DOI:
10.1039/C7RA09355A
(Paper)
RSC Adv., 2017,
7, 45442-45451
Controlled formation of flavor compounds by preparation and application of Maillard reaction intermediate (MRI) derived from xylose and phenylalanine†
Received
23rd August 2017
, Accepted 19th September 2017
First published on 25th September 2017
Abstract
An effective method for preparing Maillard reaction intermediate (MRI) derived from xylose (Xyl) and phenylalanine (Phe) in aqueous medium was proposed in this study. Maillard reaction products (MRPs) were prepared by Maillard reaction performed under stepwise increase of temperature, and cysteine was added as an indicator. The formation conditions of MRI generated from Xyl and Phe were determined through MRPs browning analysis. The MRI prepared under the determined formation conditions was purified using RP-HPLC, and characterized using UPLC-ESI-mass spectra and NMR. The molecular mass (297.1 Da) and formula (C14H19O6N) were proposed. The capacity of flavor formation of heated MRI was evaluated through GC-MS. Results showed that flavor compounds were formed during the subsequent heat treatment, and MRI could be a great potential substitute of MRPs in preparation of fresh process flavors. Therefore, controlled formation of process flavors could be achieved by preparation and application of MRI.
1. Introduction
The Maillard reaction is a non-enzymatic browning reaction occurring between amino and carbonyl groups during heat treatment and cooking. It is regarded as one of the most important reactions during food processing and storage.1–3 Maillard reaction is also a very important reaction for generating aroma compounds such as aldehyde, ketone, furan, pyrrole, etc.4 Maillard reaction products (MRPs) are usually used for flavoring production.5 They have been favored by consumers over years and intrigued by researchers due to their rich fragrance.5 Deblander et al.6 attempted to synthesize the Maillard key flavor compounds: 2-acetyl-1-pyrroline, 6-acetyl-1,2,3,4-tetrahydropyridine and 5-acetyl-2,3-dihydro-4H-1,4-thiazine by chemical synthesis. However, since most of the flavor components in MRPs are volatile, it is difficult to maintain the stability of flavored end products for application, especially during heat treatment, cooking and baking process. The loss of aroma and fragrance limits the application of MRPs. Maillard reaction is divided into three stages namely, initial, intermediate and final stage. During the first step, the reactions are initiated by aldoses and amides which lead to the formation of a labile N-substituted amino sugar. Specifically, after the nucleophilic addition, the Schiff base can rearrange via 1,2-eneaminol leading to Amadori rearrangement products (ARPs).7 During initial stage of Maillard reaction, neither aromas nor melanoidins are produced, but significant non-volatile aroma precursors such as Maillard reaction intermediates are formed.8 Compared with MRPs, MRIs have stable physicochemical properties.9 When heated, MRIs undergo dehydration and fission reaction as the Maillard reaction progresses and generate colourless reductones and heterocyclic compounds.10 These products could dehydrate and polymerize to form melanoidins that give food a tempting dark color. Though sugars and amino acids are also regarded as MRPs precursors, MRI shows higher reactivity in color and flavor formation than the reactant saccharides and amino acids.11 Hence MRI may have uniquely excellent potential as an alternative to conventional Maillard flavorings, and the foodstuff with MRI as flavoring additive could not exhibit its flavor during primary processing or storage at room temperature but generate desirable flavor during heat treatment such as cooking and baking. It ultimately leads to controlled formation of process flavors and avoids the volatility drawbacks of conventional Maillard flavorings. Additionally, Davidek et al.12 prepared ARP in an aqueous medium; however, the formation conditions of MRI were not determined. It is difficult to detect Maillard reaction intermediates in water and their generation conditions are difficult to determine, especially in a system with the presence of a variety of amino compounds. Thus MRIs are seldom prepared in aqueous medium.13–15
Huang et al.16,17 studied the inhibition of color formation in aqueous Maillard reaction of soybean peptide and xylose. They reported that browning could be well inhibited by cysteine, and effective inhibition only occurred when cysteine was added to the system after the first mild reaction step. Further research showed that once the pyrolysis products from carbohydrates were formed in Maillard reaction, the browning could not be inhibited by cysteine. But if cysteine reacted with MRI, the generation of browning substances was effectively restrained, and the low molecular weight compounds formed in intermediate reaction stage were significantly reduced. Therefore, cysteine could be applied as an efficient indicator to detect the formation of MRI in aqueous medium. According to Arrhenius equation, chemical reaction can be slowed down with decrease in temperature. Low temperature was selected for Maillard reaction to reduce the decomposition of MRI and improve the availability of MRI reacting with cysteine. The stepwise increase of temperature method was carried out as follows: amino acid and sugar solution was heated at low temperature for different times, and then cysteine was added to the reaction system (first mild reaction step), the thermal reaction was further carried out at higher temperature for certain time (elevated temperature reaction step) and various color strengths of final MRPs were detected. Then the addition moment of cysteine, at which the lightest color of MRPs was observed, could confirm the MRI formation time.
In the research of Huang et al.,16,17 cysteine was added to the system as a reactant to produce light-colored MRPs. Nevertheless, in the proposed method, cysteine was added as an indicator for the detection of MRI formation and was not further used for MRI preparation.
Maillard reaction of reducing sugar and amino acids is a very important experimental model system for flavor formation.18 For instance, benzaldehyde, benzeneacetaldehyde etc. were found in previous reported researches during the Maillard reaction of xylose (Xyl) and phenylalanine (Phe), which were identified as the typical flavor compounds of flowers.19,20 In this research, Xyl and Phe were mixed in aqueous solution, then Maillard reaction under stepwise increase of temperature was carried out with cysteine added as an indicator for the MRI formation. The prepared MRIs were purified through high performance liquid chromatography (HPLC), their molecular weight and structures were determined via mass spectra and NMR. The flavor formation capacity of MRI derived from Xyl–Phe, Xyl–Phe mixture, and their MRPs was also evaluated through gas chromatography coupled with tandem mass spectrometry (GC-MS). The controlled formation of process flavors from MRIs could be used as potential alternative to the existing Maillard flavorings.
2. Experimental section
2.1. Materials
L-Phenylalanine, D-xylose, cysteine, sodium hydroxide, benzaldehyde (98%), benzeneacetaldehyde (98%) and n-alkanes (C6–C26) were purchased from Sigma-Aldrich Chemical Co. (Shanghai, China). 1,2-Dichlorobenzene (98%) was purchased from Macklin Biochemical Co. (Shanghai, China). LC-MS grade water, formic acid and acetonitrile were purchased from Sinopharm Chemical Reagent Co. Ltd (Shanghai, China).
