DOI:
10.1039/C7RA07803J
(Paper)
RSC Adv., 2017,
7, 39221-39227
Valorization of an underused sugar derived from hemicellulose: efficient synthesis of 5-hydroxymethylfurfural from mannose with aluminum salt catalyst in dimethyl sulfoxide/water mixed solvent†
Received
15th July 2017
, Accepted 1st August 2017
First published on 11th August 2017
Abstract
Converting saccharides into 5-hydroxymethylfurfural (5-HMF) has attracted more and more research interest under the background of global energy shortage. A lot of effort has been devoted to the conversion of fructose and glucose. However, other underused sugars receive very limited attention, although some of them have considerable reserves in nature as well. In this work, mannose, a major component from hemicellulose, was effectively converted into 5-HMF under mild conditions. AlCl3·6H2O exhibited superior activity among the tested catalysts, achieving a maximum 5-HMF yield of 60% in a dimethyl sulfoxide (DMSO)/water mixed solvent at 130 °C within 45 min. Adding an appropriate amount of water in DMSO could suppress some side reactions while preserving the reactivity of mannose dehydration. Mannose showed a comparable reactivity to fructose in the employed catalytic system. The studied system was also effective in the conversion of di/trisaccharides such as cellobiose and melezitose into 5-HMF. A number of control experiments with different catalysts and additives were conducted to elucidate the preliminary mechanism.
1. Introduction
5-Hydroxymethylfurfural (5-HMF) represents a versatile biobased platform compound.1 5-HMF can be refined into varieties of high value added fine chemicals and transport fuels through oxidation, reduction, etherification, amination, alkylation and condensation.2–8 The synthesis of 5-HMF from carbohydrates is consistent with the scope of sustainable development in the future, and thus, 5-HMF has attracted widespread attention.1 5-HMF was recognized as an important and potential platform compound as early as 1990.9 Entering the 21st century, the pioneering work reported by Dumesic et al. and Zhang et al., respectively, has set off new studies toward the conversion of biomass into 5-HMF worldwide.10,11
Fructose and glucose are the most common carbohydrates for 5-HMF production.1 Fructose exists in a considerable proportion of furanoses (e.g. α- and β-furanose account for ∼31.5% of fructose at equilibrium in water at 31 °C),12 which are proposed as the active species,13 so fructose can be readily converted into 5-HMF with various catalysts, such as mineral acids, metal salts, zeolites, ionic liquids and other functionalized materials.14–19 By contrast, glucose remains recalcitrant to most catalysts used for fructose conversion. Glucose almost exists in the form of α- and β-pyranose.12 As shown in Scheme 1, it is generally accepted that glucose undergoes a two-step tandem reaction to produce 5-HMF (isomerization of glucose into fructose and dehydration of fructose into 5-HMF).11,20,21 The representative catalysts for glucose conversion typically include an active metal center (e.g. Cr, Sn, Al, Ge), which is mainly used in the form of metal salt.11,13,22–24 As a result of the above work, some related polymer feedstocks such as inulin and cellulose have been exploited as well.25–28
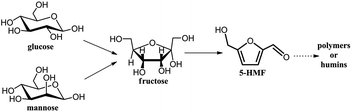 |
| Scheme 1 Schematic path on the conversion of glucose and mannose into 5-HMF. | |
In addition to fructose and glucose, other underused sugars receive far less attention in the aspect of 5-HMF synthesis, although some of them have considerable reserves in nature. As shown in Scheme 1, mannose is the C-2 epimer of glucose. Mannose is one of the major hexoses in hemicellulose.29 For instance, mannose can account for ∼10% of the dry weight of pine wood.30 Efficient conversion of mannose into 5-HMF would provide reference for the utilization of hemicellulose. However, information on such conversion still remains limited at present. Binder et al. previously demonstrated that mannose showed a similar reactivity to that of glucose in N,N-dimethylacetamide (DMA)–lithium chloride/bromide (LiCl/LiBr) with CrCl2 or CrCl3 as the catalyst. A 5-HMF yield of 69% was achieved in DMA–LiBr at 100 °C after 2 h.31 De et al. obtained a 5-HMF yield of 45% from mannose in DMA–LiCl with a H-form TiO2 catalyst at 140 °C under microwave.32 Most recently, Wrigstedt et al. gained a 5-HMF yield of 69% in a potassium bromide (aq.)—γ-valerolactone biphasic system with CrCl3·6H2O and Amberlyst-38 at 160 °C under microwave.33 Bhanja et al. synthesized a bifunctionalized mesoporous SBA-15 catalyst which converted mannose into 5-HMF in dimethyl sulfoxide (DMSO) with a yield of 61% at 135 °C under microwave.34 Flannelly et al. investigated the conversion of mannose and glucose in sulphuric acid solution. Both hexoses underwent comparable conversions with levulinic acid yields of ∼45%, a followed product through the hydration of 5-HMF, at 150 °C after 6 h.35 Guo et al. studied the conversion of mannose in aqueous medium with Nb4W4 catalyst at 120 °C, while the 5-HMF yield was lower than 10%.36 The above studies used either complicated system or microwave, and it will still be necessary to develop facile and effective approaches to produce 5-HMF by mannose and other underused sugars.
