrahydrofuran (2.0 × 10−5 mol L−1) at room temperature. As shown in Fig. 2A, when different metal ions (5 equiv. 0.1 mol L−1) were added to the solution of 1O, including Zn2+, Al3+, Fe3+, Cr3+, K+, Ba2+, Ca2+, Ni2+, Mg2+, Mn2+, Cd2+, Sr2+, Co2+, Pb2+ and Ag+, the absorption spectral had no obvious changes except Zn2+, Ni2+ and Co2+. New absorption bands centered at 408 nm (ε = 2.09 × 104 mol−1 L cm−1), 438 nm (ε = 1.25 × 104 mol−1 L cm−1) and 420 nm (ε = 1.60 × 104 mol−1 L cm−1) were observed respectively. The colorless 1O solution turned olive on addition of Zn2+, Ni2+ and Co2+ over other metal ions (Fig. 2E). The above results indicated that 1O could be used as a detective colorimetric sensor for Zn2+. But the selectivity is not so good with the interference of Ni2+ and Co2+.
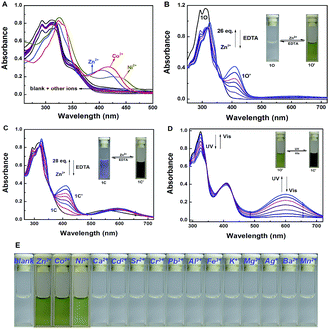 |
| Fig. 2 Changes in absorption spectra and color of 1O induced by Zn2+/EDTA and light in tetrahydrofuran (2.0 × 10−5 mol L−1): (A) changes in absorption spectra on addition of different metal ions (B) 1O induced by Zn2+/EDTA, (C) 1C induced by Zn2+/EDTA, (D) 1O′ induced by UV/vis light, (E) color changes on addition of different metal ions. | |
Under the same experiment conditions, we studied the optimal algorithm of 1O induced by Zn2+ and UV/vis light. As shown in Fig. 2B, with the addition of 26 equiv. of Zn2+ (0.1 mol L−1) to the solution of 1O, a new absorption band centered at 408 nm appeared with the concomitant color change from colorless to olive due to the formation of 1O–Zn2+ complex (1O′) with a much steadier rigid construction than 1O. When Zn2+ was added to the solution of 1C, the absorbance at 410 nm (ε = 1.88 × 104 mol−1 L cm−1) increased, at the same time, the 565 nm absorbance was red-shifted by 35 nm (Fig. 2C). The phenomena might be on account of formation of the 1C–Zn2+ complex (1C′). Then, by addition of excess EDTA (0.1 mol L−1) to the solution of 1O′ or 1C′, the absorption band all recovered to 1O or 1C because EDTA possibly stripped Zn2+ away from the cavity by the binding zone. Furthermore, as shown in Fig. 2D, when the maximum absorption band of 1O′ was reached, upon irradiation with 297 nm UV light, a new absorption band appeared clearly which centered at 600 nm (ε = 1.44 × 104 mol−1 L cm−1) and the color changed from olive to dark slate gray for the formation of the closed-ring isomer 1C′. Meanwhile, it could return back to the open-ring isomer 1O′ on irradiation with visible light (λ > 500 nm), indicating that the open-ring isomer 1O′ and the closed-ring isomer 1C′ reaction was reversible.
Fluorescence response to metal ions
Under the same experimental conditions, Fig. 3 showed the emission spectral and fluorescence color changes of 1O induced by various metal ions (5 equiv. 0.1 mol L−1) such as Zn2+, Al3+, Fe3+, Cr3+, K+, Ba2+, Ca2+, Ni2+, Mg2+, Mn2+, Cd2+, Sr2+, Co2+, Pb2+ and Ag+. We found that 1O can detect Zn2+ in tetrahydrofuran (2.0 × 10−5 mol L−1). The fluorescence of 1O was notably changed when Zn2+ was added, while the addition of other cations caused no obvious changes (Fig. 3A). When Zn2+ was added to the solution of 1O, the fluorescence intensity was enhanced evidently and the emission peak was blue shifted from 580 nm to 515 nm with a concomitant fluorescent color change from dark to bright aliceblue caused by the formation of 1O′. The results showed that there was no interference of Cd2+ or Mg2+, indicating good selectivity of Zn2+. Therefore, 1O can be used as an efficient fluorescence chemosensor for Zn2+ recognition.
