DOI:
10.1039/C7RA08987B
(Paper)
RSC Adv., 2017,
7, 49675-49683
CNTs based improved chlorine sensor from non-covalently anchored multi-walled carbon nanotubes with hexa-decafluorinated cobalt phthalocyanines
Received
14th August 2017
, Accepted 10th October 2017
First published on 25th October 2017
Abstract
To study the effect of synergetic interactions between metal-phthalocyanine and carbon nanotubes for gas sensing characteristics of carbon nanotubes, we synthesized a hybrid of cobalt(II)-1,2,3,4,8,9,10,11,15,16,17,18,22,23,24,25-hexa-decafluoro-29H,31H-phthalocyanine/multi-walled carbon nanotubes (F16CoPc/MWCNTs–COOH). The as-prepared hybrid was characterized through spectroscopic (FT-IR, UV-vis and Raman), electron microscopic (TEM and FE-SEM) and TGA investigations that confirmed the successful non-covalent anchoring of F16CoPc onto MWCNTs–COOH through π–π stacking interactions. Further, a highly reversible, reproducible, sensitive and Cl2 selective chemiresistive sensor was fabricated using F16CoPc/MWCNTs–COOH hybrid, which exhibited a sensitivity of ∼63% for 2 ppm of Cl2 and a limit of detection as low as 0.05 ppb. A plausible gas sensing mechanism for improved gas sensing characteristics of F16CoPc/MWCNTs–COOH sensor towards Cl2 was explained using Raman, X-ray photoelectron spectroscopy (XPS) and electrochemical impedance spectroscopy (EIS) studies. Herein, cobalt metal ion is found to play an important role in enhancing gas sensing characteristics of the fabricated sensor.
1. Introduction
Concern for environmental pollution, medical diagnosis, automobiles and industrial emission monitoring has led to the development of sensors that can detect chemical species in the atmosphere.1,2 Thus, it is of great concern for the development of sensors that are rapid, simple and consume low power. In this direction, nanostructured CNTs based chemiresistive sensors stand out due to their unique and interesting properties, such as large specific surface area, gas adsorption capability and high electrical conductivity.3 Chemiresistive sensors are comparatively rapid, cheap and simple to use having low power consumption and temperature requirements4 in comparison to analytical procedure based on high-performance liquid chromatography-mass spectrometry (HPLC-MS) or gas chromatography-mass spectrometry (GC-MS), both of which are quite laborious, expensive and cumbersome.5 A number of gases such as NO2, NH3, NO, CO and Cl2 have been tested using CNTs based chemiresistive sensors.6–9 However, poor sensing characteristics (lack of selectivity, irreversibility and slow response/recovery time) due to low charge transfer between the pristine CNTs and gas molecules hamper commercialization of CNTs based sensors. Further, a variety of methods have been developed for making CNT networks, such as direct growth on substrates, dispersions, electrophoresis and Langmuir–Blodgett.5 However, limitations to these methods are the requirement of expensive and specific equipment for mounting CNTs directly on substrates. The primary hurdles in solution-based methods are poor solubility of CNTs in solvents and very less stability of dispersions of CNTs. To improve the solubility and gas sensing characteristics of CNTs based sensors, hybrids of CNTs with materials, such as metal oxides, noble metal nanoparticles and organic semiconductors, have been explored.10–13
The CNTs based hybrid gas sensors have attracted extensive attention due to synergic effects of two or more components through the strong electron transfer interaction.14
Hsu et al.15 have monitored the residual chlorine concentration using phenyl-capped aniline tetramer (PCAT) doped with SWCNTs in drinking water with detection range of 0.06–60 mg l−1. Muñoz et al.16 demonstrated the capability of modified MWCNTs nanocomposite sensors with CuO nanoparticles for the sensitive sensing of free chlorine. Li et al.17 have reported the Cl2 selective hybrid of single-wall carbon nanotubes (SWCNTs) with polymers such as chlorosulfonated polyethylene and hydroxypropyl cellulose as chemiresistive sensors but were having primary issues of response and recovery time with these sensors. Furthermore, Gohier et al.18 have reported MWCNTs as well as nitrogen-doped and polyethyleneimine (PEI) functionalized MWCNTs based room temperature Cl2 sensor that can detect a Cl2 concentration down to 27 ppb, but sensor recovery could only be possible by heating it up to 75 °C for 60 min. In spite of various efforts, the characteristics such as gas response, selectivity and stability are still not ideal.
These challenging issues of improving carbon nanotube sensing characteristics have initiated to explore hybrids of carbon nanotubes with metallo-phthalocyanines (MPcs). MPcs are extensively used as excellent sensing materials as they exhibit remarkable gas sensing characteristics due to their fascinating chemical and physical properties and good thermal and chemical stability.19 Moreover, solubility of hybrids is better in comparison with pristine CNTs, which make them more attractive for different applications.20 The simple solution processing methods such as solution assembly, dip dropping and spin coating can be used for deposition of hybrid films. Recently, we have fabricated ppb level chemiresistive Cl2 sensor using substituted phthalocyanine and CNTs based hybrids.21–24 Further, the central metal ion, substitution of functional groups on phthalocyanine ring, influences the morphology and remarkably tunes the gas sensing characteristics of phthalocyanine molecules.25
In the present study, multi-walled carbon nanotubes (MWCNTs–COOH) are non-covalently functionalized with Co(II)-1,2,3,4,8,9,10,11,15,16,17,18,24,25-hexa-decafluoro-29H,31H-phthalocyanine (F16CoPc) for fabrication of ppb level Cl2 selective chemiresistive sensor. In comparison to our earlier reports, F16CoPc molecule proved to be a better material for CNTs functionalization because of tuneable electronic properties due to shorter π–π stacking inter-molecular distance (∼3.23 Å).26,27 Moreover, molecular functionalization causes molecular orbitals to get closer to the Fermi level that leads to an increase in its ionization potential and electron affinity.28
2. Experimental
The commercially available MWCNTs and F16CoPc samples were procured from Sigma-Aldrich Pvt. Ltd., India. Furthermore, acidification of MWCNTs bearing an acidic group (–COOH) was carried out according to the multi-step procedures developed by Smalley and co-workers.29 F16CoPc/MWCNTs–COOH hybrid was synthesized using solution assembly method through π–π stacking between MWCNTs–COOH and F16CoPc in DMF. Herein, varied amount of F16CoPc (0.1 to 0.5 wt%) was dissolved in 5 ml of dimethylformamide (DMF) and subjected to ultra-sonication, to form F16CoPc solution in DMF. This solution was then added dropwise to MWCNTs–COOH suspensions in DMF and the resulting mixtures were sonicated at room temperature (25 °C) for 3 h and subsequently stirred in dark for 6 h at 80 °C. The mixture was filtered through a PTEF filter (0.22 μm, Millipore) and washed thoroughly with DMF to remove excess of F16CoPc derivative, subsequently rinsed with ethanol for several times and finally dried to acquire desired, F16CoPc/MWCNTs–COOH hybrid.