In this work, we were committed to explore effective approaches to convert mannose into 5-HMF. We employed a series of metal salts and mineral acids as the catalysts. Some aluminum salts showed good catalytic activities in a DMSO/H2O mixed solvent. Some parameters such as water content, catalyst type and loading, reaction temperature, reaction time, feedstock type were investigated in detail. A number of control experiments with different catalysts or simple model compounds were also conducted to facilitate understanding the reaction mechanism.
2. Experimental
2.1 Materials
D-Mannose (99%), D-glucose (99%), D-fructose (99%), D-melezitose monohydrate (99%), 5-hydroxymethylfurfural (5-HMF, 99%), MnCl2·4H2O (99%), NiCl2·6H2O (99%), FeCl2·4H2O (99%), FeCl3·6H2O (99%), LaCl3·7H2O (99%), CoCl2·6H2O (99%), ZnCl2 (99%), AlCl3·6H2O (98%), InCl3·4H2O (99%), 1,3-dihydroxyacetone (97%) and levulinic acid (99%) were purchased from Aladdin (China). D-Cellobiose (98%) and CuCl2·2H2O (99%) were purchased from Alfa Aesar (China). CrCl3·6H2O (98%) and DL-glyceraldehyde (90%) were purchased from Sigma-Aldrich (China). YbCl3·6H2O (99%), aluminum trifluoromethanesulfonate (Al(OTf)3, 99%) and AlBr3 (99%) were purchased from Energy Chemical (China). SnCl4·5H2O (99%), H3BO3 (99%), AlCl3 (99%), Al(NO3)3·9H2O (98%), Al2(SO4)3·18H2O (99%), dimethyl sulfoxide (DMSO, 99%), Al(OH)3 (99%), glycerol (99%) and 1,2-propanediol (99%) were purchased from Sinopharm (China). Hydrochloric acid (HCl, 37 wt%) and sulfuric acid (H2SO4, 98 wt%) were provided by a local supplier. All of the commercial chemicals were used as received. Purified water (H2O) with a resistivity of 18.2 MΩ cm was produced by an ultra-pure water system (Taoshi Brand, China).
2.2 Reaction procedure
Typically, mannose (60 mg), catalyst (10 mol% to mannose) and solvent (1 mL) were added into a reaction vial with a magnetic stir bar. The vial was sealed and inserted into a heating block. The mixture was stirred at a speed of ∼400 rpm at the reaction temperature. After a specified time, the vial was taken out and immersed in an ice-water bath to quench the reaction. Then, 1 mL of H2O and a certain amount of glycerol (internal standard) were added into the vial. A small amount of reaction mixture was taken out and further diluted with H2O. All the samples were analyzed by high performance liquid chromatography (HPLC) after filtration.
2.3 Analysis method
HPLC was performed on a Shimadzu LC-16 system equipped with a Shimadzu RID-20 refractive index detector and an Agilent Hi-Plex ligand exchange column (H-form, 300 × 7.7 mm). A 0.005 M aqueous solution of H2SO4 was used as the mobile phase with a flow rate of 0.65 mL min−1. The column and detector temperatures were 65 °C and 50 °C, respectively. Glycerol was added as the internal standard for quantitative calculations. It should be specified that the retention times of 1,3-dihydroxyacetone and glycerol are overlapped under the analysis conditions, and 1,3-dihydroxyacetone could be an isomerization product from glyceraldehyde. Thus, 1,2-propanediol was used as an alternative internal standard for the control experiments with glyceraldehyde, 1,3-dihydroxyacetone and glycerol. The conversion and product yield data were calculated by mole.
3. Results and discussion
3.1 Catalyst screening test
Mannose is the C-2 epimer of glucose,29 implying that a catalyst enabling the conversion of glucose into 5-HMF could be the potential candidate in this work. According to previous report on glucose conversion,11,22,23,37–43 a wide range of metal salts and mineral acids were employed as the catalysts for the preliminary test.