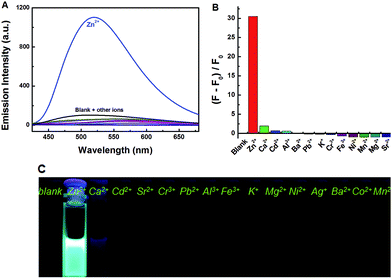 |
| Fig. 3 Fluorescence changes of 1O induced by the addition of various metal ions (5.0 equiv.): (A) emission intensity in tetrahydrofuran; (B) emission intensity; (C) photos of fluorescence. | |
To further evaluate the responsive nature of 1O induced by Zn2+, a series of fluorescence titration tests were carried out in tetrahydrofuran (2.0 × 10−5 mol L−1) at room temperature. When Zn2+ was added to the solution of 1O from 0 to 18 equiv., the fluorescence intensity increased significantly with a blue shift of 65 nm from 580 nm to 515 nm (Fig. 4A). The titration experiment was shown in Fig. S6.† Compared with 1O, the fluorescence intensity was enhanced by 31 fold. The increased emission intensity and blue shift could be ascribed to the formation of 1O′. The fluorescent quantum yield of 1O′ was determined to be 0.044. The stable chelation of 1O with Zn2+ inhibited the C
N isomerization and led to a rigid fluorophore structure, causing enhanced fluorescence intensity.55,56 The fluorescence spectrum of 1O′ recovered to that of 1O by adding an aqueous solution of excess EDTA (0.1 mol L−1) which possibly strips Zn2+ away from the cavity by the binding zone, indicating that the complexation–decomplexation reaction between 1O and Zn2+ was reversible. Similar to 1O, the fluorescence of 1C could also be effectively modulated by Zn2+ in tetrahydrofuran. After adding 8.0 equiv. of Zn2+ to the solution of 1C, its fluorescence intensity was enhanced by 4 folds and the emission peak was blue-shifted from 580 to 530 nm due to the formation of 1C′ (Fig. 4B). We also investigated the photochromism of 1O′. As shown in Fig. 4C, when the maximum intensity was reached, upon irradiation with 297 nm UV light, the emission intensity of 1O′ was quenched to ca. 15% due to the formation of 1C′, the fluorescent quantum yield of 1C′ was determined to be 0.028. It can come back to that of 1O′ by irradiation with appropriate visible light and followed by a color change from bright aliceblue to powderblue. The irradiation with UV/vis light reaction between 1O′ and 1C′ was also reversible.
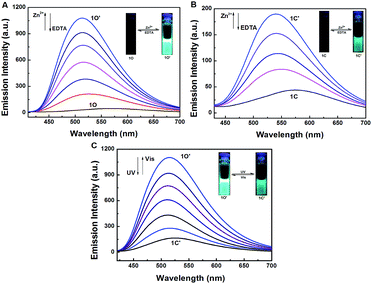 |
| Fig. 4 Changes in fluorescence and color of 1O induced by Zn2+/EDTA and light in tetrahydrofuran (2.0 × 10−5 mol L−1): (A) 1O induced by Zn2+/EDTA, (B) 1C induced by Zn2+/EDTA, (C) 1O′ triggered by light. | |
To investigate the coordination mode of 1O with Zn2+, Job's plot analysis was performed according to the reported method.57 As shown in Fig. 5A, the maximum value was achieved when the molar fraction of [1O]/([1O] + [Zn2+]) was about 0.5, demonstrating a 1
:
1 stoichiometry between 1O and Zn2+. Based on the 1
:
1 stoichiometry and fluorescence titration data, the association constant (Ka) of 1O with Zn2+ was calculated from the slope and intercept of the linear plot to be 7.17 × 103 L mol−1 (R = 0.984) (Fig. 5B). The detection limit was calculated to be 1.28 × 10−6 mol L−1 for Zn2+ (Fig. 5C). To further confirm the coordination mode of 1O and Zn2+, ESI mass spectra were recorded. The ESI-MS peak at 699.1031 assigned to [1O + Zn2+ − 3H]− (calcd 699.0241) was observed (Fig. S7†), providing strong evidence for the formation of a 1
:
1 complex between 1O and Zn2+. The proposed binding mode between 1O and Zn2+ was shown in Scheme 3.
