DOI:
10.1039/C7RA06755K
(Paper)
RSC Adv., 2017,
7, 40367-40370
Deep eutectic solvents as performance additives in biphasic reactions†
Received
17th June 2017
, Accepted 11th August 2017
First published on 18th August 2017
Abstract
Deep eutectic solvents act as surfactants in biphasic (hydrophobic/aqueous) reaction mixtures enabling higher interfacial surface areas at lower mechanical stress as compared to simple emulsions. Exploiting this effect the rate of a chemoenzymatic epoxidation reaction was increased more than six-fold.
Introduction
The potential of biocatalysis for better environmentally acceptable chemical production is widely recognised today.1–3 However, the majority of industrially relevant products are rather hydrophobic by nature, which poses a significant challenge to biocatalysis, which generally occurs in aqueous reaction media. Hence, many biocatalytic reactions are performed in aqueous media using rather low concentrations of the starting material (frequently in the lower millimolar range). As a consequence the economic interest in such biocatalytic reactions is low because of the environmental impact of such reactions tends to be high.1 Therefore, one of the major challenges faced in biocatalysis is to bridge the gap between hydrophobic reagents and hydrophilic reaction conditions. For this reason, the two liquid phase (2LP) approach was used very often in the past.4,5 Here, a hydrophobic organic solvent serves as substrate reservoir and product sink enabling overall high reagent loadings and also circumventing aqueous side reactions such as hydrolysis or further reactions of the desired products.6–8
Such heterogeneous reaction mixtures, however, are often kinetically hampered by phase transfer rates. i.e. the rate of diffusion of the starting material from the organic phase into the aqueous reaction phase becomes overall rate-limiting. Therefore, increasing the interfacial area between the hydrophobic and the aqueous phase is essential in maximising diffusion rates.
More recently, deep eutectic solvents (DES) have attracted much attention due to their advantage of facile preparation, high biodegradability and low toxicity.9 Interestingly, higher catalytic efficiencies of several kind of enzymes tested were achieved in DES compared to the normal solvent.10–12 It has been established in the past that various deep eutectic solvents such as, choline chloride/urea and choline chloride/glycerol, are able to reduce the surface tension of water in organic-aqueous 2LPS.13 However, to the best of our knowledge, practical implementation with biocatalytic reactions has not been implemented yet. Therefore, we became interested in evaluating the effect of DES on a biocatalytic 2LPS reaction. As a model reaction we chose the chemoenzymatic epoxidation of soybean oil (SBO) using Penicillium camemberti lipase (PCL, Scheme 1). In this reaction aqueous hydrogen peroxide is used to in situ generate peracids from free fatty acid ‘impurities’ within the SBO.14,15 These peracids then spontaneously perform a Prilezhaev-type epoxidation on the C
C-double bonds within SBO. PCL was chosen as catalyst for the perhydrolysis reaction due to its inactivity towards triglycerides,16,17 thereby circumventing any undesired hydrolysis of the SBO. The resulting epoxidised SBO is considered as a promising substitute for phthalates as plasticiser.18,19
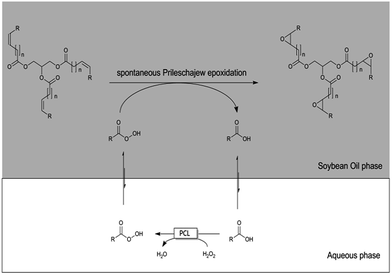 |
| Scheme 1 Chemoenzymatic epoxidation of SBO in 2LP reaction mixture. SBO forms the organic layer. Free carboxylic acids are present in the SBO partition between the oil and the aqueous layer (containing H2O2 and enzyme) wherein they are transformed into peracids. The latter partition between both layers and mediate the Prileschajew epoxidation of the SBO. | |
Experimental sections
Materials
Penicillium camemberti lipase (PCL) was obtained from Amano pharmaceutical Co., Ltd (Nagoya, Japan). SBO was supplied by Kerry Oils & Grains Ltd. (Shenzhen, China). Hydrogen peroxide (30%, w/w), choline chloride, urea, glycerol, sorbitol and xylitol were purchased from Aladdin Chemistry Co., Ltd (Shanghai, China). All other reagents were analytical grade.