Fig. 1 illustrates the results of catalyst screening in DMSO at 130 °C for 30 min. As shown, the conversion of mannose did not essentially occur in the absence of a catalyst, with very low conversion (∼5%), and no 5-HMF was detected. MnCl2·4H2O, FeCl2·4H2O, FeCl3·6H2O, CuCl2·2H2O, CoCl2·6H2O and H3BO3 did not catalyze the formation of 5-HMF, possibly because these catalysts were unable to isomerize mannose into a proper intermediate, such as fructose. Although 5-HMF could be produced by employing NiCl2·6H2O, LaCl3·7H2O, ZnCl2, YbCl3·6H2O and H2SO4, the 5-HMF yields were still low. The large difference between mannose conversion and 5-HMF yield over FeCl3·6H2O, CuCl2·2H2O and H2SO4 indicated that a significant level of side reactions occurred. The side products were proposed to be undesired oligomers, polymers and humins.44
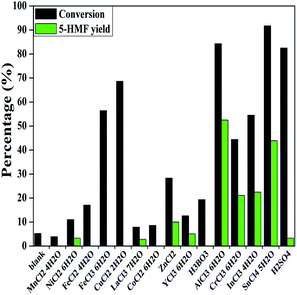 |
| Fig. 1 Conversion of mannose in the presence of different catalysts in DMSO at 130 °C for 30 min. | |
By contrast, CrCl3·6H2O and InCl3·4H2O led to moderate yields of 5-HMF (∼22%). The reaction with SnCl4·5H2O achieved an improved 5-HMF yield (∼44%). Among the tested catalysts, AlCl3·6H2O exhibited the superior catalytic performance with ∼84% conversion and a 5-HMF yield of ∼52%. The anhydrous AlCl3 was also tested, giving very close results to AlCl3·6H2O (∼87% conversion and ∼51% 5-HMF yield). In consideration of the hydroscopicity of AlCl3, leading to difficulty for weighing, AlCl3·6H2O was employed for subsequent studies. As reported, some metal salts (e.g. CrCl3, AlCl3) may hydrolyze into metal ion species (e.g. M(OH)2+ or M(OH)2+) and companion acids during the dehydration of glucose into 5-HMF.45–47 It was proposed that the metal ion species mainly played a role in isomerizing glucose into fructose, while the companion acid catalyzed the subsequent dehydration of fructose into 5-HMF.45,48 The conversion of mannose into 5-HMF is a dehydration process, and the produced water will promote the hydrolysis of metal salts. Accordingly, the latter four metal salts may possess the aforementioned properties during the reaction, leading to the production of 5-HMF.45–47
3.2 Effect of water content in DMSO
During the catalyst screening tests, AlCl3·6H2O exhibited a poor solubility in DMSO and some AlCl3·6H2O precipitation appeared after reaction. The other tested metal salts could dissolve well in DMSO. It is speculated that the undissolved AlCl3·6H2O may limit 5-HMF yield. To overcome this problem, we attempted to add an appropriate amount of water as co-solvent. As shown in Fig. S1,† adding little water obviously improved the dissolution of AlCl3·6H2O. Therefore, the effect of water content in DMSO was next examined.
Five DMSO/H2O solutions with volume fractions of 1000/0, 950/50, 900/100, 800/200 and 600/400 (μL μL−1) were employed for this investigation. The molar fractions of water (χw) in the solutions were calculated to be 0, 0.17, 0.3, 0.5 and 0.72. Fig. 2 presents AlCl3·6H2O catalyzed conversion of mannose in DMSO with different amounts of water at 130 °C. The mannose conversion remained at 81–86% in the systems of χw ≤ 0.3, and it slightly decreased to 71% when χw reached 0.5. However, the mannose conversion sharply dropped to 17% with further increasing χw to 0.72. Because the formation of 5-HMF from mannose is a dehydration reaction, water of course will inhibit such process. However, DMSO/H2O binary mixtures retain DMSO-like properties at χw < 0.5,49 which could be the reason that relatively high conversions (71–86%) were obtained in DMSO/H2O of 950/50, 900/100 and 800/200 (μL μL−1). The 5-HMF yield showed a similar trend as mannose conversion, and it kept at 44–56% in the systems of χw ≤ 0.5. A maximum 5-HMF yield of 56% was obtained in DMSO/H2O of 950/50 (μL μL−1) (χw = 0.17). The conversion was also monitored in anhydrous DMSO and DMSO/H2O of 950/50 (μL μL−1) with reaction time at 130 °C. As shown in Fig. S2,† AlCl3·6H2O and AlCl3 showed close activities in DMSO, of which the conversion rate was a little faster than that in DMSO/H2O of 950/50 (μL μL−1) within 20 min. Adding water may improve the hydrolysis of AlCl3·6H2O, but water itself is deleterious to the dehydration of mannose into 5-HMF, accounting for the slower conversion rate in DMSO/H2O of 950/50 (μL μL−1) within short time. As reported, an appropriate amount of water could suppress some side reactions such as inter- and intra-molecular dehydration of sugar to a certain degree,48 leading to an improved 5-HMF yield of 60% as shown in Fig. S2.† Fig. S3† showed the chromatograms on the conversion of mannose in DMSO and DMSO/H2O of 950/50 (μL μL−1) without catalyst at 130 °C for 1 h. The results demonstrated that anhydrous DMSO could promote the formation of mannose dimer, while adding a little water suppressed such side reaction. Compared with anhydrous DMSO system, the completely dissolved AlCl3·6H2O did not significantly increase the yield of 5-HMF. DMSO/H2O binary mixtures display H2O-like properties at χw > 0.5, especially at χw ≥ 0.7,50 which may lead to the inferior mannose conversion and 5-HMF yield in DMSO/H2O of 600/400 (μL μL−1). Fig. S4–S6† present the conversion of mannose with SnCl4·6H2O, CrCl3·6H2O and InCl3·4H2O in varied DMSO/H2O solutions, respectively. However, the addition of water significantly suppressed the formation of 5-HMF with increasing χw in the systems. As compared above, AlCl3·6H2O was chosen as the catalyst for the subsequent investigations and the DMSO/H2O of 950/50 (μL μL−1) solution was further employed.