2.2. Maillard reaction performed under stepwise increase of temperature
Model system (5 g) consisting of Xyl and Phe in a 2
:
1 relative molar ratio was thoroughly mixed with 80 mL deionized water. The solution pH was adjusted to pH 7.4 with NaOH (6 mol L−1). The solution was initially heated at 80 °C for different times (0–90 min) in the temperature and pressure resistant bottles. L-Cysteine (Cys) was then added (1% of the mixture) to the solution, and the pH was re-adjusted to pH 7.4. Moreover, the solution temperature was raised to 120 °C and held in oil bath for 110 min. The mixture was immediately cooled in ice water. The products of the Maillard reaction performed under stepwise increase of temperature (80 °C and 120 °C) were used for browning intensity measurements. The control group was prepared without the addition of Cys.
2.3. Browning intensity measurement
The browning intensity of MRPs was evaluated using a UV-vis spectrophotometer (UV-1800, Shimazu Co., Shanghai, China) by measuring the absorbance at 420 nm (A420).
The Maillard reaction products prepared under stepwise increase of temperature were diluted 10-folds before the absorbance measurement because of their excessive dark color, while the browning intensity of contrast group samples was measured without dilution due to their light color.
2.4. Preparation of MRI
The critical conditions of MRI formation were determined by measuring the browning intensity of the MRPs prepared through the Maillard reaction under stepwise increase of temperature. The critical conditions of MRI formation were similar to those of initial stage of the Maillard reaction (heating at 80 °C for 70 min) which MRPs had the minimum A420. Therefore, the MRI derived from Xyl and Phe (80 mL containing 5 g of Xyl and Phe in a 2
:
1 relative molar ratio at pH 7.4) was prepared under these conditions without the addition of Cys and the reaction was then terminated in ice water.
2.5. Purification and identification of MRI
2.5.1 Purification of MRI. The water in the MRI solution was removed by means of a rotary evaporator at 45 °C. Then the solid was mixed with anhydrous ethanol (500 mL). The undissolved components were filtered off and the solution was dried using rotary evaporation under vacuum (30 °C). Then the solid was dissolved in water and was applied to a column filled with Dowex 50WX4 ion exchange resin in H+-form.12 The column was first washed with water and then with ammonium hydroxide (0.2 mol L−1). The fractions containing the MRI were further purified using semi-preparative RP-HPLC. The C18 RP-HPLC (10 μm, 22 × 200 mm) column was used for the purification purpose. The elution rate was set at 3.0 mL min−1 by linear gradient from 2 to 100% acetonitrile/0.1% formic acid over 18 min.RP-HPLC was used for quantitative analysis of MRI. The X-Select C18 RP-HPLC column (3.5 μm, 4.6 × 150 mm) and photo diode-array detector 2996 (Waters, USA) were used for the sample analysis at 215 nm. The elution conditions were similar to that of semi-preparative RP-HPLC. The calibration for the MRI was prepared using the purified product.
The Xyl–Phe conversion into MRI (N-(1-deoxy-α-D-xylulos-1-yl)-phenylalanine) was calculated as the percentage of the measured molar concentration of N-(1-deoxy-α-D-xylulos-1-yl)-phenylalanine to the initial molar concentration of phenylalanine (y = 1.1039x − 2.8301, R2 = 0.9971).
2.5.2 UPLC-MS analysis of MRI. The UPLC-ESI-MS spectrum was obtained by mass spectrometry (Waters Synapt MALDI Q-TOF MS, USA) with positive ESI mode using Waters Acquity PDA detector. The ionization conditions were as followed: capillary voltage 3.5 kV, cone voltage 20 V, and extractor voltage 7 V. The source block temperature and the desolvation temperature were 100 °C and 400 °C, respectively. The cone gas flow was adjusted to 50 L h−1. The scanning of the sample was recorded over the range of m/z 20–1000 with a scan time of 1 s and an inter scan delay of 0.1 s. To facilitate the protonation of MRI required for the detection by ESI+-MS, water containing 0.1% formic acid was used as eluent. The CSH C18 (1.7 μm, 2.1 × 100 mm) column was used for Ultra Performance Liquid Chromatography (UPLC) analysis. Analytical UPLC conditions on LC-MS were as followed: injection volume was 1 μL, flow rate was 0.3 mL min−1, and UV detector was set at a range of 200–600 nm. The samples were analyzed by linear gradient from 2 to 100% MeOH/0.1% formic acid over 20 min by directly injecting the sample. Data were obtained using Mass Lynx software (version 4.1, Waters, Milford, MA, USA).
2.5.3 NMR analysis of MRI. After MRI purification by HPLC, the recovered products were analyzed by NMR. All NMR experiments were performed on a Bruker DRX 400 MHz spectrometer (Bruker Bio Spin, Germany) equipped with a 5 mm PABBO probe and operated at 25 °C (298 K). Data were obtained using MestReNova software (version 9.0.1, Mestrelab Research, Escondido, CA, USA).
N-(1-Deoxy-D-xylulos-1-yl)-L-phenylalanine 1. (400 MHz, D2O, ppm): δ = 7.39–7.25 (m, 5H, PhH), 4.42 (d, J = 8.3 Hz, 1H, H-3), 4.11–4.07 (m, 2H, H-5a + H-6), 4.02 (s, 2H, H-1), 3.70–3.55 (m, 2H, H-4 + H-5b), 3.10 (dd, J = 12.0 Hz, J = 4.0 Hz, 1H, H-8a), 3.06 (d, J = 12.0 Hz, 1H, H-8b); 13C NMR (100 MHz, D2O, ppm): 208.41 (C
O), 175.04–174.45 (COOH), 137.40–136.98 (C-9), 132.07–130.98 (C-10 + C-11 + C-12 + C-13 + C-14), 77.82 (C-3), 73.49 (C-4), 64.05 (C-5), 54.87 (C-6), 52.42 (C-1), 38.17 (C-8).
N-(α-D-Xylofuranos-1-yl)-L-phenylalanine 3′-α. (400 MHz, D2O, ppm): δ = 7.39–7.25 (m, 5H, PhH), 4.25 (m, J = 3.8 Hz, 1H, H-4), 4.10 (d, J = 4.4 Hz, 1H, H-3), 4.08 (t, J = 4.4 Hz, 1H, H-6), 3.98 (dd, J = 10.2 Hz, J = 2.7 Hz, 1H, H-5a), 3.86 (m, 1H, H-8a), 3.63 (dd, J = 10.2 Hz, J = 4.6 Hz, 1H, H-5b), 3.25 (d, J = 10.5 Hz, 1H, H-1a), 3.19 (m, 1H, H-8b), 3.07 (d, J = 10.5 Hz, 1H, H-1b); 13C NMR (100 MHz, D2O, ppm): 175.04–174.45 (COOH), 137.40–136.98 (C-9), 134.37–129.98 (C-10 + C-11 + C-12 + C-13 + C-14), 103.37–105.03 (C-2), 80.53 (C-3), 73.97 (C-4), 66.36 (C-5), 64.05 (C-6), 54.87 (C-1), 38.57–37.10 (C-8).