Preparation of DESs
DESs were prepared in different molar ratios of choline chloride to hydrogen bone donors (glycerol, urea, xylitol and sorbitol) as shown in Table 1. The eutectic mixtures were obtained by rotary evaporation of the two components at 100 °C in water bath until a homogeneous transparent liquid was formed.20
Table 1 Choline chloride based deep eutectic solvents and their components
Entry |
Hydrogen bond acceptor |
Hydrogen bond donor |
Molar ratio |
1 |
Choline chloride |
Urea |
1 : 2 |
2 |
Glycerol |
1 : 1 |
3 |
Xylitol |
1 : 1 |
4 |
Sorbitol |
1 : 1 |
Epoxidation reaction
Reactions were performed in a 10 mL Erlenmeyer flask at 40 °C with magnetic stirring (500 rpm). The reaction mixture contained SBO (3.0 g), hydrogen peroxide (30%, 1.2 mL), PCL (300 mg) and DESs (4.26 g). Sample from reaction without adding DESs was used as control. Reactants was withdrawn at time intervals, and the oxirane oxygen contents of epoxidized HBO were measured according to the previous described method with minor modification.21 In briefly, 0.1 g epoxy oil was obtained from upper phase of the reactant sample after centrifugation with a speed of 12
000 rpm. And then it was dissolved in 5 mL chloroform and titrated against 0.1 N HBr in a glacial acetic acid solution, and crystal violet was used as an indicator. The solution would turn as bluish-green colour at the end point. The oxirane oxygen content was calculated using equation below:
where, L is the volume of HBr solution (mL), N is the normality of HBr solution and W is the mass of the sample (g).
The conversion of unsaturated to epoxy groups was determined by the following expression:22
where, OO
e is the experimental oxirane oxygen; OO
t, theoretical maximum oxirane oxygen, which was determined to be 7.64% from the followed equation:
where,
Ai (126.9) and
Ao (16.0) are the molecular weight of iodine and oxygen, respectively and IV
0 is the initial iodine value of the SBO sample.”
General analysis method
Surface tension measurements
The interfacial tensions of oil/water or containing DESs (30%, 50% and 70% choline chloride/sorbitol) solution without adding PCL lipase were determined with the plate method using a DCAT 21 tensiometer (Dataphysics, Germany) and the temperature was set as 25.0 ± 0.1 °C. The interfacial tensions of each sample were continuously recorded for 3000 seconds (result was shown by Fig. S1 in ESI†).
Confocal microscopy analysis
Microstructure of oil/water or containing DESs droplets were investigated using laser scanning confocal microscopy (LSCM, Leica, Germany). Nile red was used as oil-phase marker and the excitation wavelength was set as 553 nm. All sample were agitated for at least 15 min before the observations. A drop of the stained sample was placed on a concave confocal microscope 1.2 mm slide, and then typical images were obtained.
Droplet size analysis
The size of oil droplets were analyzed by using a Mastersizer 2000 laser diffractometer with the Hydrosizer 2000S module (Malvern Instruments, UK). The SBO was extemporaneously dispersed in water (or containing DESs with various contents) at 500 rpm until an obscuration rate of 10–20% was obtained. Then, the droplet size distributions (d3,2 and d4,3) were investigated at 30 min. Each sample was measured in triplicate.
Result and discussion
In a first set of experiments, we evaluated a range of different DES for the chemoenzymatic epoxidation reaction. As shown in Fig. 1, sugar-based DES excelled in their performance enabling roughly ten-fold increased reaction rates as compared to aqueous reaction conditions. And single component of DES did not show similar beneficial effect on enhancing the catalytic efficiency as that of DES (Fig. S2 in ESI†).
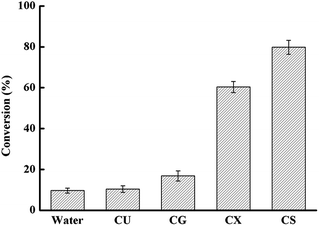 |
| Fig. 1 Chemoenzymatic epoxidation of SBO in different reaction media. General conditions: reactions were performed in a 10 mL Erlenmeyer flask at 40 °C for 12 h with magnetic stirring (500 rpm). The reaction mixture contained SBO (3.0 g), hydrogen peroxide (30%, 1.2 mL), PCL (300 mg) and DES (4.26 g). CU: choline chloride/urea, CG: choline chloride/glycerol, CX: choline chloride/xylitol, CS: choline chloride/sorbitol. | |
Encouraged by these results, we investigated the DES composed of choline chloride (ChCl) and sorbitol in more detail. As shown in Fig. 2, the ChCl/sorbitol content had a significant influence on the rate and final yield of the chemoenzymatic epoxidation reaction.