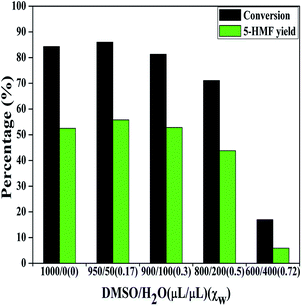 |
| Fig. 2 Catalytic conversion of mannose in varied DMSO/H2O mixed solvents at 130 °C for 30 min. | |
3.3 Effect of catalyst type and loading
A number of aluminum salts were then employed to examine their effects on the conversion of mannose into 5-HMF. In order to maintain a 10 mol% ratio of aluminum atom to mannose, 5 mol% Al2(SO4)3·18H2O was added herein. As seen in Fig. 3a, the tested aluminum salts generally worked well for the production of 5-HMF except Al2(SO4)3·18H2O. Average conversion of ∼80% and 5-HMF yield of ∼45% could be obtained in the presence of Al(NO3)3·9H2O, Al(OTf)3 and AlBr3, respectively. As compared, no improved 5-HMF yield was achieved with other aluminum salts than AlCl3·6H2O. These results indicate that the actual active catalyst enabling the conversion of mannose into 5-HMF should be attributed to an aluminum species. One reason accounting for the inferior performance of Al2(SO4)3·18H2O could be that the active aluminum species as discussed was not effectively produced. Moreover, the steric hindrance of anion should be taken into account as well, while this point needs more in-depth studies.
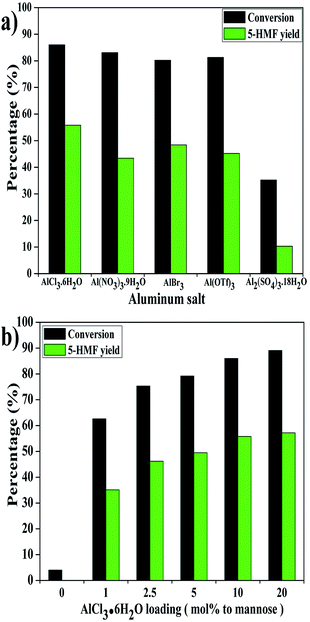 |
| Fig. 3 Effect of aluminum salts (a) and catalyst loadings (b) on the conversion of mannose into 5-HMF at 130 °C for 30 min. | |
Fig. 3b illustrates the conversion of mannose with different AlCl3·6H2O loadings. As shown in Fig. 3b, no 5-HMF was detected in the absence of a catalyst. The mannose conversion (∼3%) was lower than that of blank test (∼5%) in Fig. 1 due to the suppression of water. Only 1 mol% AlCl3·6H2O loading could lead to 63% conversion with a 5-HMF yield of 35%. With increasing catalyst usage, the mannose conversion grew in an upward trend, while the 5-HMF yield was found stagnant at AlCl3·6H2O loading ≥10 mol%. Higher usage of catalyst may accelerate more side reactions or 5-HMF degradation, which may explain why 5-HMF yield stopped increasing. Herein, the AlCl3·6H2O loading of 10 mol% was selected for the next investigation.
3.4 Effect of reaction temperature and time
In order to get an optimized 5-HMF yield, the conversion of mannose was monitored as a function of time at different temperatures. As illustrated in Fig. 4, the conversion of mannose could proceed steadily at 110 °C, reaching 68% with a 5-HMF yield of 44% after 75 min. When the reaction was conducted at 130 °C, the mannose conversion and 5-HMF yield almost reached the maximum of 96% and 60%, respectively, after 45 min. Further elevating temperature to 140 °C did not improve the 5-HMF yield but obviously shortened reaction time to ∼30 min to achieve the optimum. As mentioned above, dehydration of glucose into 5-HMF is considered to undergo a two-step tandem reaction, including the isomerization of glucose into fructose and the dehydration of fructose into 5-HMF.11,20,21 Both the isomerization of glucose and dehydration of fructose are endothermic.51,52 Mannose should have similar thermodynamic properties as glucose based on the fact that it is the C-2 epimer of glucose, which accounts for the significantly accelerated conversion at higher temperatures. The slight decrease of 5-HMF yield at 140 °C indicated that 5-HMF could degrade after long time. Moreover, since it is difficult to separate either catalyst or 5-HMF from the homogeneous system, the catalyst circulation test is outside the scope of this work at present.