Fourier transform infrared (FT-IR) spectra were acquired on Perkin Elmer Frontier FT-IR spectrometer. Raman spectroscopic measurements were carried out using Renishaw invia micro-Raman spectrometer. Ultraviolet-visible (UV-vis) spectra were recorded on the UV-1601PC (Shimadzu, Japan) spectrophotometer. The morphology of F16CoPc/MWCNTs–COOH hybrid is obtained by field-emission scanning electron microscopy (FE-SEM, Carl Zeiss, supra 55) and transmission electron microscopy (TEM, Jeol, TEM-2100). Thermo gravimetric analysis (TGA) was carried out using a thermogravimetry analyzer (Hitachi STA 7200) under a nitrogen atmosphere from 40 to 900 °C at a scan rate of 10 °C min−1. X-ray photoelectron spectroscopy (XPS) was performed using Mg Kα X-ray beams as the excitation source (1253.6 eV) and MAC2 electron analyzer system attached to MBE machine (EVA-32 Riber, France). The binding energy scale was calibrated to Au 4f7/2 line of 84.0 eV.
The gas sensing studies were performed in a home-built stainless steel test chamber (1000 ml) containing sample holder geometry as shown in Fig. 1. To fabricate the gas sensor, 2 mg of the as-prepared F16CoPc/MWCNTs–COOH hybrid was dispersed in 1 ml of DMF and then 30 μL of the above solution was poured onto the glass substrate with two pre-coated gold electrodes (3 mm × 3 mm at a spacing of 1 mm) and then allowed to dry at ambient temperature. The silver wires were connected to the gold electrodes using silver paste. The effective area of the sensor was 3 mm × 1 mm. Sensor resistance was recorded continuously by applying a constant bias of 3 V during both dosing and purging cycles as a function of time using computer interfaced Keithley electrometer 6517A. The internal temperature of test chamber is monitored by a thermocouple. A desired concentration of (Cl2, NO2, NO, C2H5OH, H2S, CO and NH3) gases in the test chamber was achieved by injecting a known quantity of gas using a micro-syringe. After exposure, once a steady state was achieved, sensor resistance was recovered by opening the lid of the test chamber. The response of the gas sensor was calculated using the eqn (1);
|
S (%) = |(Ra − Rg)/Ra| × 100
| (1) |
here,
Ra and
Rg represent the sensor resistance in air and gas environment, respectively. The response time is the time required for sensor resistance to reach 90% of its equilibrium value after the gas is introduced into the test chamber and the recovery time was measured as the time required for the sensor resistance to regain 90% of its original value after the removal of gas. XPS study of exposed samples was performed by
ex situ exposure of gases to the samples in gas sensing set-up (
Fig. 1) and transferring them to XPS analysis chamber. Electrochemical impedance spectroscopy study was performed using a frequency response analyzer (FRA) attached with a potentiostat (Autolab) in the frequency range of 10 Hz to 1 MHz.