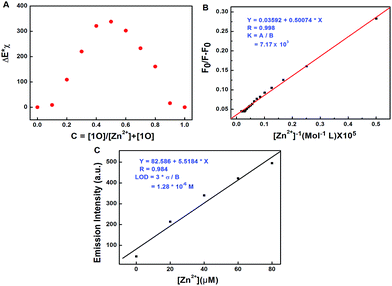 |
| Fig. 5 (A) Job's plot showing the 1 : 1 complex of 1O–Zn2+ in tetrahydrofuran, (B) Hildebrand–Benesi plot based on the 1 : 1 for 1O, the binding constant of 1O with Zn2+ was calculated to be 7.17 × 103 L mol−1, (C) the limit of detection (LOD), LOD is 1.28 × 10−6 mol L−1. | |
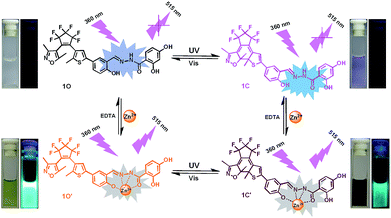 |
| Scheme 3 Photochromism, color and fluorescence changes of 1O induced by Zn2+/EDTA and lights. | |
Application in logic circuit
On the basis of the fact that the absorption and fluorescent intensity of the target diarylethene could be effectively modulated by Zn2+/EDTA and light, a type of logic circuits was constructed by using light irradiation and Zn2+/EDTA as the input signals. The fluorescence at 515 nm was used as an output signal. As shown in Fig. 6, the photochromic behaviors of 1O could be effectively modulated by Zn2+/EDTA and UV/vis light. Thus, one logic circuit was constructed by using the combination of four input signals (In1: 297 nm UV light, In2: λ > 500 nm visible light, In3: Zn2+, and In4: EDTA) and an output (fluorescence emission at 515 nm). The four inputs and one output could be either “on” or “off” state with different Boolean values. When 297 nm light was employed, In1 was switched to “on” state with a Boolean value of “1”. Similarly, In2 was “1” corresponding to irradiation with appropriate visible light (λ > 500 nm), In3 was “1” corresponding to the addition of Zn2+, and In4 was “1” corresponding to the addition of EDTA. The emission intensity of 1O at 515 nm was regarded as the initial value and when the change of fluorescence intensity at 515 nm was 31 fold larger than the initial value, it was regarded as “on” state with a Boolean value of “1”. Otherwise, it was regarded as “off” state with a Boolean value of “0”. Upon the stimuli of different inputs, the diarylethene exhibited an on–off–on photochromic switching behavior. As a result, 1O could read a string of four inputs and write one output (Table 2).
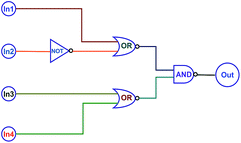 |
| Fig. 6 The combinational logic circuits equivalent to the truth table give in Table 1, In1 (297 nm UV light), In2 (λ > 500 nm light), In3 (Zn2+), In4 (EDTA) and output (λem = 515 nm). | |
Table 2 Truth table for all possible strings of four binary-input date and the corresponding output digit
Inputs |
Output λem = 515 nm |
In1 (UV) |
In2 (vis) |
In3 (Zn2+) |
In4 (EDTA) |
0 |
0 |
0 |
0 |
0 |
1 |
0 |
0 |
0 |
0 |
0 |
1 |
0 |
0 |
0 |
0 |
0 |
1 |
0 |
1 |
0 |
0 |
0 |
1 |
0 |
1 |
1 |
0 |
0 |
0 |
1 |
0 |
1 |
0 |
1 |
1 |
0 |
0 |
1 |
0 |
0 |
1 |
1 |
0 |
1 |
0 |
1 |
0 |
1 |
0 |
0 |
0 |
1 |
1 |
0 |
1 |
1 |
1 |
0 |
0 |
1 |
1 |
0 |
1 |
0 |
1 |
0 |
1 |
1 |
0 |
0 |
1 |
1 |
1 |
0 |
1 |
1 |
1 |
1 |
0 |
Conclusions
In summary, a highly sensitive fluorescent “turn-on” sensor based on a photochromic diarylethene derivative with a salicylhydrazide unit was developed. It exhibited excellent photochromic properties, high selectivity and specificity toward Zn2+ over other metal ions. It could also be used as a naked-eye detector for Zn2+. Furthermore, the diarylethene showed excellent fluorescent switching behaviors with distinctive color changes in response to the combinational inputs of light and Zn2+. Based on these characteristics, a logic circuit was designed by the fluorescence intensity as the output signal with the inputs of UV/vis lights and Zn2+/EDTA. All these results will be helpful for the design and construction of new diarylethene derivatives with multi-addressable states and potential applications in fluorescent sensors for special ions.
Conflicts of interest
There are no conflicts of interest to declare.
