DOI:
10.1039/C7RA06599J
(Paper)
RSC Adv., 2017,
7, 36852-36859
Enhanced hydrogen storage properties of a dual-cation (Li+, Mg2+) borohydride and its dehydrogenation mechanism†
Received
13th June 2017
, Accepted 21st July 2017
First published on 25th July 2017
Abstract
In this paper, we present a new method to synthesize a dual-cation (Li+, Mg2+) borohydride. It is found that Li–Mg–B–H is formed by mechanical milling a mixture of LiBH4 and MgCl2 with a molar ratio of 3
:
1 in diethyl ether (Et2O) and a subsequent heating process. The morphology and structure of the as-prepared Li–Mg–B–H compound are studied by SEM, XRD, FTIR and NMR measurements. Further experiments testify that Li–Mg–B–H can release approximately 12.3 wt% of hydrogen under 4 bar initial hydrogen pressure from room temperature to 500 °C and reach a maximum desorption rate of 13.80 wt% per h at 375 °C, which is 30 times faster than that of pristine LiBH4. Thermal analysis indicates that the decomposition process of the new compound involves three steps: (1) Li–Mg–B–H first decomposes into LiBH4 and MgH2 and synchronously releases a number of H2 molecules; (2) MgH2 decomposes to Mg and H2; (3) LiBH4 reacts with Mg, generating H2, MgB2 and LiH. Moreover, Li–Mg–B–H is proved to be partially reversible, which can release 5.3 wt% hydrogen in the second dehydrogenation process. The strategy of altering the χp of metal ions in borohydrides may shed light on designing dual-cation borohydrides with better hydrogen storage performance.
Introduction
Hydrogen, which produces nearly zero pollutant emission from power generators, is regarded as one of the most promising cost-effective and renewable energy carriers during past decades. However, a safe hydrogen storage technology with a high energy density still challenges scientists worldwide.1,2 LiBH4, which has high gravimetric and volumetric hydrogen densities (18.5 wt% and 121 kg·H2 per m3), is regarded as one of the most promising hydrogen storage materials.3,4 Nevertheless, LiBH4 is thermodynamically stable and kinetically sluggish in dehydrogenation. Besides, extremely rigorous temperature and pressure conditions are required for LiBH4 to re-form, which severely limits its practical on-board automobile application.
In past decades, numerous attempts have been carried out to destabilize LiBH4, including catalyst doping,5–10 reactive composite formation,11–16 nanoconfinement17,18 and a combination of strategies.19–26 Recently, Orimo et al. found that the thermodynamic stability of ionic borohydrides can be correlated fairly well with the Pauling electronegativity χp of metal ions, Mn+; the higher χp of Mn+, the less stable M(BH4)n will be.27,28 This finding suggests that the thermodynamic stability of LiBH4 can be tuned by using metal ions Mn+ with higher χp to partially substitute the Li cations to form a dual-cation borohydride LiM(BH4)n+1. Employment of this strategy has yielded several novel dual-cation borohydrides. Jiang et al.29 successfully synthesized a new Li–Ca–B–H complex borohydride with its first dehydrogenation step started at ca.70 °C, much lower than those of pristine LiBH4 and Ca(BH4)2. Choudhury et al.26 prepared a new complex hydride LiMn(BH4)3 with a 3
:
1 ratio of precursor materials LiBH4 and MnCl2 via the solid-state mechano-chemical process. Thermogravimetric analysis (TGA) of LiMn(BH4)3 indicated that ca. 8.0 wt% hydrogen can be released between 135 °C and 155 °C in a single dehydrogenation step. Kim et al.30 found that ball milling LiBH4 and ScCl3 produced LiCl and a unique crystalline hydride, which has been unequivocally identified via multinuclear solid-state nuclear magnetic resonance (NMR) to be LiSc(BH4)4. Fang et al.31 claimed that they had synthesized a dual-cation borohydride directly from LiBH4 and Mg(BH4)2 with molar ratio of 1
:
1. Rapid hydrogen release from the LiBH4/Mg(BH4)2 sample was initiated at around 240 °C, which is about 30 °C and 170 °C lower than that of LiBH4 and Mg(BH4)2. However, Bardají et al.32 investigated the physical mixture of xLiBH4(1−x)Mg(BH4)2 with x = 0, 0.10, 0.25, 0.33, 0.40, 0.50, 0.60, 0.66, 0.75, 0.80, 0.90, 1 and found it was only a physical mixture but not a dual-cation borohydride. The eutectic composition was found to exist at 0.50 < x < 0.60 exhibiting a eutectic melting at 180 °C and the decomposition of the material begins right after the melting. At 270 °C the x = 0.50 composite release about 7.0 wt% of hydrogen. Therefore no confirmed synthesis of dual-cation LiMg(BH4)3 has been reported yet.