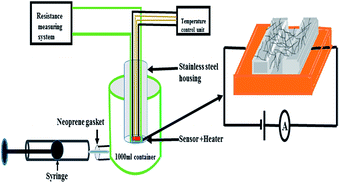 |
| Fig. 1 Gas sensing set-up used in the present study. | |
3. Results and discussion
3.1 Characterization of F16CoPc/MWCNTs–COOH hybrid
Fig. 2 shows the FTIR spectra of F16CoPc, MWCNTs–COOH and F16CoPc/MWCNTs–COOH hybrid. The observed IR peaks for F16CoPc (Fig. 2(a)) at positions 498, 605, 754, 845, 965 and 1158 cm−1 correspond to hexa-decafluoro substituents and two strong bands at 1496 and 1325 cm−1 correspond to the stretching of C
C and C
N, respectively; the other observed characteristic peaks at 1283, 1529 and 1622 cm−1 are attributed to phthalocyanine macrocycles.14,30,31 The MWCNTs–COOH (Fig. 2(b)) shows a peak at 1037 cm−1 corresponding to C–O stretching vibration, a peak at 3440 cm−1 due to the O–H stretching; the characteristic peak at 1637 cm−1 due to C
C stretching confirmed the graphitic structure of CNTs.32 The presence of peaks at 2855 and 2921 cm−1 are attributed to the asymmetric and symmetric CH2 stretching in CNTs.32,33 The peaks appearing in F16CoPc and MWCNTs–COOH (Fig. 2(c)) can be found in F16CoPc/MWCNTs–COOH hybrid and are observed to be red shifted in the wave-numbers in comparison to their individual peaks due to electron delocalization by π–π interaction between F16CoPc and CNTs. This observation demonstrates that F16CoPc molecules were successfully anchored onto the surface of MWCNTs–COOH.34
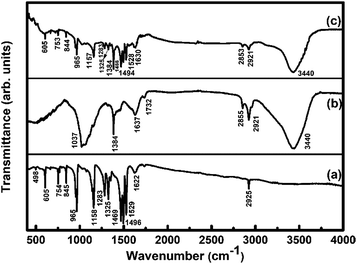 |
| Fig. 2 FTIR spectra of (a) F16CoPc; (b) MWCNTs–COOH and (c) F16CoPc/MWCNTs–COOH hybrid. | |
To study the interaction between F16CoPc molecules and MWCNTs–COOH, Raman spectra of F16CoPc/MWCNTs–COOH hybrid was compared with those of F16CoPc and MWCNTs–COOH (Fig. 3). The peaks at 143, 176, 208, 283, 470, 513, 587, 680, 738 and 965 cm−1 in F16CoPc sample were obtained due to vibrations of isoindole moieties.35 The peaks between 1200 and 1600 cm−1 correspond to pyrrole groups and a band at 1544 cm−1 corresponds to cobalt ion, which is in good agreement with reported studies.36,37 Raman spectra of MWCNTs–COOH exhibit the characteristic G-band (related to C–C vibration of the carbon material with a sp2 orbital structure) around 1593 cm−1 and D band (associated with sp2 C with defects) around 1360 cm−1.38,39 Comparison of Raman spectra of the hybrid with F16CoPc and MWCNTs–COOH shows a combination of their individual characteristic Raman peaks with a change in peak positions and their intensities. The intensity ratio of D band to the G band (ID/IG), known as a ratio of sp3-hybridized carbon atom relative to sp2-bonded carbon atom, is found to be 1.16 and 1.31 for MWCNTs–COOH and hybrid sample, respectively.40,41 This small variation of ID/IG ratio indicated that F16CoPc were attached to the surface of MWCNTs–COOH through a non-covalent modification.40
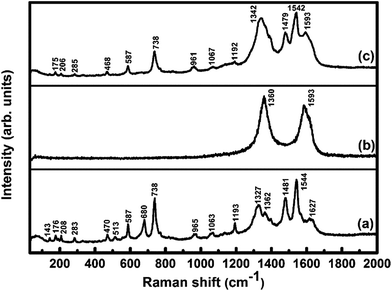 |
| Fig. 3 Raman spectra of (a) F16CoPc; (b) MWCNTs–COOH and (c) F16CoPc/MWCNTs–COOH hybrid. | |
Fig. 4 depicts the UV-visible absorption spectra of F16CoPc, MWCNTs–COOH and F16CoPc/MWCNTs–COOH hybrid. The UV-visible spectrum of F16CoPc exhibited two strong absorption bands; one broad B band in the wavelength at around 307 nm due to the electronic transitions from the highest occupied molecular orbital (HOMO) a2u to the lowest unoccupied molecular orbital (LUMO) eg level and the other Q band doublet at around 632 nm arising from the electronic transitions from HOMO a1u level to LUMO eg level.42 The UV-visible absorption spectra of MWCNTs–COOH has been found to be featureless.43 However, in case of hybrids, the Q-band is found to be comparatively broadened and red shifted by 20 nm as compared to that of F16CoPc. This observation is concomitant with an expanded macrocyclic conjugated structure of F16CoPc and reduced energy difference between the HOMO and the LUMO to facilitate charge transfer between the F16CoPc macrocycle and CNTs due to the π–π interaction.30,40
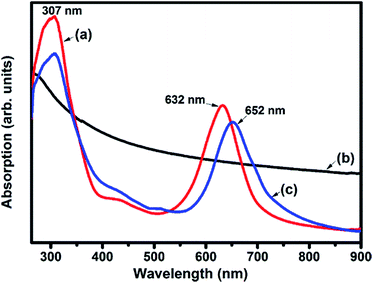 |
| Fig. 4 UV-visible absorption spectra of (a) F16CoPc; (b) MWCNTs–COOH and (c) F16CoPc/MWCNTs–COOH hybrid. | |
Fig. 5(a) shows TEM image of MWCNTs–COOH which are primarily empty long tubes with a mean diameter of 14 nm. The morphological features of the investigated hybrid (Fig. 5(b)) highlight the exo-hedral coverage of F16CoPc molecules on the sidewalls of MWCNTs–COOH with a mean diameter of about 40 nm in comparison to MWCNTs–COOH, confirming the anchoring of 26 nm thick F16CoPc molecules on MWCNTs–COOH. Further, Fig. 5(c) shows the SEM image of MWCNTs–COOH arranged in the groups of long tubular-shaped structures. The SEM image of F16CoPc/MWCNT–COOH hybrid (Fig. 5(d)) reveals that F16CoPc molecules are anchored on the surface of MWCNTs–COOH matrix making MWCNTs–COOH surface thicker and are in consonance with the TEM investigations.