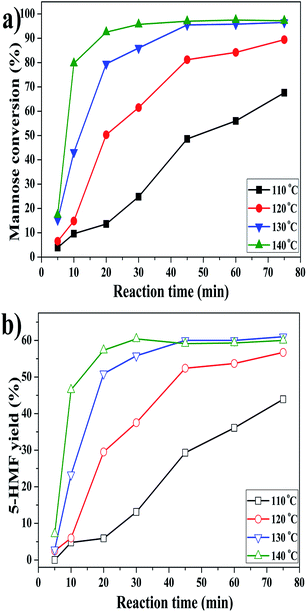 |
| Fig. 4 Effect of reaction temperature and time on the conversion of mannose into 5-HMF in DMSO/H2O of 950/50 (μL μL−1). | |
3.5 Comparison with other saccharides
Fructose and glucose are the isomers of mannose, while both of the two saccharides are the most common carbohydrates for 5-HMF production. We then compared the reactivity of mannose, fructose and glucose. Fig. 5 presents the reactivity comparison in DMSO/H2O of 950/50 (μL μL−1) with AlCl3·6H2O catalyst at 130 °C. As shown, fructose exhibited the fastest reaction rate with 92% conversion and 56% 5-HMF yield after only 20 min, which was consistent with that reported by Guo et al.36 However, mannose showed almost comparable conversion and 5-HMF yield to fructose with extending a bit of time.
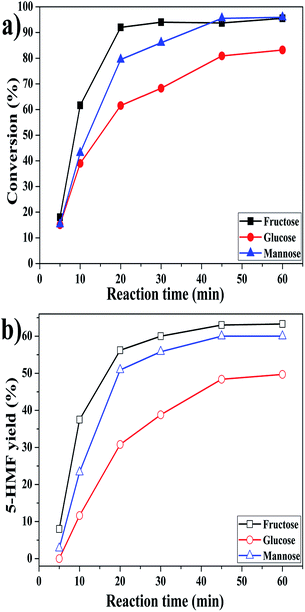 |
| Fig. 5 Comparison on the conversion of mannose, fructose and glucose in DMSO/H2O of 950/50 (μL μL−1) at 130 °C. | |
Disaccharide and trisaccharide were also tested in the system. Fig. 6 illustrates the production of 5-HMF from cellobiose and melezitose over time at 130 °C. Both cellobiose and melezitose produced 5-HMF steadily, achieving a yield of 46% and 54%, respectively, after 120 min. The 5-HMF formation from di/trisaccharide was slower than that from monosaccharide, because the former feedstocks needed to be hydrolyzed first. In addition, melezitose can be partially hydrolyzed to glucose and turanose, the latter of which is an isomer of sucrose.53 As reported, sucrose had a superior reactivity to cellobiose,34,54 which may be one reason that melezitose showed a better performance than cellobiose.
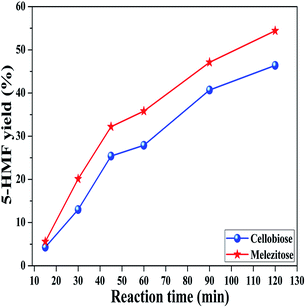 |
| Fig. 6 The production of 5-HMF from cellobiose and melezitose in DMSO/H2O of 950/50 (μL μL−1) at 130 °C. | |
3.6 Mechanistic insights
A number of control experiments were conducted to get some mechanistic insights on the catalytic conversion of mannose. Due to the existence of water, AlCl3·6H2O may undergo hydrolysis into HCl, aluminum complexes and Al(OH)3. To clarify which component catalyzed the conversion, both HCl and Al(OH)3 were tested as the catalyst, respectively. It was assumed that AlCl3·6H2O (10 mol% to mannose) completely hydrolyzed, then the amount of formed HCl should be 30 mol% to mannose. As listed in Table 1 (entry 2), HCl promoted the conversion of mannose. However, both HCl and Al(OH)3 were inactive to the formation of 5-HMF (entries 2 and 3). Vlachos et al. and Hu et al. demonstrated that AlCl3 could hydrolyze into an active aluminum complex, Al(OH)2+ or Al(OH)2+, which played a role in isomerizing glucose.45–47 As a result, the active aluminium complex was also proposed to be the key species for converting mannose into 5-HMF. As given in entry 4, the performance of mannose conversion appeared to be similar to the one with HCl alone, possibly because the active aluminium complex was not formed to an appreciable extent under this condition. Partial hydrolysis of AlCl3·6H2O could produce HCl and aluminum complexes as the products. As seen in entries 5–7 (Table 1), the 5-HMF yield significantly decreased with increasing the usage of HCl, even though the reaction time was extended. Adding HCl could suppress the hydrolysis of AlCl3·6H2O, leading to less aluminum complexes, which further verified that aluminum complexes such as Al(OH)2+ or Al(OH)2+ should be the actual active catalyst.