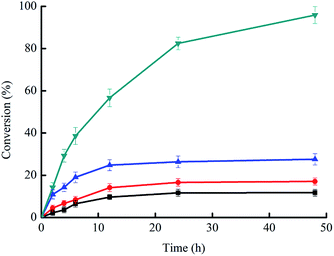 |
| Fig. 2 Time courses of the chemoenzymatic epoxidation of SBO to epoxy SBO in water with various content of ChCl/sorbitol 0% ( ), 30% ( ), 50% ( ) or 70% ( ). General conditions: reactions were performed in a 10 mL Erlenmeyer flask at 40 °C for 48 h with magnetic stirring (500 rpm). The reaction mixture contained SBO (3.0 g), hydrogen peroxide (30%, 1.2 mL), PCL (300 mg) and ChCl/sorbitol DES (4.26 g). | |
We estimated the catalytic performance of PCL to increase from roughly 3 min−1 (in case of the aqueous only reaction conditions) to more than 19 min−1 in case of 70% ChCl/sorbitol. At the same time, also the final conversion increased from 12% to more than 95%. In terms of waste generated, this also corresponds to a reduction of the E-factor2 from 23.4 gwaste gproduct−1 (in case of the aqueous conditions) to approx. 2 gwaste gproduct−1 as in case of 70% ChCl/sorbitol.
We suspected the interfacial area size to be the most decisive factor for this observation and therefore determined the average size distribution of the SBO droplets in the reaction system using laser diffraction (Fig. 3).
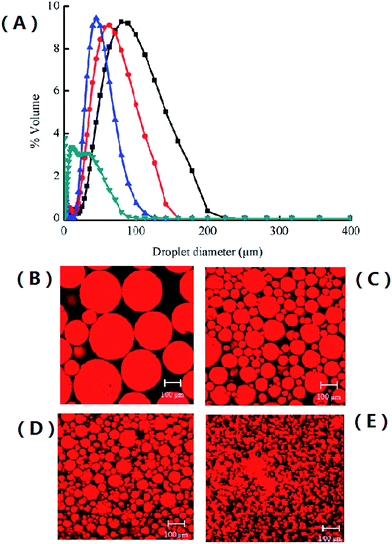 |
| Fig. 3 Droplet size and distribution of SBO in water containing ChCl/sorbitol DES. (A) 0% ( ), 30% ( ), 50% ( ) and 70% ( ). Typical confocal laser scanning microscopy images of the SBO emulsions with various content of ChCl/sorbitol (B–E) 0% (B), 30% (C), 50% (D) and 70% (E). | |
Indeed, there was a good correlation between the mean average size of the droplets and the initial rate of the chemoenzymatic epoxidation reaction. Plotting the droplet surface (calculated with formula of S = 4πR2) against the initial rate gave an almost linear relationship. Therefore we conclude that the interfacial surface area correlates directly with the overall rate of the reaction. It is worth mentioning here that this effect can be attributed to a reduction of the surface tension of the SBO droplets in the aqueous reaction system (Fig. 4).
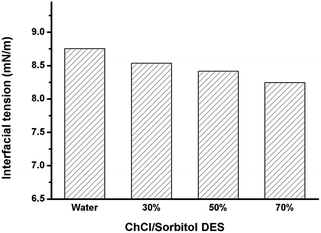 |
| Fig. 4 Interfacial tension of SBO in water containing various content of ChCl/sorbitol DES (0, 30, 50 and 70%). Interfacial tension of each sample after 3000 second were shown. | |
To rationalise the correlation between surface area and productivity of the 2LPS two factors should be taken into account: first, as interfacially activated enzymes lipases exhibit significantly higher activity if situated at the interface.23 Hence, increasing the relative surface area will also increase the concentration of active lipases. Second, also faster partitioning of the reagents between both phases (due to a higher surface area) effects the overall rate of a diffusion-limited reaction. Further studies dissecting both effects are currently underway.
Finally, it is also worth mentioning here that reduction of the interfacial surface tension also results in less mechanical force necessary to obtain emulsions which will reduce the mechanical stress exceeded on the biocatalysts hence achieving more robust biocatalytic reactions. However, by adding some traditional surfactants into the reaction, the catalytic efficiency of our reactions have not been obviously elevated, even that they would have negative effect on the reaction process (Fig. S2 in ESI†).
Conclusions
Overall, in this study we have demonstrated that stable micro emulsions can be obtained by simple addition of sugar-based DES. These ‘performance-additives’ efficiently lower the surface tension of hydrophobic organic phases in aqueous reaction media and thereby enable more efficient biphasic biocatalytic reactions.
Taking the beneficial effect of DES on enzyme stability15,24,25 into account we are very confident that this concept may represent a future-pointing approach for biocatalysis by achieving more robust (due to the stabilizing effect) and more efficient (due to the increased surface area in 2LPSs) reactions and thereby make biocatalytic transformations of hydrophobic starting materials more attractive and environmentally more acceptable. Extension of this approach to other enzyme reactions is currently ongoing in our laboratory.
Conflicts of interest
There are no conflicts to declare.
Acknowledgements
This work was supported by the National Natural Science Foundation of China (31471690 and 21406076), and Science and Technology Planning project of Guangdong province (2014B020204003, 2015B020231006).