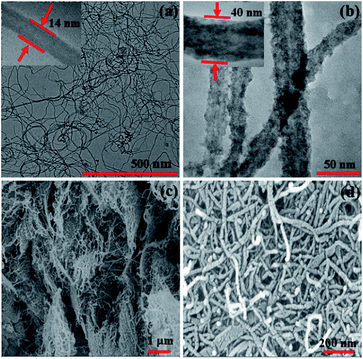 |
| Fig. 5 TEM images of (a) MWCNTs–COOH and (b) F16CoPc/MWCNTs–COOH hybrid (inset shows magnified view), and SEM images of (c) MWCNTs–COOH and (d) F16CoPc/MWCNTs–COOH hybrid. | |
The weight loss as a function of temperature for F16CoPc, MWCNTs–COOH and F16CoPc/MWCNTs–COOH hybrid materials was investigated using TGA plots (Fig. 6). An overall weight loss of 49.05% up to 900 °C is observed for F16CoPc (Fig. 6(a)) comprising major weight losses between 200 to 330 °C and 366 to 604 °C, corresponding to desorption of adsorbed water and the decomposition of F16CoPc, respectively.20,44,45 TGA plots of MWCNTs–COOH (Fig. 6(b)) exhibit a weight loss of about 8.11% due to destruction of the residual carbon and decarboxylation of oxidized species,45 whereas F16CoPc/MWCNTs–COOH (Fig. 6(c)) shows a weight loss of 23.93% on heating the hybrid to 900 °C, corresponding to decomposition of the F16CoPc on MWCNTs–COOH surface.20
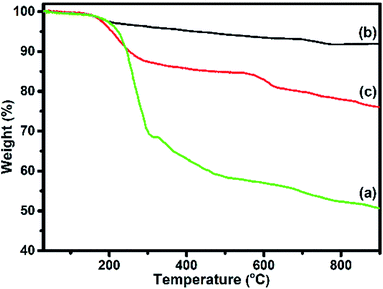 |
| Fig. 6 TGA curves of (a) F16CoPC; (b) MWCNTs–COOH and (c) F16CoPc/MWCNTs–COOH hybrid. | |
Further, the amount of F16CoPc molecule absorbed on the MWCNTs–COOH was calculated using the ratio of difference in weight loss between MWCNTs–COOH and F16CoPc/MWCNTs–COOH hybrid to weight loss for F16CoPc and was found to be 32.23%.
3.2 Gas sensing properties of F16CoPc/MWCNTs–COOH hybrid
To discuss the gas sensing characteristics of fabricated sensors (F16CoPc/MWCNTs–COOH), we exposed them to 500 ppb of different test gases (Cl2, NO2, NO, H2S, C2H5OH, NH3 and CO) at room temperature (25 °C). The selectivity histogram for the observed responses of the sensor for these test gases is shown in Fig. 7(a). It can be noted from the histogram that 0.3 wt% F16CoPc/MWCNTs–COOH sensor exhibits the best response among all the prepared sensors towards Cl2, with a sensitivity value of ∼26% and was subsequently chosen for further sensing characterization. For all other test gases, the sensitivity value was <4%. Nevertheless, the sensor exhibited an irreversible behaviour at room temperature as it was not able to recover to its baseline resistance value even after a long interval of time. Moreover, recovery characteristics of the sensor were found to be greatly improved on heating. In such a situation, to make the sensor reversible, the operating temperature of the sensors was optimized. To find an optimum operating temperature, we exposed the sensor to 500 ppb of Cl2 at different operating temperatures ranging from 30 to 200 °C and maximum response of ∼41% was obtained at a temperature of 150 °C (Fig. 7(b)). Furthermore, sensor response was observed to decrease beyond 150 °C due to desorption of Cl2 from sensor surface. Fig. 7(c) shows the variation of response curve for different doses of Cl2 (40–2000 ppb) at 150 °C. It demonstrates that there is a decrease in the sensor resistance after exposure to Cl2 and it gets saturated after some time and again starts approaching to its initial baseline value after removal of Cl2, which reveals the good reversibility of sensor.
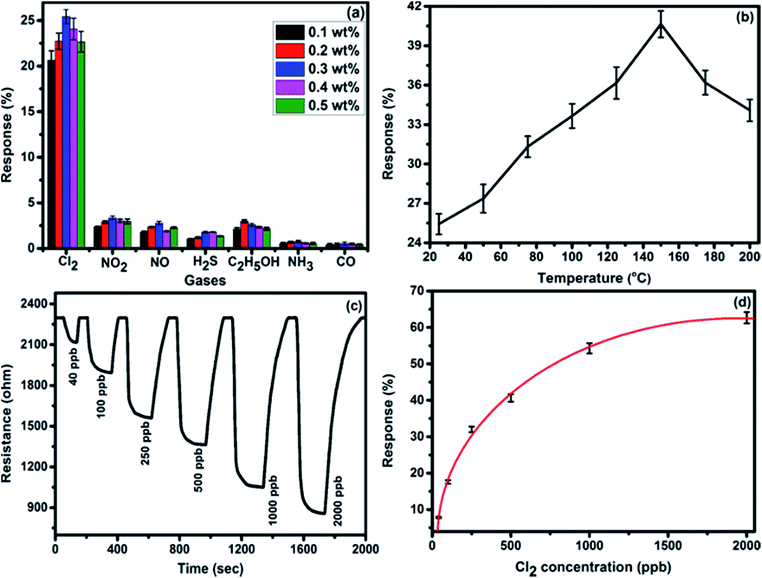 |
| Fig. 7 (a) Selectivity histogram of F16CoPc/MWCNTs–COOH sensors for 500 ppb of Cl2, NO2, NO, C2H5OH, H2S, NH3 and CO at room temperature. (b) Sensor response as a function of temperature for 500 ppb of Cl2 concentration. (c) Response curves of sensor for different doses of Cl2 at 150 °C. (d) Variation in the response of sensor with Cl2 concentration (experimental curve (dotted lines) and fitting curve (solid lines)). (The standard error bars indicate the response variations using three times repeated testing of three sensor devices). | |
Fig. 7(d) exhibits the response behaviour of F16CoPc/MWCNTs–COOH sensor to 40–2000 ppb concentrations of Cl2 at 150 °C, which indicates its increased response with the increase in Cl2 concentration. This behaviour can be explained on the basis of surface area and the number of effective occupancy of active sites available on the sensor film provided by phthalocyanine molecules anchored on MWCNTs–COOH, which is in good agreement with the morphological study of the hybrid.46
There is lesser coverage of surface area at a lower concentration of Cl2 and hence it interacts with lesser number of active sites available on sensor surface, leading to lower response. Nevertheless, at a higher concentration, Cl2 covers comparatively larger surface area and interacts with larger number of active sites leading to higher sensor response.