N-(β-D-Xylofuranos-1-yl)-L-phenylalanine 3′-β. 1H NMR (400 MHz, D2O, ppm): δ = 7.39–7.25 (m, 5H, Ph-H), 4.22 (m, 1H, H-4), 4.08 (m, 2H, H-3 + H-6), 3.86 (m, 2H, H-5a, H-8a), 3.62 (m, 1H, H-5b), 3.20 (d, J = 10.1 Hz, 1H, H-1a), 3.19 (m, 1H, H-8b), 3.18 (d, J = 10.1 Hz, 1H, H-1b); 13C NMR (100 MHz, D2O, ppm): 175.04–174.45 (COOH), 137.40–136.98 (C-9), 134.37–129.98 (C-10 + C-11 + C-12 + C-13 + C-14), 104.20 (C-2), 80.53 (C-3), 73.97 (C-4), 66.36 (C-5), 64.05 (C-6), 54.87 (C-1), 38.17 (C-8).
N-(α-D-Xylofuranos-1-yl)-L-phenylalanine 3-α. 1H NMR (400 MHz, D2O, ppm): δ = 7.39–7.25 (m, 5H, Ph-H), 4.17 (d, J = 4.6 Hz, 1H, H-1), 4.08 (m, 1H, H-6), 4.01–3.90 (m, 3H, H-2 + H-5), 3.86 (m, 1H, H-8a), 3.81 (m, 1H, H-3), 3.17–3.13 (m, 1H, H-4), 3.19 (m, 1H, H-8b); 13C NMR (100 MHz, D2O, ppm): 175.04–174.45 (COOH), 137.40–136.98 (C-9), 134.37–129.98 (C-10 + C-11 + C-12 + C-13 + C-14), 84.05 (C-4), 80.53 (C-1), 76.62 (C-2), 75.62 (C-3), 64.04 (C-5), 54.87 (C-6), 38.17 (C-8).
N-(β-D-Xylofuranos-1-yl)-L-phenylalanine 3-β. 1H NMR (400 MHz, D2O): δ = 7.39–7.25 (m, 5H, Ph-H), 4.08 (m, 1H, H-6), 4.01–3.96 (m, 1H, H-4), 3.92 (m, 1H, H-5a), 3.85 (m, 1H, H-5b), 3.86 (m, 1H, H-8a), 3.19 (m, 1H, H-8b), 3.11 (d, J = 4.6 Hz, 1H, H-1), 3.09–3.07 (m, 2H, H-3 + H-2); 13C NMR (100 MHz, D2O, ppm): 175.04–174.45 (COOH), 137.40–136.98 (C-9), 134.37–129.98 (C-10 + C-11 + C-12 + C-13 + C-14), 84.05 (C-4), 80.53 (C-1), 76.62 (C-2), 75.62 (C-3), 64.04 (C-5), 54.87 (C-6), 38.17 (C-8).
2.6. Evaluation of flavor formation capacity of MRI derived from Xyl–Phe, Xyl–Phe mixture, and their MRPs
2.6.1 Preparation of MRPs. Phe and Xyl were mixed in water in the molar ratio Xyl to Phe of 2
:
1 (Phe concentration was 4.5 mmol L−1). The mixture was heated at 130 °C for 150 min. The Maillard reaction could be conducted completely at such high temperature and long time.21–23
2.6.2 Thermal treatment of MRI and MRPs. The purified MRI was dissolved in water at a concentration of 4.5 mmol L−1 and pH was adjusted to 7.4. The MRI and MRPs solutions were heated at 100 °C for different time (0–120 min). The mixture of Xyl and Phe was simultaneously heated as control.
2.6.3 Headspace solid phase microextraction/gas chromatography/mass spectrometry (HS-SPME-GC/MS) analysis. The analysis of volatile compounds was performed using a gas chromatography/mass spectrometer (Finnigan Trace GC/MS, Finnigan, California, USA) equipped with a DB-Wax capillary column (30 m × 0.25 mm × 0.25 mm; J&W Scientific, Folsom, CA, USA). The volatile compounds were sampled with a carboxen/polydimethylsiloxane (75 mm) SPME fiber (Supelco, Bellefonte, PA, USA). Three grams of each MRPs sample were placed in a 15 mL vial, and an internal standard of 1,2-dichlorobenzene (50 mg in 1 mL of methanol) was added prior to trapping. The vial was sealed with a polytetrafluoroethylene/butyl septum and equilibrated at 50 °C for 30 min in the presence of the SPME fiber. After the equilibration time, the sample injection was conducted in the splitless mode at 250 °C for 3 min. The GC temperature was initially held at 40 °C for 3 min, then raised to 80 °C at 5 °C min−1, raised to 160 °C at 10 °C min−1, held at 160 °C for 0.5 min, raised to 175 °C at 2 °C min−1, raised to 230 °C at 10 °C min−1, and finally held at 230 °C for 7 min. Helium was used as carrier gas at a linear velocity of 1.8 mL min−1. Mass spectra were obtained in electron impact mode at an energy voltage of 70 eV and an emission current of 35 mA. The detector was set to scan the range m/z 35–450 at a rate of 4.45 scans per s.The identification of volatile compounds was carried out by comparing their mass spectra with the Wiley 6.0 (Wiley, New York, NY, USA), NIST (National Institute of Standards and Technology, Gaithersburg, MD, USA), Replib libraries and by comparing their Kovats indices (KIs) with those of standard compounds. Linear KIs of the compounds were calculated using a series of n-alkanes (C6–C26) injected under the same chromatographic conditions and compared with available literature data. The identified MRPs volatile compounds were quantified by GC/MS. Approximate quantities of the volatile compounds were estimated by comparing their peak areas with that of the internal standard, obtained from the total ion current chromatogram, assuming that the relative response factor was 1 and the recovery ratio was 100%. The quantitative formula was as follows:
where
Wi is the concentration (μg mL
−1) of compound
i,
Ai is the peak area of compound
i,
As is the peak area of internal standard,
ms is the mass of internal standard,
V is the volume of sample, and
f′ is a relative correction factor assumed to be 1.
Benzaldehyde (y = 3.82 × 106x − 0.038, R2 = 0.9993) and benzeneacetaldehyde (y = 5.50 × 105x − 0.082, R2 = 0.9991) in the sample were quantitated based on external calibration curve by comparing the peak areas with those of standard solutions containing known amounts of the pure compound.
2.7. Statistical analysis
The results were presented as mean values ± standard deviation from SPSS version 19.0 (IBM, Armonk, NY, USA). All measurements were done in triplicates and p < 0.05 was considered as significant.
3. Results and discussion
3.1. Determination of MRI formation condition
The MRPs were prepared by Maillard reaction performed under stepwise increase of temperature. The reaction was divided into two stages. During the first stage, the reaction was set at 80 °C for different reaction time (0–90 min), then, in the second stage, Cys was added and the temperature was increased to 120 °C for 110 min.