Acknowledgements
This work was supported by the Science Funds of Natural Science Foundation of Jiangxi Province (20171ACB20025); the Project of the Science Funds of Jiangxi Education Office (GJJ160795); the “5511” Science and Technology Innovation Talent Project of Jiangxi Province (20165BCB18015); the Project of Jiangxi Science and Technology Normal University Advantage Sci-Tech Innovative Team (2015CXTD002).
Notes and references
- C. Andreini, L. Banci and I. Bertini, et al., J. Proteome Res., 2006, 5, 3173–3178 CrossRef CAS PubMed.
- P. S. Hariharan and S. P. Anthony, Anal. Chim. Acta, 2014, 848, 74–79 CrossRef CAS PubMed.
- A. Bhattacharyya, S. Ghosh and S. C. Makhal, et al., Spectrochim. Acta, Part A, 2017, 183, 306–311 CrossRef CAS PubMed.
- S. Enthaler, X. F. Wu and M. Weidauer, et al., Inorg. Chem. Commun., 2014, 46, 320–323 CrossRef CAS.
- Y. Han, C. Fu and J. Kuang, et al., Postharvest Biol. Technol., 2016, 116, 8–15 CrossRef CAS.
- H. Yang, Z. Y. Huang and J. Li, et al., Ecol. Indic., 2014, 45, 103–109 CrossRef CAS.
- D. K. Khajuria, C. Disha and R. Vasireddi, et al., Mater. Sci. Eng., C, 2016, 63, 78–87 CrossRef CAS PubMed.
- C. Andreini, L. Banci and I. Bertini, et al., J. Proteome Res., 2006, 5, 3173–3178 CrossRef CAS PubMed.
- M. Saleem and K. H. Lee, RSC Adv., 2015, 5, 72150–72287 RSC.
- X. Fang, H. Li and G. Zhao, et al., Biosens. Bioelectron., 2013, 42, 308–313 CrossRef CAS PubMed.
- M. Hosseini, A. Ghafarloo and M. R. Ganjali, et al., Sens. Actuators, B, 2014, 198, 411–415 CrossRef CAS.
- K. Vogt, J. Mellor and G. Tong, et al., Neuron, 2000, 26, 187–196 CrossRef CAS PubMed.
- A. Takeda, M. Nakamura and H. Fujii, et al., Metallomics, 2013, 5, 417–423 RSC.
- B. Szewczyk, Front. Aging Neurosci., 2013, 5, 33 Search PubMed.
- S. Kumari, S. Joshi and T. C. Cordova-Sintjago, et al., Sens. Actuators, B, 2016, 229, 599–608 CrossRef CAS.
- S. Wang, X. Fei and J. Guo, et al., Talanta, 2015, 148, 229–236 CrossRef PubMed.
- H. Nie, W. Yang and M. Yang, et al., Dyes Pigm., 2016, 127, 67–72 CrossRef CAS.
- S. Mukherjee, P. Mal and H. Stoeckli-Evans, J. Lumin., 2016, 172, 124–130 CrossRef CAS.
- H. N. Kim, W. X. Ren and J. S. Kim, et al., Chem. Soc. Rev., 2012, 41, 3210–3244 RSC.
- C. Kar, M. D. Adhikari and A. Ramesh, et al., RSC Adv., 2012, 2, 9201–9206 RSC.
- Y. L. Pak, K. M. K. Swamy and J. Yoon, Sensors, 2015, 15, 24374–24396 CrossRef PubMed.
- M. Sohrabi, M. Amirnasr and H. Farrokhpour, et al., Sens. Actuators, B, 2017, 250, 647–658 CrossRef CAS.
- V. K. Gupta, A. K. Singh and L. K. Kumawat, et al., Sens. Actuators, B, 2016, 222, 468–482 CrossRef CAS.
- G. Kasirajan, V. Krishnaswamy and N. Raju, et al., J. Photochem. Photobiol., A, 2017, 341, 136–145 CrossRef CAS.
- B. Vidya, G. Sivaraman and R. V. Sumesh, et al., ChemistrySelect, 2016, 1, 4024–4029 CrossRef CAS.
- W. Wang, Y. N. Lei and Q. L. Liu, et al., Heterocycles, 2016, 92, 1204–1214 CrossRef CAS.
- Q. Li, Y. Zhang and Y. Jin, et al., RSC Adv., 2015, 5, 68815–68821 RSC.
- J. An, M. Yan and Z. Yang, et al., Dyes Pigm., 2013, 99, 1–5 CrossRef CAS.