Inspired by the theory and experiments above, it seems that thermodynamic stability of LiBH4 can be decreased by introducing metal ions with higher χp. Besides, the most feasible way to synthesize a dual-cation borohydride is milling LiBH4 with metal chloride. In this paper, we focus on the Li–Mg–B–H system, aiming to elucidate the possible formation of a dual-cation borohydride via wet-milling LiBH4 and MgCl2 in Et2O. The samples are carefully characterized and determined by the SEM, XRD, FTIR and NMR measurements, and their hydrogen storage properties have been investigated.
Experimental section
All sample operations were performed in an Ar-filled glovebox, which was equipped with a circulative purification system to maintain the H2O and O2 levels below 0.1 ppm. LiBH4 (assay 95%, Alfa Aesar), NaBH4 (96%, Sinopharm Chemical Reagent co., Ltd) and MgCl2 (assay 98%, Sigma) were used as starting materials. Et2O (C4H10, 99.5%, Hangzhou Chemical Reagent co., Ltd) was used as solvent. 2 g mixture of LiBH4 and MgCl2 in a molar ratio of 3
:
1 together with 60 mL of Et2O was put into a stainless steel vial with a ball-to-power ratio of 20
:
1. The ball milling process was carried out on a planetary ball mill (QM-3SP4, Nanjing, China) under 1 MPa high purity H2 (99.999%) at a speed of 400 rpm. The milling process was paused 0.1 h for every 0.4 h to avoid the increase of temperature. The prepared liquid mixture flowed through a homemade filtration device to remove the by-product LiCl. Then the filtered liquid was heated to 205 °C in a homemade vial and vacuumed at the same time for 2 h to eliminate the solvent, and the dry products were obtained. In comparison, Mg(BH4)2 was synthesized from MgCl2 and NaBH4 in dried diethyl ether as described previously.33
Differential scanning calorimetry (DSC) measurements were conducted on a Netzsch STA 449 F3 analyzer under high purity flowing argon conditions (99.999%, 50 mL min−1). The hydrogen desorption/absorption properties were quantitatively evaluated by a volumetric method on a Sieverts-type apparatus, where the experimental data were monitored and recorded automatically. About 150 mg of sample was used for each temperature programmed desorption (TPD) measurement. In the temperature ramp experiments, the temperature was gradually elevated from room temperature to 500 °C at a heating rate of 2 °C min−1 for dehydrogenation (under 4 bar initial hydrogen pressure) and hydrogenation (initially hydrogen pressure being 100 bar). For isothermal examination, the sample was heated to a desired temperature rapidly and kept during the entire measurement.
Morphology and elemental distribution of samples were identified by scanning electron microscopy (SEM, Hitachi SU-70) equipped with an energy dispersive X-ray spectroscopy (EDX, HORIBAX-Max). X-ray diffraction analysis was conducted on an X'Pert Pro X-ray diffractometer (PANalytical, Netherlands) with Cu Kα radiation at 40 kV and 40 mA. A special container fully filled with high purity Ar was prepared to avoid air exposure during sample transferring and testing. Fourier transform infrared (FTIR) spectra were obtained with a Bruker Tensor 27 unit in transmission mode. Solid state magic angle spinning (MAS) NMR spectra were obtained using a Bruker Avance 300 MHz spectrometer with a wide bore 7.04 T magnet and employing a boron-free Bruker 7 mm CPMAS probe. The spectral frequency was 75 MHz for the 11B nucleus and the NMR shifts are reported in parts per million (ppm) externally referenced to NaBH4. The powder materials were packed into 7 mm ZrO2 rotors in an argon-filled glovebox and were sealed with tight fitting Kel-F caps. The one-dimensional (1D) 11B MAS NMR spectra were acquired after a 1.7 μs single π/2 pulse (corresponding to radio field strength of 92.6 kHz). The spectra were recorded at a MAS spinning rate of 5 kHz. The recovery delay was set to 5 s. Spectra were acquired at 20 °C.