Results showed that A420 of the system without Cys addition increased with increasing reaction time (Fig. 1), indicating that melanoidins were generated during heating and their concentration increased with increasing reaction time. However, the addition of Cys changed the reaction course. A420 firstly decreased and reached the lowest level at 70 min heating time at 80 °C followed by heating for 110 min at 120 °C, then significantly increased afterward (Fig. 1). These results are in good agreement with previous findings of Huang et al.16 who reported that the color-inhibiting effect of Cys was due to its interaction with ARP. Since the browning inhibition resulted from the interaction between Cys and ARP, the lightest color might be the MRI formation indicator. The higher the concentration of MRI was, the more interaction between MRI and Cys occurred, and the lighter the color of the solution was observed. The total reaction time of 180 min (Fig. 1a) corresponds to the Cys addition time of 70 min (the reaction time of the first stage) (Fig. 1b), indicating that the MRI formation time was 70 min.
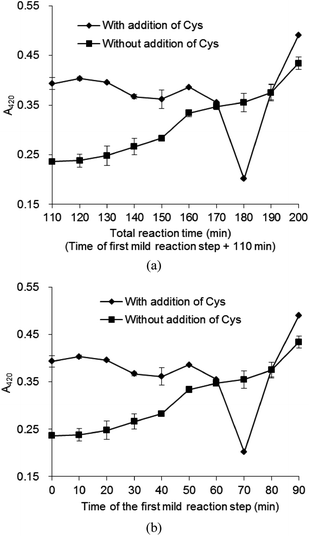 |
| Fig. 1 Effect of reaction time on browning (A420) of final Maillard reaction products (relative molar ratio of Xyl–Phe 2 : 1, initial pH 7.4, dosage of Cys: 1% of the mixture; first mild reaction step: 80 °C, 0–90 min; elevated temperature reaction step: 120 °C, 110 min. The control group was prepared without the addition of Cys). | |
MRI had not been abundantly formed before heating for 70 min at 80 °C, and the addition of Cys could not completely react with MRI, due to its competition with Phe. A part of Cys tended to react with Xyl to form Maillard reaction products,24 and was unable to inhibit the browning in high-temperature thermal reaction. Additionally, Cys could not reduce the brown color which had been formed before its addition. Although Cys was proved to be effective for browning inhibition, during the Maillard reaction performed under stepwise increase of temperature, but, when Cys was added with prolonged heating time over 70 min it could not inhibit the browning. When the heating time was extended at 80 °C, the MRI underwent enolization, deamination, dehydration, fragmentation and then generated a collection of sugar dehydration and fragmentation products. The reaction between these fragmentation products and added Cys generated melanoidins.25 Therefore, the addition of Cys after heating over 70 min at 80 °C could not inhibit the color formation, but promote the system browning. This might explain the drastic increase of A420 in the samples with Cys added after 70 min heating time at 80 °C during the first stage (Fig. 1). From the above results, it is clear that MRI derived from Phe and Xyl formation in the aqueous solution could be prepared by heating the solution at 80 °C for 70 min without Cys addition. The conversion of Xyl–Phe to MRI was 15%. The results indicated that the proposed method of Maillard reaction performed under stepwise increase of temperature could be a simple and useful method to determine the formation conditions of MRI in aqueous solution, without pure MRI as external standard. Thus, this method was the prerequisite step for the HPLC analysis for monitoring the concentration of the intermediate in the solution during the reaction.
3.2. Analysis of prepared MRI by UPLC-MS/MS
The MRI from reaction solution was separated by RP-HPLC and the purified MRI was analyzed using UPLC-MS/MS. The purity of the purified MRI was 98.26%. Evidence for the proposed MRI structures was provided through MS analysis of their elemental composition and the results are shown in Fig. 2. A single peak was shown in total ions chromatogram of purified MRI sample at retention time of 4.21 min (Fig. 2a), and its MS/MS spectrum was shown in Fig. 2b. The fragmentation pattern of the purified sample was in accordance with previous studies.26,27 MRI derived from Xyl and Phe is known as N-(1-deoxy-D-xylulos-1-yl)-phenylalanine (with molecular weight 297.1 Da) whose NH group tend to combine with a proton in ESI-MS/MS measurement.9,26,27 The parent ion with m/z = 298 was identified in spectra. A successive loss of 2 molecules of water from N-(1-deoxy-D-xylulos-1-yl)-phenylalanine (parent ion with m/z = 298) was observed, which corresponded to the positive single-charged fragments with m/z 280 and 262 respectively. In the mass spectra, the ion [M + H − H2O] was the most abundant peak (Fig. 2b). These results are in accordance with the findings of Davidek et al.12 The loss of the formic acid molecule was followed by fragmentation of a successive loss of 2 molecules of water from the parent ion with m/z 298 yielded ions at m/z 252, m/z 234 and m/z 216, respectively. The cleavage of ketosyl residue yielded ion at m/z 166, while cleavage of furan ring generated low abundant ion at m/z 178. The detailed fragmentation was shown in Scheme 1 based on the structural feature of N-(1-deoxy-D-xylulos-1-yl)-phenylalanine and the mass spectrometry information. According to the abundance of fragments (Fig. 2b), the dehydrated ions at m/z 280 and m/z 234 were the dominant ones, indicating the loss of molecules of water from the purified sample. These results are consistent with previous findings.9,12,26,27 The molecular mass and proposed formula were 297.1 Da and C14H19O6N, respectively.