Notes and references
- Y. Ni, D. Holtmann and F. Hollmann, ChemCatChem, 2014, 6, 930–943 CrossRef CAS.
- R. A. Sheldon, Green Chem., 2017, 19, 18–43 RSC.
- M. T. Reetz, J. Am. Chem. Soc., 2013, 135, 12480–12496 CrossRef CAS PubMed.
- Z. Yang and W. B. Pan, Enzyme Microb. Technol., 2005, 37, 19–28 CrossRef CAS.
- Y. L. Gu and F. Jerome, Chem. Soc. Rev., 2013, 42, 9550–9570 RSC.
- B. Buhler, I. Bollhalder, B. Hauer, B. Witholt and A. Schmid, Biotechnol. Bioeng., 2003, 81, 683–694 CrossRef CAS PubMed.
- E. Churakova, I. W. C. E. Arends and F. Hollmann, ChemCatChem, 2013, 5, 565–568 CrossRef CAS.
- Y. Ni, P. Hagedoorn, J. Xu, I. W. C. E. Arends and F. Hollmann, Chem. Commun., 2012, 48, 12056–12058 RSC.
- Y. Dai, J. van Spronsen, G. J. Witkamp, R. Verpoorte and Y. H. Choi, Anal. Chim. Acta, 2013, 766, 61–68 CrossRef CAS PubMed.
- A. A. Papadopoulou, E. Efstathiadou, M. Patila, A. C. Polydera and H. Stamatis, Ind. Eng. Chem. Res., 2016, 55, 5145–5151 CrossRef CAS.
- C. X. Zeng, S. J. Qi, R. P. Xin, B. Yang and Y. H. Wang, Bioprocess Biosyst. Eng., 2015, 38, 2053–2061 CrossRef CAS PubMed.
- H. Zhao, G. A. Baker and S. Holmes, J. Mol. Catal. B: Enzym., 2011, 72, 163–167 CrossRef CAS PubMed.
- M. K. Hadj-Kali, K. E. Al-khidir, I. Wazeer, L. El-blidi, S. Mulyono and I. M. AlNashef, Colloids Surf., A, 2015, 487, 221–231 CrossRef CAS.
- T. Vlcek and Z. S. Petrovic, J. Am. Oil Chem. Soc., 2006, 73, 247–252 CrossRef.
- P. F. Zhou, X. P. Wang, C. X. Zeng, W. F. Wang, B. Yang, F. Hollmann and Y. H. Wang, ChemCatChem, 2017, 9, 934–936 CrossRef CAS.
- Q. Y. Tang, G. M. Popowicz, X. P. Wang, J. S. Liu, I. V. Pavlidis and Y. G. Wang, ChemistrySelect, 2016, 1, 836–839 CrossRef CAS.
- P. Y. Zheng, Y. Xu, W. F. Wang, X. L. Qin, Z. X. Ning, Y. H. Wang and B. Yang, J. Am. Oil Chem. Soc., 2014, 91, 251–259 CrossRef CAS.
- Z. Xiong, Y. Yang, J. X. Feng, X. M. Zhang, C. Z. Zhang, Z. B. Tang and J. Zhu, Carbohydr. Polym., 2013, 92, 810–816 CrossRef CAS PubMed.
- D. Yang, X. W. Peng, L. X. Zhong, X. F. Cao, W. Chen, X. M. Zhang, S. J. Liu and R. C. Sun, Carbohydr. Polym., 2014, 103, 198–206 CrossRef CAS PubMed.
- K. Xu, Y. Wang, Y. Huang, N. Li and Q. Wen, Anal. Chim. Acta, 2015, 864, 9–20 CrossRef CAS PubMed.
- C. Paquot, Standard methods for the analysis of oils, fats and derivatives, Elsevier, 2013 Search PubMed.
- V. V. Goud, N. C. Pradhan and A. V. Patwardhan, J. Am. Oil Chem. Soc., 2006, 83, 635–640 CrossRef CAS.
- Y. Cajal, A. Svendsen, J. De Bolos, S. A. Patkar and M. A. Alsina, Biochimie, 2000, 82, 1053–1061 CrossRef CAS PubMed.
- Z. L. Huang, B. P. Wu, Q. Wen, T. X. Yang and Z. Yang, J. Chem. Technol. Biotechnol., 2014, 89, 1975–1981 CrossRef CAS.
- P. F. Zhou, X. P. Wang, B. Yang, F. Hollmann and Y. H. Wang, RSC Adv., 2017, 7, 12518–12523 RSC.
Footnote |
† Electronic supplementary information (ESI) available. See DOI: 10.1039/c7ra06755k |
|
This journal is © The Royal Society of Chemistry 2017 |
Click here to see how this site uses Cookies. View our privacy policy here.