Table 1 Results on the control experimentsa
Entry |
Catalyst |
Additived |
Conversion (%) |
5-HMF yield (%) |
Conditions: 60 mg of mannose with catalyst (10 mol% to mannose) was added into total 1 mL of DMSO/H2O (950/50, (μL μL−1)) mixed solvent and heated at 130 °C for 20 min. 30 mol% (to mannose) HCl was added. 30 mol% (to mannose) HCl and 10 mol% (to mannose) Al(OH)3 were added. The number in the brackets represents the dosage of additive (with respect to mannose by mol). The results were obtained after 30 min. |
1 |
AlCl3·6H2O |
None |
80 |
51 |
2b |
HCl |
None |
48 |
6 |
3 |
Al(OH)3 |
None |
5 |
0 |
4c |
HCl + Al(OH)3 |
None |
47 |
5 |
5 |
AlCl3·6H2O |
HCl (10 mol%) |
46/68e |
25/38e |
6 |
AlCl3·6H2O |
HCl (20 mol%) |
50/71e |
15/26e |
7 |
AlCl3·6H2O |
HCl (30 mol%) |
50/66e |
11/17e |
8 |
AlCl3·6H2O |
Glycerol (100 mol%) |
80 |
52 |
9 |
AlCl3·6H2O |
Glyceraldehyde (100 mol%) |
56 |
36 |
10 |
AlCl3·6H2O |
Dihydroxyacetone (100 mol%) |
51 |
32 |
Zhang et al. indicated that the open chain glucose could be considered as a glyceraldehyde attached to a glycerol.11 The catalyst may coordinate with the glyceraldehyde subunit of glucose to achieve its isomerization into fructose.11,55 Because mannose is the C-2 epimer of glucose, likewise, we think that it is comprised of glyceraldehyde and glycerol subunits. Then, both glyceraldehyde and glycerol were employed as the additives for mannose conversion. As shown in entries 8 and 9 (Table 1), glycerol did not substantially affect the conversion of mannose as compared with entry 1. However, glyceraldehyde did suppress the formation of 5-HMF, indicating that the catalyst coordinated with the glyceraldehyde part of mannose. 1,3-Dihydroxyacetone could be produced as an isomerization product from glyceraldehyde. Next, 1,3-dihydroxyacetone was also tested as an additive. As shown in entry 10 (Table 1), 1,3-dihydroxyacetone suppressed the production of 5-HMF as well. As mentioned above, fructose can also be considered as a 1,3-dihydroxyacetone attached to a glycerol. This result may imply that catalyst promote the conversion of fructose, derived from mannose isomerization, by interacting with 1,3-dihydroxyacetone subunit of fructose.
4. Conclusions
In this work, we demonstrated that mannose could be effectively converted into 5-HMF in a DMSO/H2O mixed solvent under mild conditions. AlCl3·6H2O exhibited the superior activity among the tested catalysts. Adding an appropriate amount of water in DMSO can suppress some side reactions while preserving the reactivity of mannose dehydration. Mannose showed a comparable reactivity to that of fructose in this catalytic system. A maximum 5-HMF yield of 60% could be obtained at 130 °C within 45 min. The studied system was also effective for the conversion of other di/trisaccharides into 5-HMF. An active aluminum species from the hydrolysis of AlCl3·6H2O was turned out to play a key role in converting mannose into 5-HMF. The active aluminum species primarily interacted with the glyceraldehyde subunit of mannose and 1,3-dihydroxyacetone subunit of fructose to achieve the final formation of 5-HMF. Mannose is a major sugar component of hemicellulose. The results provided herein will offer useful information on the valorization of hemicellulose and even lignocellulose in the future.
Acknowledgements
The work was supported by the Natural Science Foundation of Liaoning Province (China) (No. 2015020633), Project of Education Department of Liaoning Province (China) (No. L2015421), the National Natural Science Foundation of China (No. 21306186).
References
- R. van Putten, J. C. van der Waal, E. de Jong, C. B. Rasrendra, H. J. Heeres and J. G. de Vries, Chem. Rev., 2013, 113, 1499–1597 CrossRef CAS PubMed.