Results and discussion
Morphology and structure of as-prepared samples
SEM images of as-prepared samples are presented in Fig. 1. LiBH4 has a flocculent surface in Fig. 1a (×1.00k) and larger magnification picture (Fig. 1b) shows a smooth surface with small holes in it. Comparing Fig. 1c with 1e, the as-synthesized Mg(BH4)2 presents similar morphology with the as-prepared Li–Mg–B–H but with worse electroconductivity. Under enlarged magnification, as-prepared Li–Mg–B–H shows a smooth surface similar to that of LiBH4, while a much rougher surface with small particles on it emerges in the as-synthesized Mg(BH4)2. In addition, as we can see from the EDX mapping data of Li–Mg–B–H in Fig. S1,† the Mg and B elements are dispersed homogeneously in the Li–Mg–B–H matrix, further demonstrating the possibility of the formation of a new compound.
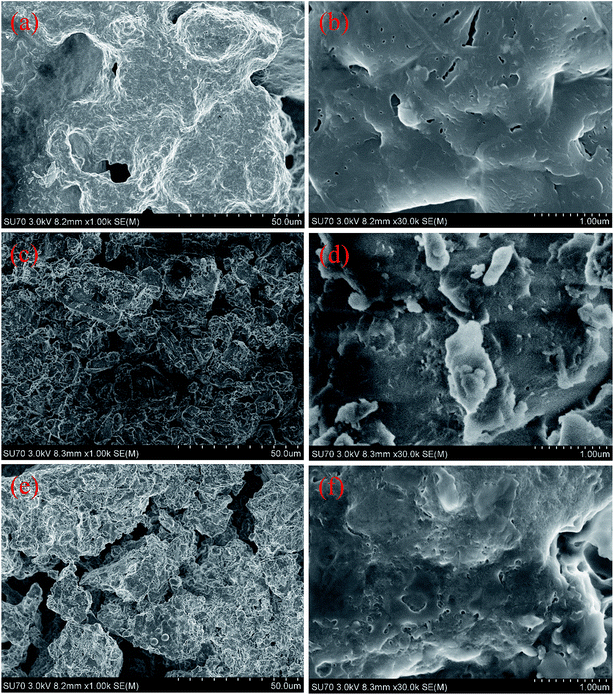 |
| Fig. 1 SEM images of (a, b) LiBH4, (c, d) as-synthesized Mg(BH4)2 and (e, f) as-prepared Li–Mg–B–H. | |
In order to determine the microstructures of as-prepared Li–Mg–B–H, XRD and FTIR examinations of LiBH4, as-synthesized Mg(BH4)2 and as-prepared Li–Mg–B–H were recorded, presented in Fig. 2. The XRD pattern of as-synthesized Mg(BH4)2 in Fig. 2c shows diffraction peaks of both low temperature phase α-Mg(BH4)2 and high temperature phase β-Mg(BH4)2,34 indicating that the as-synthesized Mg(BH4)2 was a mixture of α-Mg(BH4)2 and β-Mg(BH4)2. The physical mixture of LiBH4 and Mg(BH4)2 with a molar ratio of 1
:
1 (Fig. 2e) shows the total diffraction peaks of LiBH4 and Mg(BH4)2. The appearance of LiCl in Fig. 2d and in the filter residue (Fig. S2†) demonstrates the reaction between LiBH4 and MgCl2 occurred during the wet-chemical ball milling process. Combining all the analyses of the diffraction patterns of relevant samples, peaks at 2θ = 16°, 18.1°, 19.1°, 25°, 27.3° in Fig. 2d should be assigned predominately to a new phase, this agrees well with Fang's31 conclusion and these new phases may come from a new dual-cation borohydride.