Fig. 8 shows the response curves of F16CoPc/MWCNTs–COOH sensor for successive exposures of Cl2 in order to investigate the reproducibility of the sensor. The nearly same value of sensor response without any drift in the baseline resistance indicates the reproducible response characteristics of the sensor.
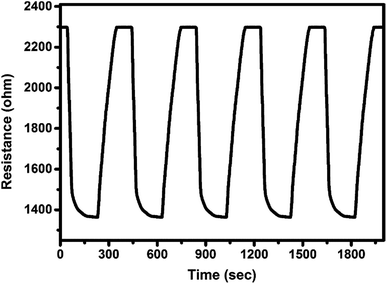 |
| Fig. 8 Reproducibility of the response curve of F16CoPc/MWCNTs–COOH sensor to 500 ppb of Cl2 at 150 °C. | |
Furthermore, response variation with the gas concentration was studied using eqn (2)47,48
|
 | (2) |
where
α and
β are coefficients that depend on operating temperature and testing material. The constant
α is adsorption capacity and
β is the strength of adsorption,
48 and they were calculated by curve fitting of response curve. The smaller the value of
β, the greater is the expected heterogeneity; the value of
β lies between 0 and 1 for normal adsorption. The values of
α and
β are found to be 1.25 and 0.53, respectively. The value of
β is lesser than one, which indicates the normal mode of adsorption on the heterogeneous surface of sensor.
47
The lowest detectable concentration of the sensor is dependent on the experimental set up used. Further, the limit of detection (LOD) of the sensor has been derived from signal-to-noise ratio (S/N). The signal-to-noise ratio is defined as ΔR/σ, where ΔR is the maximum resistance change with respect to R0 (baseline resistance) and σ refers to the root mean square (rms) noise of the baseline before exposure to gas.49
LOD is calculated using eqn (3);50
|
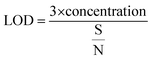 | (3) |
The signal-to-noise ratio of the sensor was 2400 and the theoretical detection limit of sensor was calculated to be 0.05 ppb.
3.3 Gas sensing mechanism
The underlying gas sensing mechanism of the sensor was investigated using XPS, Raman and impedance spectroscopy carried out before and after exposure to Cl2. The comparison of Raman spectra (Fig. 9) of the unexposed sample with the exposed sample shows a shift in position of some peaks after Cl2 exposure. On Cl2 exposure, the peak corresponding to cobalt–nitrogen bond51 at 175 cm−1 is found to shift by 7 cm−1 and peaks of macro-cyclic vibrations52 (206, 285, 468, 587, 738, 961 and 1192 cm−1) are shifted by 5 cm−1, whereas D and G band corresponding to MWCNTs–COOH at 1342 and 1593 cm−1 are shifted by 2 and 1 cm−1, respectively.38,39 The significant shift of 9 cm−1 is observed for the Raman peak at 1542 cm−1, which is due to displacement of C–N–C bridge bond, closely linked to the central metal ion of phthalocyanine molecule.53 This observation is concomitant with the predominant interaction of Cl2 with the cobalt ions of the hybrid sensor. Raman spectrum of the hybrid, recorded after removing Cl2, exhibits identical peaks as detected in fresh samples, which indicates the good reversibility of the sensor.
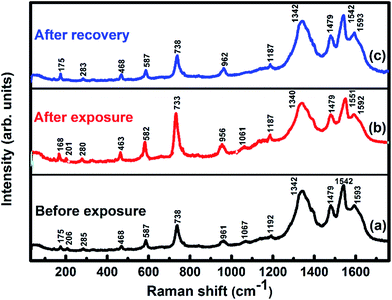 |
| Fig. 9 Raman spectra of F16CoPc/MWCNTs–COOH sensor recorded (a) before exposure; (b) after exposure to 25 ppm of Cl2 and (c) after full recovery. | |
Furthermore, X-ray photoelectron spectroscopy (XPS) (Fig. 10) of fresh F16CoPc/MWCNTs–COOH hybrid possesses peaks at 284.8, 532.4, 399.2, 687.0, 780.7 and 795.9 eV corresponding to C-1s, O-1s, N-1s, F-1s, Co-2p3/2 and Co-2p1/2 levels, respectively.54,55 In addition, on Cl2 exposure, there is a peak shift of 0.2 eV in the spectrum of core level C-1s, a shift of 0.1 eV in spectrum of O-1s and F-1s, a shift of 0.3 eV in spectrum of N-1s and a prominent peak shift of 0.8 eV in the core level spectrum of Co-2p. The major shift of 0.8 eV towards higher BE side in the Co-2p core level and shifting of peak position of nitrogen, oxygen and fluorine spectrum towards higher binding energy confirms that charge transfer interaction occurs upon adsorption of strong electron acceptor Cl2 molecules55 onto the hybrid, leading to decrease in electron density due to transfer of electrons from hybrid to Cl2. Thus, the study of Raman and XPS spectroscopic investigations demonstrate that on adsorption of Cl2, charge transfer takes place between Cl2 and hybrid through central metal cobalt ion.55 Herein, the charge can favourably travel from CNTs to F16CoPc, leading to an increase in hole concentration in CNTs and this results in fast variation of sensor resistance (Fig. 7(c)).55,56 Moreover, it has been observed that XPS spectrum, recorded after recovery shows no shifting of peak position and absence of any chlorine signal confirms that the sensing process is highly reproducible.