- A. Bhattacharyya, S. Ghosh and S. C Makhal, et al., Spectrochim. Acta, Part A, 2017, 183, 306–311 CrossRef CAS PubMed.
- N. Roy, A. Dutta and P. Mondal, et al., J. Fluoresc., 2017, 1–15 Search PubMed.
- L. Fan, J. Fluoresc., 2017, 1–7 CAS.
- T. Liu, J. Yin and Y. Wang, et al., J. Electroanal. Chem., 2016, 783, 304–307 CrossRef CAS.
- C. Wu, Y. Ikejiri and J. L. Zhao, et al., Sens. Actuators, B, 2016, 228, 480–485 CrossRef CAS.
- X. Lu, W. Zhu and Y. Xie, et al., Chem.–Eur. J., 2010, 16, 8355–8364 CrossRef CAS PubMed.
- M. Li, H. Y. Lu and R. L. Liu, et al., J. Org. Chem., 2012, 77, 3670–3673 CrossRef CAS PubMed.
- S. Zhu, J. Zhang and J. Janjanam, et al., J. Mater. Chem. B, 2013, 1, 1722–1728 RSC.
- S. C. Burdette, G. K. Walkup and B. Spingler, et al., J. Am. Chem. Soc., 2001, 123, 7831–7841 CrossRef CAS PubMed.
- L. Wang, W. Qin and X. Tang, et al., Org. Biomol. Chem., 2010, 8, 3751–3757 CAS.
- T. Mistri, M. Dolai and D. Chakraborty, et al., Org. Biomol. Chem., 2012, 10, 2380–2384 CAS.
- E. Hao, T. Meng and M. Zhang, et al., J. Phys. Chem. A, 2011, 115, 8234–8241 CrossRef CAS PubMed.
- X. Zhou, P. Li and Z. Shi, et al., Inorg. Chem., 2012, 51, 9226–9231 CrossRef CAS PubMed.
- C. Gao, X. Jin and X. Yan, et al., Sens. Actuators, B, 2013, 176, 775–781 CrossRef CAS.
- Y. Li, J. Wu and X. Jin, et al., Dalton Trans., 2014, 43, 1881–1887 RSC.
- N. Roy, A. Dutta and P. Mondal, et al., Sens. Actuators, B, 2016, 236, 719–731 CrossRef CAS.
- W. K. Dong, X. L Li and L. Wang, et al., Sens. Actuators, B, 2016, 229, 370–378 CrossRef CAS.
- R. Pardo, M. Zayat and D. Levy, Chem. Soc. Rev., 2011, 40, 672–687 RSC.
- F. Liu and K. Morokuma, J. Am. Chem. Soc., 2013, 135, 10693–10702 CrossRef CAS PubMed.
- J. Zhang, Q. Zou and H. Tian, Adv. Mater., 2013, 25, 378–399 CrossRef CAS PubMed.
- A. Uyama, S. Yamazoe and S. Shigematsu, et al., Langmuir, 2011, 27, 6395–6400 CrossRef CAS PubMed.
- H. Tian and S. Yang, Chem. Soc. Rev., 2004, 33, 85–97 RSC.
- M. Irie, T. Fukaminato and K. Matsuda, et al., Chem. Rev., 2014, 114, 12174–12277 CrossRef CAS PubMed.
- Z. X. Li, L. Y. Liao and W. Sun, et al., J. Phys. Chem. C, 2008, 112, 5190–5196 CAS.
- C. Fan, S. Pu and G. Liu, et al., J. Photochem. Photobiol., A, 2008, 197, 415–425 CrossRef CAS.
- Y. Fu, Y. Tu and C. Fan, et al., New J. Chem., 2016, 40, 8579–8586 RSC.
- J. S. Wu, W. M. Liu and X. Q. Zhuang, et al., Org. Lett., 2007, 9, 33–36 CrossRef CAS PubMed.
- P. S. Hariharan and S. P. Anthony, RSC Adv., 2014, 4, 41565–41571 RSC.
- H. A. Benesi and J. H. Hildebrand, J. Am. Chem. Soc., 1949, 71, 2703–2707 CrossRef CAS.
- S. Pu, Z. Tong and G. Liu, et al., J. Mater. Chem. C, 2013, 1, 4726–4739 RSC.
Footnotes |
† Electronic supplementary information (ESI) available. See DOI: 10.1039/c7ra13592k |
‡ These authors contributed equally to this work. |
|
This journal is © The Royal Society of Chemistry 2018 |