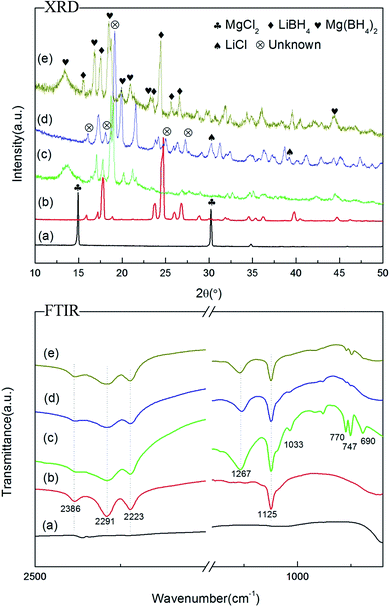 |
| Fig. 2 XRD patterns and FTIR spectra of (a) MgCl2, (b) LiBH4, (c) as-synthesized Mg(BH4)2, (d) as-prepared Li–Mg–B–H, (e) physical mixed LiBH4–Mg(BH4)2. | |
From the results of FTIR measurements, typical features of [BH4]1− group can be readily observed in the spectra, i.e. the stretching and deformation of B–H bonds in the regions between 2200 and 2400 cm−1 and 1100 and 1300 cm−1, respectively.3 The B–H absorption band is split into three contributions at 2386 cm−1, 2291 cm−1, and 2223 cm−1. The B–H bending vibration in LiBH4 is at 1125 cm−1 while that of Mg(BH4)2 is split into two contributions at 1125 cm−1 and 1267 cm−1. The presence of an absorption band around 1033 cm−1 contributes to α-Mg(BH4)2.34 Furthermore, the absorption band in the regions between 650 and 700 cm−1 originate from Mg(BH4)2. The mixed LiBH4 and Mg(BH4)2 shows all the bands of LiBH4 and Mg(BH4)2, as displayed in Fig. 2e. Combining the above analysis, we can see that as-prepared Li–Mg–B–H sample shares the same stretching and deformation of B–H bonds with physically combined LiBH4–Mg(BH4)2 but also has slight difference. It does not show the absorption band in the regions between 650 and 700 cm−1, which originate from Mg(BH4)2.
In order to further clarify the specificity of Li–Mg–B–H, NMR measurements were adopted to test the chemical shift of 11B of LiBH4, as-synthesized Mg(BH4)2, as-prepared Li–Mg–B–H, physical mixed LiBH4–Mg(BH4)2, respectively. If the as-prepared Li–Mg–B–H is a physical mixture, the chemical shift peak of 11B should be a combination of those of LiBH4 and Mg(BH4)2. However, as the NMR results shown in Fig. 3, as-prepared Li–Mg–B–H exhibits different peaks. The peaks of LiBH4, Mg(BH4)2 and physical mixed LiBH4–Mg(BH4)2 appear at −40.30, −39.89, and −40.02 ppm, respectively. The peak of physical mixed sample is asymmetric (a shoulder at around −45 ppm) and the chemical shift is just between −40.30 and-39.89 ppm, which proves the nature of physical mixing. However, the chemical shift of 11B in the primary new Li–Mg–B–H compound is −39.59 ppm, indicating a new chemical circumstance for B. Considering that the starting ingredient are 3LiBH4–MgCl2, the new compound is more likely to be LiMg(BH4)3. Due to the limitation of laboratory equipment, currently we cannot measure out the exact composition of the new compound. Through the above discussion, the as-prepared Li–Mg–B–H is not just a physical mixture of LiBH4 and Mg(BH4)2 but a new dual-cation borohydride.
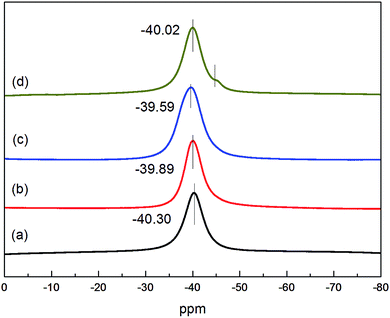 |
| Fig. 3 11B MAS NMR spectra of the (a) LiBH4, (b) as-synthesized Mg(BH4)2, (c) as-prepared Li–Mg–B–H, (d) physical mixed LiBH4–Mg(BH4)2. | |
Hydrogen desorption performance
The DSC-MS characteristics of as-prepared Li–Mg–B–H are shown in Fig. 4. DSC curve exhibits five endothermic peaks, which correspond to the structure transition (104.4 °C), melting (174.2 °C), and decomposition of as-prepared Li–Mg–B–H (260.9, 358.3, 391.6 °C), respectively.12 MS results demonstrate that the gas released is pure H2 without B2H6, and MS desorption peak temperatures are in good agreement with DSC results. Kou et al.35 found that initial hydrogen pressure had a great impact on the dehydrogenation of 2LiBH4–MgH2 system. Under four bar initial hydrogen pressure, LiBH4 reacts with Mg to yield MgB2, which is essential for the reversibility of this system. Four bar initial hydrogen pressure was also adopted to explore its effect on the decomposition of Li–Mg–B–H. Fig. S3† presents the XRD patterns of the decomposed sample of Li–Mg–B–H at different initial hydrogen pressures. We can see from Fig. S3† that when hydrogen pressure raises to 4 bar a tip bumps up at around 42°, which is the main diffraction peak of MgB2. This testifies that four bar initial hydrogen pressure can help to form MgB2 during decomposition. Hence, variable temperature hydrogen desorption behavior of the as-prepared samples was conducted using a TPD apparatus under four bar initial hydrogen pressure.