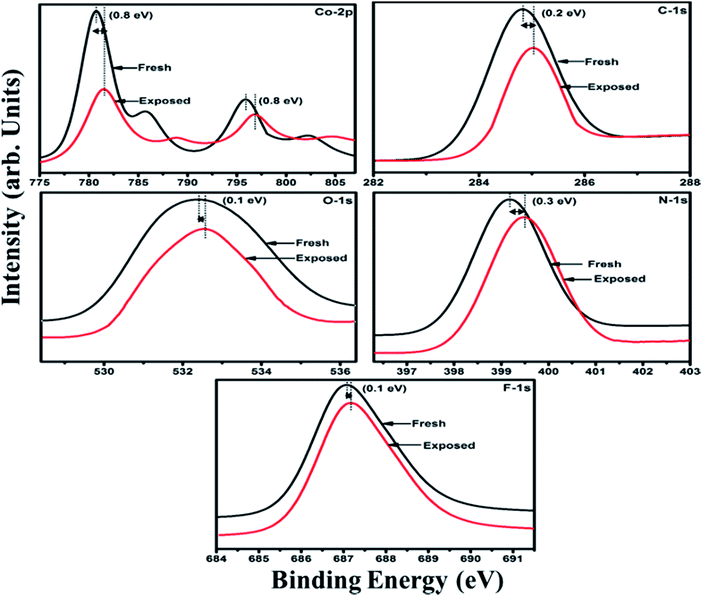 |
| Fig. 10 XPS spectra of F16CoPc/MWCNTs–COOH sensor recorded before and after exposure to 25 ppm of Cl2. | |
As demonstrated, in morphological studies, the sensing layer consists of grains of F16CoPc/MWCNTs–COOH and their respective grain boundaries. Nevertheless, exact contribution of these grains and grain boundaries towards sensing mechanism can be calculated by impedance spectroscopy of the prepared sensor. Fig. 11 shows Cole–Cole plot46,57 between imaginary components of impedance (−Z′′) as a function of real component (Z′) of F16CoPc/MWCNTs–COOH sensor in the presence of air and after exposure to 500 ppb of Cl2. These plots exhibit a single semi-circle before and after exposure to Cl2; their equivalent circuit consisting of RC network in series with a resistor R0 is shown in the inset in Fig. 11. Herein, R0 is the grain resistance and can be estimated from the intercept of the semi-circle at high frequency with the real axis. R1 and C1 are resistance and capacitance across grain boundaries, where R1 can be determined from the diameter of the arc in Fig. 11, while C1 can be calculated from the relation ωmaxR1C1 = 1, where ωmax is the frequency corresponding to the top of the arc.57 The obtained values of R0, R1 and C1 for the sensor using equivalent circuit are tabulated in Table 1.
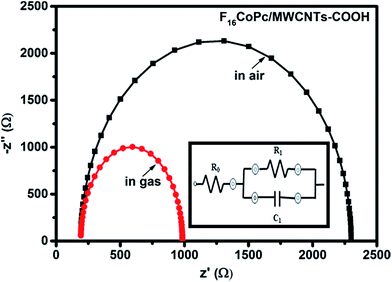 |
| Fig. 11 Impedance spectra of fresh and Cl2 exposed F16CoPc/MWCNTs–COOH sensor (inset shows the equivalent circuit used for analysis of data obtained from F16CoPc/MWCNTs–COOH sensor). | |
Table 1 Impedance parameters obtained for F16CoPc/MWCNTs–COOH sensor by fitting experimental data to the equivalent circuit
Sensor |
Conditions |
Parameters |
R0 (Ω) |
R1 (Ω) |
C1 (nF) |
F16CoPc/MWCNTs–COOH |
Unexposed |
192 |
2108 |
3.34 |
Exposed to 500 ppb Cl2 |
192 |
792 |
7.15 |
The mathematical formulation for this equivalent circuit46 can be given as follows:
where,
Z′ = R0 + [R1/(1 + ωR1C1)2] |
and
Z′′ = [ωR12C1/(1 + ωR1C1)2] |
It has been observed that the parameter R0 remained nearly the same in air and in the presence of Cl2, whereas R1 decreases and C1 increases on exposure to Cl2 gas. This can be addressed on the basis of incoming Cl2 adsorbed onto the grains outer surfaces leading to transfer of electron from hybrid to Cl2 and improves the hole-conductivity through charge transfer between phthalocyanines and MWCNTs–COOH in concomitant with XPS results.57
Comparatively, a drastic fall in resistance (R1) across grain boundary along with larger shift in BE (0.8 eV) and Raman shift (9 cm−1) of cobalt ion on exposing the F16CoPc/MWCNTs–COOH23 sensor to Cl2 in comparison to F16CuPc/MWCNTs–COOH–23 and F16ZnPc/MWCNTs–COOH–24 based sensor establish the formation of an efficient charge transfer between F16CoPc/MWCNTs–COOH sensor and Cl2 to make an improved chlorine sensor.
4. Conclusions
In conclusion, we have explored F16CoPc as functional moieties for the non-covalent functionalization of CNTs through the solution assembly method and subsequently the F16CoPc/MWCNTs–COOH hybrid was investigated as ppb-level Cl2 sensor. In comparison to F16CuPc/MWCNTs–COOH and F16ZnPc/MWCNTs–COOH sensors, the present results demonstrate that the F16CoPc/MWCNTs–COOH sensor exhibits high sensitivity (62.66% for 2 ppm with LOD of 0.05 ppb), excellent reproducibility and selectivity. X-ray photoelectron, Raman and electrochemical impedance spectroscopic studies revealed the efficient charge transfer between F16CoPc/MWCNTs–COOH sensor and chlorine. These outcomes underline the potential of such hybrid material in developing a new low cost Cl2 sensor with excellent gas sensing characteristics.
Conflicts of interest
There are no conflict to declare.
Acknowledgements
The authors are thankful to Council of Scientific and Industrial Research (CSIR), New Delhi, India for providing financial assistance to accomplish this research work.
Notes and references
- Z. Ting, M. Syed, V. M. Nosang and A. D. Marc, Nanotechnology, 2008, 19, 332001 CrossRef PubMed.