- W. Gong, K. Zheng and P. Ji, RSC Adv., 2017, 7, 34776–34782 RSC.
- Z. Xu, P. Yan, H. Li, K. Liu, X. Liu, S. Jia and Z. C. Zhang, ACS Catal., 2016, 6, 3784–3788 CrossRef CAS.
- Z. Wang and Q. Chen, Green Chem., 2016, 18, 5884–5889 RSC.
- Z. Xu, P. Yan, W. Xu, S. Jia, Z. Xia, B. Chung and Z. C. Zhang, RSC Adv., 2014, 4, 59083–59087 RSC.
- H. Li, Z. Xu, P. Yan and Z. C. Zhang, Green Chem., 2017, 19, 1751–1756 RSC.
- Q. Deng, J. Xu, P. Han, L. Pan, L. Wang, X. Zhang and J. Zou, Fuel Process. Technol., 2016, 148, 361–366 CrossRef CAS.
- P. Han, G. Nie, J. Xie, X. E, L. Pan, X. Zhang and J. Zou, Fuel Process. Technol., 2017, 163, 45–50 CrossRef CAS.
- B. F. M. Kuster, Starch/Staerke, 1990, 42, 314–321 CrossRef CAS.
- Y. Román-Leshkov, J. N. Chheda and J. A. Dumesic, Science, 2006, 312, 1933–1937 CrossRef PubMed.
- H. Zhao, J. E. Holladay, H. Brown and Z. C. Zhang, Science, 2007, 316, 1597–1600 CrossRef CAS PubMed.
- S. J. Angyal, Adv. Carbohydr. Chem. Biochem., 1984, 42, 63–65 CrossRef.
- Y. J. Pagán-Torres, T. Wang, J. M. R. Gallo, B. H. Shanks and J. A. Dumesic, ACS Catal., 2012, 2, 930–934 CrossRef.
- D. Garcés, E. Díaz and S. Ordóñez, Ind. Eng. Chem. Res., 2017, 56, 5221–5230 CrossRef.
- F. N. D. C. Gomes, F. M. T. Mendes and M. M. V. M. Souza, Catal. Today, 2017, 279, 296–304 CrossRef CAS.
- X. Zhou, Z. Zhang, B. Liu, Q. Zhou, S. Wang and K. Deng, J. Ind. Eng. Chem., 2014, 20, 644–649 CrossRef CAS.
- Z. Ma, H. Hu, Z. Sun, W. Fang, J. Zhang, L. Yang, Y. Zhang and L. Wang, ChemSusChem, 2017, 10, 1669–1674 CrossRef CAS PubMed.
- H. Guo, X. Qi, Y. Hiraga, T. M. Aida and R. L. Smith Jr, Chem. Eng. J., 2017, 314, 508–514 CrossRef CAS.
- W. Li, T. Zhang, H. Xin, M. Su, L. Ma, H. Jameel, H. Chang and G. Pei, RSC Adv., 2017, 7, 27682–27688 RSC.
- Y. Zhang, E. A. Pidko and E. J. M. Hensen, Chem.–Eur. J., 2011, 17, 5281–5288 CrossRef CAS PubMed.
- E. A. Pidko, V. Degirmenci, R. A. van Santen and E. J. M. Hensen, Angew. Chem., Int. Ed., 2010, 49, 2530–2534 CrossRef CAS PubMed.
- S. Hu, Z. Zhang, J. Song, Y. Zhou and B. Han, Green Chem., 2009, 11, 1746–1749 RSC.
- Y. Yang, C. Hu and M. M. Abu-Omar, J. Mol. Catal. A: Chem., 2013, 376, 98–102 CrossRef CAS.
- Z. Zhang, Q. Wang, H. Xie, W. Liu and Z. K. Zhao, ChemSusChem, 2011, 4, 131–138 CrossRef CAS PubMed.
- C. Antonetti, M. Melloni, D. Licursi, S. Fulignati, E. Ribechini, S. Rivas, J. C. Parajó, F. Cavani and A. M. R. Galletti, Appl. Catal., B, 2017, 206, 364–377 CrossRef CAS.
- C. Antonetti, A. M. R. Galletti, S. Fulignati and D. Licursi, Catal. Commun., 2017, 97, 146–150 CrossRef CAS.
- C. Chiappe, M. J. R. Douton, A. Mezzetta, C. S. Pomelli, G. Assanelli and A. R. de Angelis, ACS Sustainable Chem. Eng., 2017, 5, 5529–5536 CrossRef CAS.
- H. Shirai, S. Ikeda and E. W. Qian, Fuel Process. Technol., 2017, 159, 280–286 CrossRef CAS.
- https://en.wikipedia.org/wiki/Hemicellulose, accessed on 2017.07.07.