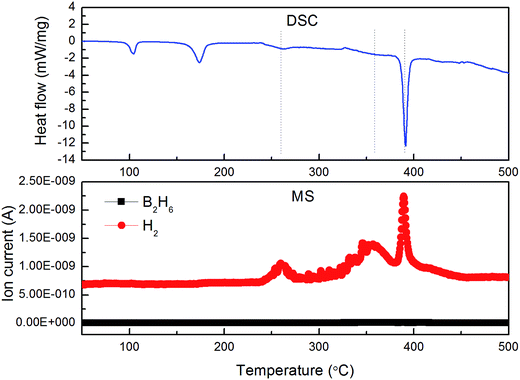 |
| Fig. 4 DSC and MS profiles of Li–Mg–B–H at a heating rate of 5 °C min−1 from room temperature to 500 °C. | |
Fig. 5 shows TPD curves of LiBH4, as-synthesized Mg(BH4)2 and as-prepared Li–Mg–B–H. It was observed that the decomposition of the pristine LiBH4 started sluggishly at 320 °C, resulting in a final release of 4.3 wt% hydrogen at 500 °C. As-synthesized Mg(BH4)2 first decomposed at 300 °C, quickly releasing a large amount of H2 with the elevating temperature and finally reached a 11.7 wt% hydrogen release at 500 °C. Worth-noting, the operating temperature for hydrogen desorption of as-prepared Li–Mg–B–H was significantly reduced to 250 °C, 70 °C and 50 °C lower compared to that of pristine LiBH4 and as-synthesized Mg(BH4)2, respectively. In total, 12.3 wt% hydrogen was released from the dual-cation borohydride, which is three times larger comparing to that of pristine LiBH4 (4.3 wt%) and higher than that of as-synthesized Mg(BH4)2 (11.7 wt%). Furthermore, the new compound can release hydrogen at a rate of 13.80 wt% per h at 375 °C, just as fast as as-synthesized Mg(BH4)2, 30 times faster than pristine LiBH4 (0.45 wt% per h). The findings indicate that the idea of using higher χp of metal ions Mg2+ to partially substitute the Li cations to form a dual-cation borohydride Li–Mg–B–H truly improves both the dehydrogenation thermodynamics and kinetics of LiBH4.