- A. Kumar, A. Singh, A. K. Debnath, S. Samanta, D. K. Aswal, S. K. Gupta and J. V. Yakhmi, Talanta, 2010, 82, 1485–1489 CrossRef CAS PubMed.
- S. Iijima, Nature, 1991, 354, 56–58 CrossRef CAS.
- A. Das, R. Dost, T. H. Richardson, M. Grell, D. C. Wedge, D. B. Kell, J. J. Morrison and M. L. Turner, Sens. Actuators, B, 2009, 137, 586–591 CrossRef CAS.
- J. G. Weis, J. B. Ravnsbæk, K. A. Mirica and T. M. Swager, ACS Sens., 2016, 1, 115–119 CrossRef CAS.
- A. L. Ndiaye, C. Varenne, P. Bonnet, É. Petit, L. Spinelle, J. Brunet, A. Pauly and B. Lauron, Thin Solid Films, 2012, 520, 4465–4469 CrossRef CAS.
- E. S. Snow, F. K. Perkins, E. J. Houser, S. C. Badescu and T. L. Reinecke, Science, 2005, 307, 1942–1945 CrossRef CAS PubMed.
- N. H. Quang, M. Van Trinh, B.-H. Lee and J.-S. Huh, Sens. Actuators, B, 2006, 113, 341–346 CrossRef.
- Z. J. Han, H. Mehdipour, X. Li, J. Shen, L. Randeniya, H. Y. Yang and K. Ostrikov, ACS Nano, 2012, 6, 5809–5819 CrossRef CAS PubMed.
- R. Leghrib, A. Felten, J. J. Pireaux and E. Llobet, Thin Solid Films, 2011, 520, 966–970 CrossRef CAS.
- M. Penza, G. Cassano, R. Rossi, M. Alvisi, A. Rizzo, M. A. Signore, T. Dikonimos, E. Serra and R. Giorgi, Appl. Phys. Lett., 2007, 90, 173123 CrossRef.
- B. Wang, Y. Wu, X. Wang, Z. Chen and C. He, Sens. Actuators, B, 2014, 190, 157–164 CrossRef CAS.
- A. K. Sharma, R. Saini, R. Singh, A. Mahajan, R. K. Bedi and D. K. Aswal, AIP Conf. Proc., 2014, 1591, 671–673 CrossRef CAS.
- X. Liang, Z. Chen, H. Wu, L. Guo, C. He, B. Wang and Y. Wu, Carbon, 2014, 80, 268–278 CrossRef CAS.
- L. H. H. Hsu, E. Hoque, P. Kruse and P. Ravi Selvaganapathy, Appl. Phys. Lett., 2015, 106, 063102 CrossRef.
- J. Muñoz, F. Céspedes and M. Baeza, Microchem. J., 2015, 122, 189–196 CrossRef.
- J. Li, Y. Lu and M. Meyyappan, IEEE Sens. J., 2006, 6, 1047–1051 CrossRef CAS.
- A. Gohier, J. Chancolon, P. Chenevier, D. Porterat, M. Mayne-L'Hermite and C. Reynaud, Nanotechnology, 2011, 22, 105501 CrossRef CAS PubMed.
- A. Yazc, N. Unus, A. Altndal, B. Salih and O. Bekaroglu, Dalton Trans., 2012, 41, 3773–3779 RSC.
- E. N. Kaya, T. Basova, M. Polyakov, M. Durmus, B. Kadem and A. Hassan, RSC Adv., 2015, 5, 91855–91862 RSC.
- R. Saini, A. Mahajan, R. K. Bedi, D. K. Aswal and A. K. Debnath, Sens. Actuators, B, 2014, 198, 164–172 CrossRef CAS.
- R. Saini, A. Mahajan, R. K. Bedi, D. K. Aswal and A. K. Debnath, RSC Adv., 2014, 4, 15945–15951 RSC.
- A. K. Sharma, A. Mahajan, R. Saini, R. K. Bedi, S. Kumar, A. K. Debnath and D. K. Aswal, Sens. Actuators, B, 2018, 255, 87–99 CrossRef CAS.
- A. K. Sharma, A. Mahajan, R. K. Bedi, S. Kumar, A. K. Debnath and D. K. Aswal, Appl. Surf. Sci., 2018, 427, 202–209 CrossRef CAS.
- B. Kadem, M. Göksel, A. Şenocak, E. Demirbaş, D. Atilla, M. Durmuş, T. Basova, K. Shanmugasundaram and A. Hassan, Polyhedron, 2016, 110, 37–45 CrossRef CAS.
- H. Jiang, J. Ye, P. Hu, F. Wei, K. Du, N. Wang, T. Ba, S. Feng and C. Kloc, Sci. Rep., 2014, 4, 7573 CrossRef CAS PubMed.
- P. A. Pandey, L. A. Rochford, D. S. Keeble, J. P. Rourke, T. S. Jones, R. Beanland and N. R. Wilson, Chem. Mater., 2012, 24, 1365–1370 CrossRef CAS.
- D. G. de Oteyza, A. El-Sayed, J. M. Garcia-Lastra, E. Goiri, T. N. Krauss, A. Turak, E. Barrena, H. Dosch, J. Zegenhagen, A. Rubio, Y. Wakayama and J. E. Ortega, J. Chem. Phys., 2010, 133, 214703 CrossRef CAS PubMed.
- J. Liu, A. G. Rinzler, H. Dai, J. H. Hafner, R. K. Bradley, P. J. Boul, A. Lu, T. Iverson, K. Shelimov, C. B. Huffman, F. Rodriguez-Macias, Y.-S. Shon, T. R. Lee, D. T. Colbert and R. E. Smalley, Science, 1998, 280, 1253–1256 CrossRef CAS PubMed.
- Z. Yang, H. Pu, J. Yuan, D. Wan and Y. Liu, Chem. Phys. Lett., 2008, 465, 73–77 CrossRef CAS.