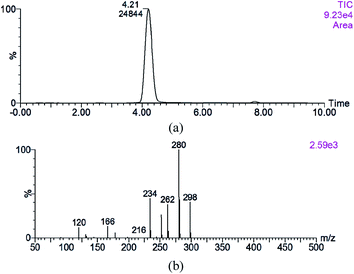 |
| Fig. 2 Total ions chromatogram (a) and LC-MS/MS spectrum (b) of purified MRI. | |
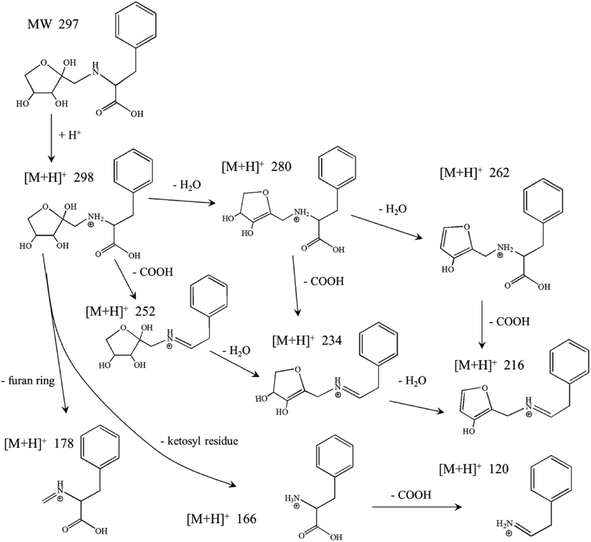 |
| Scheme 1 N-(1-Deoxy-D-xylulos-1-yl)-phenylalanine fragmentation ([M + H]+ 298, 280, 262, 234) in the LC-MS/MS spectrum. | |
3.3. Analysis of prepared MRI by NMR
The NMR spectroscopy is a powerful tool to investigate the structure of molecules. The NMR data of the purified sample confirmed signals in the expected regions. Five major sets of sugar resonances attributable to the 1-deoxy-D-xylulosyl moiety were observed. The chemical shift δ of 104.2 and 52.41 ppm in 13C NMR, and 3.28–3.21 ppm in 1H NMR showed the presence of 3′-α and 3′-β (Scheme 2). The formation of the intra molecular hydrogen bonds led to a five-membered ring that blocked the pathway of 4-OH approaching the carbonyl group from the upside, and thus the rearrangement gave the tautomers 3′-α and 3′-β.28 The chemical shift δ of 80.53 and 64.04 ppm in 13C NMR, and 3.92, 3.85 and 3.14 ppm in 1H NMR showed that 3-α and 3-β (Scheme 2) probably existed in the prepared MRI. These results are consistent with the findings of Zhao et al.29 The chemical shift δ of 208.41 in 13C NMR indicated the chained keto form of N-(1-deoxy-D-xylulos-1-yl)-phenylalanine. In chained keto molecule of N-(1-deoxy-D-xylulos-1-yl)-phenylalanine, intra molecular hydrogen bonds between 2-OH, 3-OH, and oxygen of carbonyl group of MRI keto-form, were possibly formed in aqueous solution. Similar results were previously reported based on 13C NMR spectroscopic investigations,28,30 revealing that the Amadori ketoses exists in aqueous solution as an equilibrium of different conformers including α-furanose and β-furanose, ring and open ring.9,30 A tautomeric equilibrium involving ring opening, keto–enol tautomerism, and ring closure was proposed (Scheme 2). The tautomer 3-α and 3-β named as N-(α-D-xylulos-1-yl)-phenylalanine and N-(β-D-xylulos-1-yl)-phenylalanine were from the rearrangement of the labile enol form that was not observed in NMR spectra (Fig. S2†).
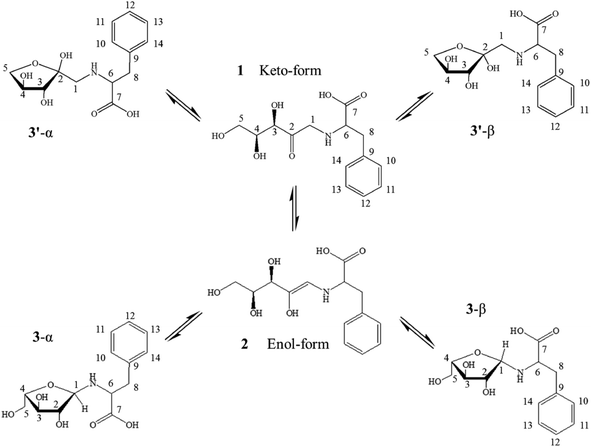 |
| Scheme 2 Supposed isomerization of N-(1-deoxy-D-xylulos-1-yl)-phenylalanine (N-(1-deoxy-D-xylulos-1-yl)-L-phenylalanine 1, N-(α-D-xylofuranos-1-yl)-L-phenylalanine 3′-α, N-(β-D-xylofuranos-1-yl)-L-phenylalanine 3′-β, N-(α-D-xylofuranos-1-yl)-L-phenylalanine 3-α, N-(β-D-xylofuranos-1-yl)-L-phenylalanine 3-β). | |
3.4. Preparation of MRI in aqueous solution and its thermal decomposition to form flavor compounds
The MRI was prepared in aqueous solution using the following conditions: Xyl to Phe relative molar ratio 2
:
1, initial pH 7.4, solution concentration 58.8 mg mL−1 and reaction time of 70 min at 80 °C. Recent studies showed that the MRPs flavoring could usually be prepared at higher temperature above 100 °C,31–33 however, the high temperature facilitated the development of more labile flavor components.5,34 It remarkably increased the formation of meaty aroma and some desirable flavor precursors, which are known as thermal process flavoring products.32,35,36 During this study, the proposed method for preparing MRI derived from Xyl–Phe was combined with the conventional technique for the preparation of thermal process flavoring, but using much lower temperature for Maillard reaction.
The MRI and MRPs were heated at 100 °C, respectively, and the volatile compounds in the final products were measured using GC-MS. The major volatile compounds in MRPs before the heat treatment were shown in Table 1. Strong flower-like scent was felt from the MRPs, without any off-flavor. The results of changes in MRI concentration and total volatile compounds during thermal reaction are shown in Fig. 3 and 4. During the thermal reaction, 1,2- and 2,3-enolizations of ARP initiate β-elimination reactions in acidic or base catalyzed conditions, which eventually lead to the formation of reactive intermediates.2,37,38 As the reaction time prolonged, the concentration of MRI was decreased (Fig. 3a) while the total concentration of volatile compounds was increased (Fig. 4). However, MRI solution nearly showed no volatile compounds before the heat treatment (Fig. 4), indicating that the fresh flavor was generated during heat treatment of MRI. Results showed that, prolonging heating after 80 min, the concentration of volatile compounds in MRI drastically declined, confirming the occurrence of aldol and aldehyde–amine condensations, which led to the formation of melanoidins.2 These results suggested that the fresh flavor from Xyl and Phe could be produced at 100 °C for no more than 80 min reaction time (Fig. 4). The increasing concentration of volatile compounds from 0 to 1.68 μg mL−1 was observed in the heated Xyl–Phe for 120 min, indicating that fresh flavor could also be formed in a mixture of sugar and amino acid. However, both the concentration of volatile compounds and their generation rate in heated Xyl–Phe were much lower than that in MRI solution. These results revealed that the formation of fresh flavor was higher using MRI than using Xyl–Phe during heat treatment. As shown in Fig. 3b, a drastic increase in A420 of MRI solution was observed compared to that of Xyl–Phe during the heat treatment. Thus, the reactivity of Xyl–Phe in Maillard reaction was inferior to that of MRI. Compared with MRI and Xyl–Phe, MRPs showed an inverse trend of volatile compounds concentration during the heat treatment (Fig. 4). The volatile compounds in MRPs decreased during the heat treatment, and this might be due to the volatilization of flavor compounds and polymerization reaction to generate melanoidins in the final stage of Maillard reaction. MRI showed a great advantage of flavor formation compared to MRPs. The concentration of volatile compounds in heated MRI was higher than that in the heated MRPs after approximately 30 min thermal reaction. After heat treatment at 100 °C for 80 min, the total concentration of volatile compounds generated from MRI was 9.44 and 8.77 times higher than that from MRPs and Xyl–Phe, respectively. The major volatile compounds generated during 80 min thermal reaction are shown in Table 1. Acetone, 2,5-dimethyl-pyrazine, nonanal, furfural, 2-ethyl-1-hexanol, 1-(2-furanyl)-ethanone, benzaldehyde, 2-acetyl-5-methylfuran, 2,4-dimethyl-benzaldehyde 3-phenylfuran, 2,3-dimethyl-2-cyclopenten-1-one were reported as important Maillard reaction products.39–42 Benzaldehyde and benzeneacetaldehyde were observed as the major volatile compounds in all the four samples, and the concentration of both increased during the heat treatment of MRI (Table 2). After the heat treatment of MRI, the concentration percentage of benzaldehyde and benzeneacetaldehyde were higher than that of the heated MRPs (Table 2). The flavor profile of MRPs had been changed during its heat treatment and the flower fragrance was faded. Thus, the MRI derived from Xyl–Phe could be applied as a flower-like flavor precursors and the flavor could be highlighted more during the heat treatment of MRI rather than that of MRPs.