- T. Marzialetti, M. B. V. Olarte, C. Sievers, T. J. C. Hoskins, P. K. Agrawal and C. W. Jones, Ind. Eng. Chem. Res., 2008, 47, 7131–7140 CrossRef CAS.
- J. B. Binder, A. V. Cefali, J. J. Blank and R. T. Raines, Energy Environ. Sci., 2010, 3, 765–771 CAS.
- S. De, S. Dutta, A. K. Patra, B. S. Rana, A. K. Sinha, B. Saha and A. Bhaumik, Appl. Catal., A, 2012, 435–436, 197–203 CrossRef CAS.
- P. Wrigstedt, J. Keskiväli and T. Repo, RSC Adv., 2016, 6, 18973–18979 RSC.
- P. Bhanja, A. Modak, S. Chatterjee and A. Bhaumik, ACS Sustainable Chem. Eng., 2017, 5, 2763–2773 CrossRef CAS.
- T. Flannelly, M. Lopes, L. Kupiainen, S. Dooley and J. J. Leahy, RSC Adv., 2016, 6, 5797–5804 RSC.
- J. Guo, S. Zhu, Y. Cen, Z. Qin, J. Wang and W. Fan, Appl. Catal., B, 2017, 200, 611–619 CrossRef CAS.
- T. Deng, X. Cui, Y. Qi, Y. Wang, X. Hou and Y. Zhu, Chem. Commun., 2012, 48, 5494–5496 RSC.
- T. Ståhlberg, M. G. Sørensen and A. Riisager, Green Chem., 2010, 12, 321–325 RSC.
- Y. Shen, J. Sun, Y. Yi, B. Wang, F. Xu and R. Sun, J. Mol. Catal. A: Chem., 2014, 394, 114–120 CrossRef CAS.
- T. Ståhlberg, S. Rodriguez-Rodriguez, P. Fristrup and A. Riisager, Chem.–Eur. J., 2011, 17, 1456–1464 CrossRef PubMed.
- F. Tao, H. Song, J. Yang and L. Chou, Carbohydr. Polym., 2011, 85, 363–368 CrossRef CAS.
- F. Tao, H. Song and L. Chou, J. Mol. Catal. A: Chem., 2012, 357, 11–18 CrossRef CAS.
- Z. Ding, J. Shi, J. Xiao, W. Gu, C. Zheng and H. Wang, Carbohydr. Polym., 2012, 90, 792–798 CrossRef CAS PubMed.
- J. Heltzel, S. K. R. Patil and C. R. F. Lund, in Reaction Pathways and Mechanisms in Thermocatalytic Biomass Conversion II, ed. M. Schlaf and Z. C. Zhang, Springer, 2016, pp. 105–118 Search PubMed.
- V. Choudhary, S. H. Mushrif, C. Ho, A. Anderko, V. Nikolakis, N. S. Marinkovic, A. I. Frenkel, S. I. Sandler and D. G. Vlachos, J. Am. Chem. Soc., 2013, 135, 3997–4006 CrossRef CAS PubMed.
- J. Tang, X. Guo, L. Zhu and C. Hu, ACS Catal., 2015, 5, 5097–5103 CrossRef CAS.
- J. Tang, L. Zhu, X. Fu, J. Dai, X. Guo and C. Hu, ACS Catal., 2017, 7, 256–266 CrossRef CAS.
- S. Jia, Z. Xu and Z. C. Zhang, Chem. Eng. J., 2014, 254, 333–339 CrossRef CAS.
- Y. Koga, Y. Kasahara, K. Yoshino and K. Nishikawa, J. Solution Chem., 2001, 30, 885–893 CrossRef CAS.
- N. Zhang, W. Li, C. Chen and J. Zuo, Comput. Theor. Chem., 2013, 1017, 126–135 CrossRef CAS.
- Y. B. Tewari, Appl. Biochem. Biotechnol., 1990, 23, 187–203 CrossRef CAS PubMed.
- R. S. Assary, P. C. Redfern, J. R. Hammond, J. Greeley and L. A. Curtiss, J. Phys. Chem. B, 2010, 114, 9002–9009 CrossRef CAS PubMed.
- https://en.wikipedia.org/wiki/Melezitose, accessed on 2017.07.07.
- Y. Qu, L. Li, Q. Wei, C. Huang, P. Oleskowicz-Popiel and J. Xu, Sci. Rep., 2016, 6, 26067 CrossRef PubMed.
- S. Jia, K. Liu, Z. Xu, P. Yan, W. Xu, X. Liu and Z. C. Zhang, Catal. Today, 2014, 234, 83–90 CrossRef CAS.
Footnote |
† Electronic supplementary information (ESI) available. See DOI: 10.1039/c7ra07803j |
|
This journal is © The Royal Society of Chemistry 2017 |