- Y. Wan, Q. Liang, Z. Li, S. Xu, X. Hu, Q. Liu and D. Lu, J. Mol. Catal. A: Chem., 2015, 402, 29–36 CrossRef CAS.
- Z. Zhao, Z. Yang, Y. Hu, J. Li and X. Fan, Appl. Surf. Sci., 2013, 276, 476–481 CrossRef CAS.
- M. Penza, R. Rossi, M. Alvisi, M. A. Signore, G. Cassano, D. Dimaio, R. Pentassuglia, E. Piscopiello, E. Serra and M. Falconieri, Thin Solid Films, 2009, 517, 6211–6216 CrossRef CAS.
- P. Li, Y. Ding, A. Wang, L. Zhou, S. Wei, Y. Zhou, Y. Tang, Y. Chen, C. Cai and T. Lu, ACS Appl. Mater. Interfaces, 2013, 5, 2255–2260 CAS.
- M. Anghelone, D. Jembrih-Simbürger and M. Schreiner, Spectrochim. Acta, Part A, 2015, 149, 419–425 CrossRef CAS PubMed.
- S. Tuncel, E. N. Kaya, M. Durmus, T. Basova, A. G. Gurek, V. Ahsen, H. Banimuslem and A. Hassan, Dalton Trans., 2014, 43, 4689–4699 RSC.
- M. Szybowicz and J. Makowiecki, J. Mater. Sci., 2012, 47, 1522–1530 CrossRef CAS.
- A. Wang, Y. Wang, W. Yu, Z. Huang, Y. Fang, L. Long, Y. Song, M. P. Cifuentes, M. G. Humphrey, L. Zhang, J. Shao and C. Zhang, RSC Adv., 2016, 6, 20120–20127 RSC.
- P. C. Eklund, J. M. Holden and R. A. Jishi, Carbon, 1995, 33, 959–972 CrossRef CAS.
- Y. Wang, N. Hu, Z. Zhou, D. Xu, Z. Wang, Z. Yang, H. Wei, E. S.-W. Kong and Y. Zhang, J. Mater. Chem., 2011, 21, 3779–3787 RSC.
- V. Datsyuk, M. Kalyva, K. Papagelis, J. Parthenios, D. Tasis, A. Siokou, I. Kallitsis and C. Galiotis, Carbon, 2008, 46, 833–840 CrossRef CAS.
- H. Banimuslem, A. Hassan, T. Basova, A. D. Gülmez, S. Tuncel, M. Durmuş, A. G. Gürek and V. Ahsen, Sens. Actuators, B, 2014, 190, 990–998 CrossRef CAS.
- T. Mugadza and T. Nyokong, Electrochim. Acta, 2009, 54, 6347–6353 CrossRef CAS.
- B.-P. Jiang, L.-F. Hu, D.-J. Wang, S.-C. Ji, X.-C. Shen and H. Liang, J. Mater. Chem. B, 2014, 2, 7141–7148 RSC.
- J.-Q. Zeng, S.-N. Sun, J.-P. Zhong, X.-F. Li, R.-X. Wang, L.-N. Wu, L. Wang and Y.-J. Fan, Int. J. Hydrogen Energy, 2014, 39, 15928–15936 CrossRef CAS.
- A. T. Mane, S. T. Navale, S. Sen, D. K. Aswal, S. K. Gupta and V. B. Patil, Org. Electron., 2015, 16, 195–204 CrossRef CAS.
- D. Kumar, P. Chaturvedi, P. Saho, P. Jha, A. Chouksey, M. Lal, J. S. B. S. Rawat, R. P. Tandon and P. K. Chaudhury, Sens. Actuators, B, 2017, 240, 1134–1140 CrossRef CAS.
- I. Sayago, H. Santos, M. C. Horrillo, M. Aleixandre, M. J. Fernández, E. Terrado, I. Tacchini, R. Aroz, W. K. Maser, A. M. Benito, M. T. Martínez, J. Gutiérrez and E. Muñoz, Talanta, 2008, 77, 758–764 CrossRef CAS.
- F. Rigoni, S. Tognolini, P. Borghetti, G. Drera, S. Pagliara, A. Goldoni and L. Sangaletti, Analyst, 2013, 138, 7392–7399 RSC.
- G. Chen, T. M. Paronyan, E. M. Pigos and A. R. Harutyunyan, Sci. Rep., 2012, 2, 343 CrossRef PubMed.
- G. S. S. Saini, S. Sukhwinder, K. Sarvpreet, K. Ranjan, S. Vasant and S. K. Tripathi, J. Phys.: Condens. Matter, 2009, 21, 225006 CrossRef CAS PubMed.
- R. Kötz and E. Yeager, J. Electroanal. Chem. Interfacial Electrochem., 1980, 113, 113–125 CrossRef.
- M. Szybowicz, W. Bała, K. Fabisiak, K. Paprocki and M. Drozdowski, J. Mater. Sci., 2011, 46, 6589–6595 CrossRef CAS.
- J.-H. Yang, Y. Gao, W. Zhang, P. Tang, J. Tan, A.-H. Lu and D. Ma, J. Phys. Chem., 2013, 117, 3785–3788 CAS.
- R. A. Hatton, N. P. Blanchard, V. Stolojan, A. J. Miller and S. R. P. Silva, Langmuir, 2007, 23, 6424–6430 CrossRef CAS PubMed.
- A. L. Verma, S. Saxena, G. S. S. Saini, V. Gaur and V. K. Jain, Thin Solid Films, 2011, 519, 8144–8148 CrossRef CAS.
- N. H. Al-Hardan, M. J. Abdullah and A. A. Aziz, Int. J. Hydrogen Energy, 2010, 35, 4428–4434 CrossRef CAS.
|
This journal is © The Royal Society of Chemistry 2017 |