Table 1 Major volatile compounds in the products of heated Phe–Fru, MRPs and MRI
Compound name |
Kovats RIb |
Observed RI |
Identc |
Relative content of volatile compounds (μg L−1) |
MRPsd (non-heated) |
MRPsd |
MRId |
Xyl–Phed |
http://www.flavornet.org. NIST 2.0. MS, identified by NIST mass spectral database; RI, agreed with the retention indices published in literatures; Std, compounds were identified by standard (concentration 4.5 mmol L−1, pH 7.4, 100 °C, 0–120 min). The total ion chromatograms for the chemical composition of these samples are presented in ESI data. |
Acetone |
813a |
797 |
MS, RI |
32.05 ± 2.37 |
29.05 ± 1.25 |
ND |
ND |
Styrene |
1254 |
1242 |
MS, RI |
1.61 ± 0.09 |
1.90 ± 0.21 |
159.78 ± 8.96 |
ND |
Octanal |
1275 |
1276 |
MS, RI |
2.61 ± 0.55 |
1.29 ± 0.12 |
12.61 ± 1.18 |
ND |
2,5-Dimethyl-pyrazine |
1348 |
1332 |
MS, RI |
6.34 ± 0.32 |
4.14 ± 0.14 |
ND |
ND |
2-Methyl-2-cyclopenten-1-one |
1357 |
1361 |
MS, RI |
6.05 ± 0.57 |
4.18 ± 0.66 |
ND |
ND |
Nonanal |
1390 |
1382 |
MS, RI |
13.15 ± 1.11 |
4.49 ± 0.28 |
59.00 ± 2.45 |
ND |
Furfural |
1439 |
1456 |
MS, RI |
40.07 ± 1.97 |
14.68 ± 1.03 |
25.48 ± 1.74 |
ND |
2-Ethyl-1-hexanol |
1488 |
1490 |
MS, RI |
11.52 ± 0.82 |
4.77 ± 0.15 |
71.29 ± 2.96 |
ND |
1-(2-Furanyl)-ethanone |
1512 |
1497 |
MS, RI |
15.67 ± 0.95 |
5.20 ± 0.09 |
18.88 ± 0.22 |
ND |
Benzaldehyde |
1508 |
1512 |
MS, RI, Std |
1681.32 ± 14.84 |
417.51 ± 7.21 |
7280.44 ± 40.03 |
222.02 ± 3.89 |
2-Acetyl-5-methylfuran |
1593 |
1595 |
MS, RI, |
60.63 ± 2.61 |
3.12 ± 0.42 |
34.46 ± 2.13 |
ND |
Benzeneacetaldehyde |
1648 |
1635 |
MS, RI, Std |
43.48 ± 2.55 |
11.08 ± 0.87 |
1523.41 ± 10.94 |
5.90 ± 0.12 |
Acetophenone |
1593 |
1595 |
MS, RI |
326.07 ± 4.88 |
57.65 ± 3.00 |
353.30 ± 9.17 |
ND |
2,4-Dimethyl-benzaldehyde |
1710 |
1722 |
MS, RI |
25.46 ± 2.59 |
5.82 ± 0.13 |
131.82 ± 4.02 |
17.69 ± 1.33 |
3-Phenylfuran |
1863 |
1865 |
MS, RI |
14.31 ± 1.99 |
28.21 ± 2.03 |
24.64 ± 1.61 |
ND |
Benzyl alcohol |
2007 |
2026 |
MS, RI |
17.29 ± 1.05 |
4.62 ± 0.07 |
24.07 ± 1.97 |
ND |
2-Butanone |
881 |
862 |
MS, RI |
9.92 ± 0.06 |
ND |
ND |
ND |
Undecane |
1100 |
1088 |
MS, RI |
2.21 ± 0.01 |
ND |
ND |
ND |
2,3-Dimethyl-2-cyclopenten-1-one |
1535 |
1533 |
MS, RI |
5.41 ± 0.04 |
ND |
ND |
ND |
1-Octanol |
1561 |
1558 |
MS, RI |
50.70 ± 1.57 |
ND |
423.85 ± 9.22 |
ND |
6-Methyl-5-hepten-2-one |
1339 |
1330 |
RI |
ND |
ND |
11.86 ± 0.93 |
ND |
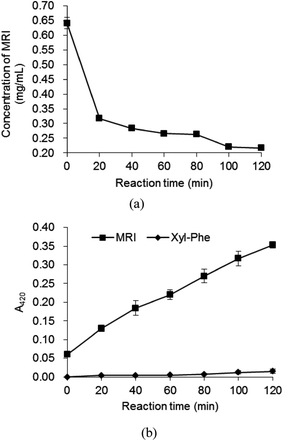 |
| Fig. 3 Time course for concentration (a) and A420 (b) of heated MRI at 100 °C (concentration 4.5 mmol L−1, pH 7.4, 100 °C, 0–120 min). | |
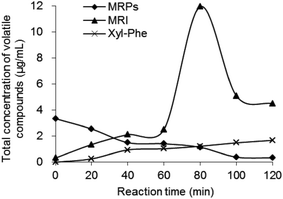 |
| Fig. 4 Time course for total concentration of volatile compounds derived from MRI, MRPs and Xyl–Phe during the reaction at 100 °C (concentration 4.5 mmol L−1, pH 7.4, 100 °C, 0–120 min). | |
Table 2 The concentration of benzaldehyde and benzeneacetaldehyde of Phe–Fru, MRPs and MRI solution
Samples |
Benzeneacetaldehyde (μg L−1) |
Benzaldehyde (μg L−1) |
Before heating |
After heating |
Before heating |
After heating |
MRPs |
218.18 ± 12.80 |
164.19 ± 12.89 |
1217.11 ± 10.74 |
892.38 ± 15.41 |
MRI |
ND |
1318.34 ± 9.47 |
ND |
909.00 ± 5.00 |
Xyl–Phe |
ND |
675.87 ± 13.75 |
ND |
189.25 ± 3.85 |
MRI was decomposed when it was heated to form reductones, aldols and aldehydes.43 Besides, the hydrolysis of MRI may produce sugar degradation products together with regenerating the amino acid.35 It might also degrade without hydrolysis. Therefore, the degradation is quite complex and depends on the conditions and environment. The reverse of the Amadori rearrangement and hydrolysis of Schiff base may regenerate the sugar and amino acid.37,38
The potential of MRI to undergo Maillard reaction and achieve controlled formation of fresh process flavors could be confirmed and it might provide achievability and pleasure of cooking for consumers.
4. Conclusions
The MRPs were prepared by Maillard reaction performed under stepwise increase of temperature. The formation conditions of MRI generated from Xyl and Phe were determined as heating at 80 °C for 70 min. The prepared MRI derived from Xyl–Phe model system was purified by HPLC. The obtained compound was characterized and confirmed by ESI-mass spectra and NMR. Its molecular mass and formula were determined as 297.1 Da and C14H19O6N, respectively. During the thermal reaction of MRI, the total volatile content significantly increased compared with MRPs and the mixture of Xyl–Phe. The controlled MRI fresh flavors were formed and could be a great potential substitute of MRPs flavors.
Conflicts of interest
The authors declare no competing financial interest and there are no conflicts to declare.
Funding
This work was financially supported by projects of the National Natural Science Foundation of China [grant numbers 31671826], the National Key R&D Program of China [grant numbers 2017YFD0400105] and “Collaborative innovation center of food safety and quality control in Jiangsu Province”.
Abbreviations used
ARP | Amadori rearrangement products |
MRPs | Maillard reaction products |
MRI | Maillard reaction intermediate |
Xyl | Xylose |
Phe | Phenylalanine |
Cys | Cysteine |
RP-HPLC | Reversed phase high performance liquid chromatography |
UPLC | Ultra performance liquid chromatography |
MS | Mass spectrometry |
NMR | Nuclear magnetic resonance |
HS-SPME | Headspace solid phase microextraction |
GC | Gas chromatography |
Acknowledgements
The research was supported by the National Natural Science Foundation of China 31671826, the National Key R&D Program of China 2017YFD0400105, and “Collaborative innovation center of food safety and quality control in Jiangsu Province”.
References
- X. Yu, M. Zhao, J. Hu and S. Zeng, Formation and antioxidant activity of volatile compounds produced by heating glucose with tyrosine/histidine in water-ethanol and water-glycerol media, Food Chem., 2012, 133, 1394–1401 CrossRef CAS.
- A. José, H. Rufián and P. Silvia, Maillard Reaction, in Encyclopedia of Food and Health, ed. C. Benjamin, M. F. Paul and T. Fidel, Academic Press, Cambridge, 2016, pp. 593–600 Search PubMed.
- P. Mariela, J. R. Gustavo and E. L. Cecilia, In vivo effects of Maillard reaction products derived from biscuits, Food Chem., 2016, 196, 204–210 CrossRef PubMed.
- I. K. Muhammad, J. Cheorun and R. T. Muhammad, Meat flavor precursors and factors influencing flavor precursors – a systematic review, Meat Sci., 2015, 110, 278–284 CrossRef PubMed.
- J. K. Parker, Thermal Generation or Aroma, in Flavour Development, Analysis and Perception in Food and Beverages, ed. J. K. Parker, S. Elmore and L. Methven, Wood Head Publishing Series, Cambridge, 2015, pp. 151–185 Search PubMed.
- J. Deblander, S. Aeken, A. Adams, N. Kimpe and K. A. Tehrani, New short and general synthesis of three key Maillard flavour compounds: 2-Acetyl-1-pyrroline, 6-acetyl-1,2,3,4-tetrahydropyridine and 5-acetyl-2,3-dihydro-4H-1,4-thiazine, Food Chem., 2015, 168, 327–331 CrossRef CAS PubMed.
- A. D. Troise, C. C. Berton-Carabin and V. Fogliano, Amadori products formation in emulsified systems, Food Chem., 2016, 199, 51–58 CrossRef CAS PubMed.
- C. Perez-Locas and V. A. Yaylayan, The Maillard reaction and food quality deterioration, Chemical Deterioration and Physical Instability of Food and Beverages, L. H. Skibsted, J. Risbo and M. L. Andersen, Wood Head Publishing Series, Cambridge, 2010, pp. 70–94 Search PubMed.
- V. H. Nanishankar, M. S. Sudhanva and U. Sushma, ZnCl2-mediated practical protocol for the synthesis of Amadori ketoses, Food Chem., 2014, 158, 340–344 CrossRef PubMed.
- W. M. Coleman and H. L. Chung, Pyrolysis GC-MS analysis of Amadori compounds derived from selected amino acids and glucose, J. Anal. Appl. Pyrolysis, 2002, 62, 215–223 CrossRef CAS.
- C. Cerny, F. Fitzpatrick and J. Ferreira, Effect of salt and sucrose addition on the formation of the Amadori compound from methionine and glucose at 40 °C, Food Chem., 2011, 125, 973–977 CrossRef CAS.
- T. Davidek, K. Kraehenbuehl, S. Devaud, F. Robert and I. Blank, Analysis of Amadori Compounds by High-Performance Cation Exchange Chromatography Coupled to Tandem Mass Spectrometry, Anal. Chem., 2005, 77(1), 140–147 CrossRef CAS PubMed.
- V. V. Mossine and T. P. Mawhinney, N-(1-Deoxy-D-fructos-1-yl)-L-histidine (“D-Fructose-L-histidine”): a Potent Copper Chelator from Tomato Powder, J. Agric. Food Chem., 2007, 55, 10373–10381 CrossRef CAS PubMed.
- I. Jerić and Š. Horvat, Screening for glucose-triggered modifications of glutathione, J. Pept. Sci., 2009, 15(8), 540–547 CrossRef PubMed.
- T. E. Gloe, I. Stamer, C. Hojnik, T. M. Wrodnigg and T. K. Lindhorst, Are D-manno-configured Amadori products ligands of the bacterial lectin FimH, Beilstein J. Org. Chem., 2015, 11, 1096–1104 CrossRef CAS PubMed.
- M. G. Huang, X. M. Zhang, K. Eric, S. Abbas, K. Hayat, P. Liu and S. Q. Xia, Inhibiting the color formation by gradient temperature-elevating Maillard reaction of soybean peptide-xylose system based on interaction of L-cysteine and Amadori compounds, J. Pept. Sci., 2012, 18, 342–349 CrossRef CAS PubMed.
- M. Huang, X. Zhang and K. Eric, Comparation sensory characteristic, non-volatile compounds, volatile compounds and antioxidant activity of MRPs by novel gradient temperature-elevating and traditional isothermal methods, J. Food Sci. Technol., 2015, 52(2), 858–866 CrossRef CAS PubMed.
- Z. Hao, C.-Y. Lu, B. Xiao, N. Weng, B. Parker, M. Knapp and C.-T. Ho, Separation of amino acids, peptides and corresponding Amadori compounds on a silica column at elevated temperature, J. Chromatogr. A, 2007, 1147, 165–171 CrossRef CAS PubMed.
- S. Seisonen, E. Kivima and K. Vene, Characterization of the aroma profiles of different honeys and corresponding flowers using solid-phase micro extraction and gas chromatography-mass spectrometry/olfactometry, Food Chem., 2015, 169, 34–40 CrossRef CAS PubMed.
- S. Sharma and R. Kumar, Effect of temperature and storage duration of flowers on essential oil content and composition of damask rose (Rosa × damascena Mill.) under western Himalayas, J. Appl. Res. Med. Aromat. Plants, 2016, 3, 10–17 Search PubMed.
- E. Bertrand, X. Meyer, E. Machado-Maturana, J.-L. Berdagué and A. Kondjoyan, Modelling the Maillard reaction during the cooking of a model cheese, Food Chem., 2015, 184, 229–237 CrossRef CAS PubMed.
- M.-J. Oh, Y. Kim, S. H. Lee, K.-W. Lee and H.-Y. Park, Prediction of CML contents in the Maillard reaction products for casein-monosaccharides model, Food Chem. DOI:10.1016/j.foodchem.2017.07.141.
- L. Sun and Y. Zhuang, Characterization of the Maillard Reaction of Enzyme-Hydrolyzed Wheat Protein Producing Meaty Aromas, Food Bioprocess Technol., 2012, 5, 1287–1294 CrossRef CAS.
- C. Cerny and S. A. Firmenich, The role of sulfur chemistry in thermal generation of aroma, in Flavour Development, Analysis and Perception in Food and Beverages, ed. J. K. Parker, S. Elmore and L. Methven, Wood Head Publishing Series, Cambridge, 2015, pp. 187–210 Search PubMed.
- D. S. Mottram, The Maillard Reaction: Source of Flavour in Thermally Processed Foods, in Flavours and Fragrances, ed. R. G. Berger, Springer-Verlag, Berlin, 2007, pp. 269–283 Search PubMed.
- M. D. Linetsky, E. V. Shipova, R. D. Legrand and O. O. Argirov, Glucose-derived Amadori compounds of glutathione, Biochim. Biophys. Acta, 2005, 1724, 181–193 CrossRef CAS PubMed.
- P. Stefanowicz, K. Kapczynska, A. Kluczykand and Z. Szewczuk, A new procedure for the synthesis of peptide-derived Amadori products on a solid support, Tetrahedron Lett., 2007, 48, 967–969 CrossRef CAS.
- A. Jakas, A. Katić, N. Bionda and Š. Horvat, Glycation of a lysine-containing tetrapeptide by D-glucose and D-fructose – influence of different reaction conditions on the formation of Amadori/Heyns products, Carbohydr. Res., 2008, 343, 2475–2480 CrossRef CAS PubMed.
- M. Zhao, Y. Wang, C. Huo, C. Li, X. Zhang, L. Peng and S. Peng, Stereo selective synthesis of novel N-(a-L-arabinofuranos-1-yl)-L-amino acids, Tetrahedron: Asymmetry, 2009, 20, 247–258 CrossRef CAS.
- M. Kaufmann, P. M. Meissner, D. Pelke, C. Mügge and L. W. Kroh, Structure-reactivity relationship of Amadori rearrangement products compared to related ketoses, Carbohydr. Res., 2016, 428, 87–99 CrossRef CAS PubMed.
- E. Karangwa, X. Zhang, N. Murekatete, K. Masamba, V. R. Linda, A. Shabbar, Y. Zhang, E. Duhoranimana, B. Muhoza and S. Song, Effect of substrate type on sensory characteristics and antioxidant capacity of sunflower Maillard reaction products, Eur. Food Res. Technol., 2015, 240(5), 939–960 CrossRef CAS.
- J. Liu, M. Liu, C. He, H. Song and F. Chen, Effect of thermal treatment on the flavor generation from Maillard reaction of xylose and chicken peptide, LWT–Food Sci. Technol., 2015, 64(1), 316–325 CrossRef CAS.
- S. Song, S. Li, L. Fan, K. Hayat, Z. Xiao, L. Chen and Q. Tang, A novel method for beef bone protein extraction by lipase-pretreatment and its application in the Maillard reaction, Food Chem., 2016, 208(1), 81–88 CrossRef CAS PubMed.
- D. P. Balagiannis, Predicting aroma formation with kinetic models, in Flavour Development, Analysis and Perception in Food and Beverages, ed. J. K. Parker, S. Elmore and L. Methven, Wood Head Publishing Series, Cambridge, 2015, pp. 211–233 Search PubMed.
- A. J. Trevisan, A. L. D. De, G. R. Sampaio, R. A. Soares and D. H. Markowicz Bastos, Influence of home cooking conditions on Maillard reaction products in beef, Food Chem., 2016, 196, 161–169 CrossRef CAS PubMed.
- A. Michalska, J. Honke, G. Łysiak and W. Andlauer, Effect of drying parameters on the formation of early and intermediate stage products of the Maillard reaction in different plum (Prunus domestica L.) cultivars, LWT–Food Sci. Technol., 2016, 65, 932–938 CrossRef CAS.
- S. I. F. S. Martins, A. T. M. Marcelis and M. A. J. S. Boekel, Kinetic modelling of Amadori N-(1-deoxy-D-fructos-1-yl)-glycine degradation pathways. Part I – Reaction mechanism, Carbohydr. Res., 2003, 338, 1651–1663 CrossRef CAS PubMed.
- T. Kocadaǧlı and V. Gökmen, Multiresponse kinetic modelling of Maillard reaction and caramelisation in a heated glucose/wheat flour system, Food Chem., 2016, 211, 892–902 CrossRef PubMed.
- H. Yu, M. Z. M. Keh, Y.-X. Seow, P. K. C. Ong and W. Zhou, Kinetic Study of High-Intensity Ultrasound-Assisted Maillard Reaction in a Model System of D-Glucose and L-Methionine, Food Bioprocess Technol., 2017 DOI:10.1007/s11947-017-1971-7.
- C. Cao, J. Xie, L. Hou, J. Zhao, F. Chen, Q. Xiao, M. Zhao and M. Fan, Effect of glycine on reaction of cysteine-xylose: insights on initial Maillard stage intermediates to develop meat flavor, Food Res. Int., 2017 DOI:10.1016/j.foodres.2017.06.012.
- X. Yu, M. Zhao, F. Liu, S. Zeng and J. Hu, Antioxidants in volatile Maillard reaction products Identification and interaction, LWT–Food Sci. Technol., 2013, 53, 22–28 CrossRef CAS.
- G. Su, L. Zheng, C. Cui, B. Yang, J. Ren and M. Zhao, Characterization of antioxidant activity and volatile compounds of Maillard reaction products derived from different peptide fractions of peanut hydrolysate, Food Res. Int., 2011, 44, 3250–3258 CrossRef CAS.
- H. Nursten, The Chemistry of Nonenzymic Browning, The Maillard Reaction, Royal Society of Chemistry, Cambridge, 2005, pp. 5–26 Search PubMed.
Footnote |
† Electronic supplementary information (ESI) available. See DOI: 10.1039/c7ra09355a |
|
This journal is © The Royal Society of Chemistry 2017 |