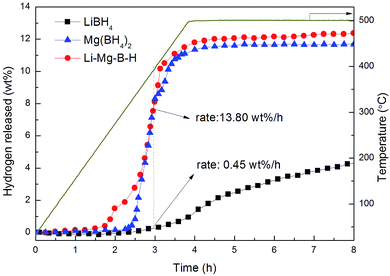 |
| Fig. 5 TPD profiles of LiBH4, Mg(BH4)2 and Li–Mg–B–H at a heating rate of 2 °C min−1 from room temperature to 500 °C under 4 bar initial hydrogen pressure. | |
Dehydrogenation reaction mechanism
In order to fully understand the dehydrogenation process, the as-prepared Li–Mg–B–H was heated to different temperatures (295, 395, and 495 °C) according to the DSC-MS and TPD results and the decomposed products were collected and applied with XRD and FTIR measurements, displayed in Fig. 6. In the XRD patterns, Fig. 6b shows that high-temperature phase of LiBH4, MgH2 and MgO (MgO comes from the air contamination of the sample during operation) formed after heated at 295 °C. From the FTIR profile in Fig. 6b, we can see that the peaks of the B–H bonding at 2291, 2223 and 1125 cm−1, which confirm the presence of LiBH4.6 When heated up to 395 °C, metallic Mg and MgO are the only phases detected by XRD, whereas the diffraction peaks of MgH2 are completely absent. The FTIR pattern shows that LiBH4 still exists. So in this step, MgH2 decomposes to Mg and H2. As the dehydrogenation temperature further increases to 495 °C, the final products are Mg, MgO, MgB2 and LiH. And the peaks of B–H bonds disappear in FTIR, indicating the complete decomposition of LiBH4. To sum up, the main decomposition pathway of the new compound under four bar initial hydrogen pressure can be described as follows:
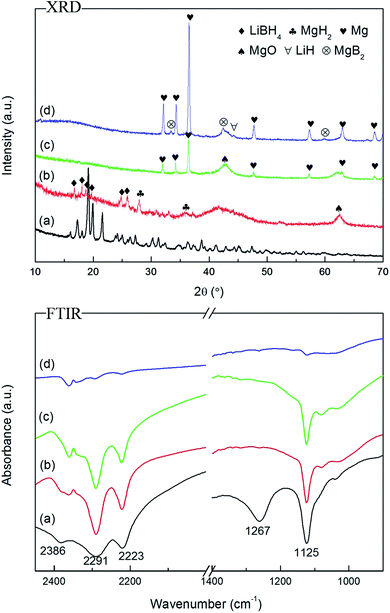 |
| Fig. 6 XRD patterns and FTIR spectra of Li–Mg–B–H decomposed at different temperatures: (a) room temperature, (b) 295 °C, (c) 395 °C, and (d) 495 °C. | |
(Step 1)
Li–Mg–B–H → xLiBH4 + MgH2 + B + H2 (0 < x ≤ 1) |
(Step 2)
(Step 3)
xLiBH4 + Mg → 0.5xMgB2 + xLiH + 1.5xH2 + (1 − 0.5x)Mg |
Hydrogen storage reversibility
Reversibility is one of the key features for hydrogen storage materials, especially for on-board applications. In order to study the reversibility of the new compound, we use temperature programmed absorption as well as isothermal rehydrogenation. Fig. 7a shows the temperature absorption curve from room temperature to 500 °C with a heating rate of 2 °C min−1. The pressure is increasing linearly before the temperature is heated to 420 °C, while maintaining and even decreasing when the temperature is above 420 °C. This indicates that the dehydrogenated product of Li–Mg–B–H could be rehydrogenated at about 420 °C. From the isothermal rehydrogenation curve at 420 °C in Fig. 7b, the reduction in hydrogen pressure is related to the amount of absorbed hydrogen. XRD patterns (see in Fig. S4†) show that the rehydrogenated sample is composed of MgH2 and LiBH4. The dehydrogenation performance of the rehydrogenated sample was further carried out. The dehydrogenation curve is shown in Fig. 8, and it can be observed that the rehydrogenated products can release 5.3 wt% hydrogen, indicating that the new compound has certain reversibility. Nevertheless, this is a great improvement for the reversibility of Li–Mg–B–H comparing with a previous work,31 which can only be rehydrogenated to MgH2 and release about 2 wt% hydrogen. Owing to the complexity of Li–Mg–B–H, detailed experimental and theoretical studies are still required to better understand the cyclic de/rehydrogenation behaviors of the Li–Mg–B–H system.
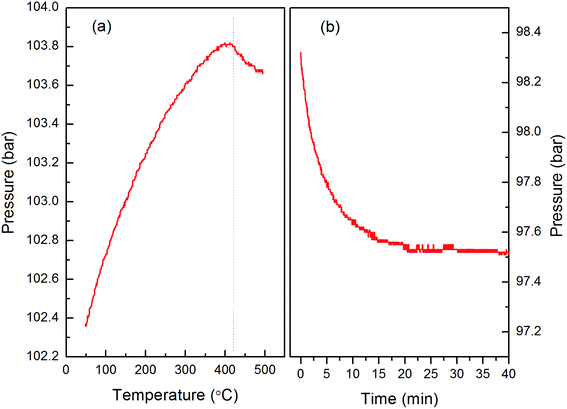 |
| Fig. 7 The rehydrogenation curves of the dehydrogenated sample of Li–Mg–B–H: (a) temperature programmed absorption from room temperature to 500 °C at a heating rate of 2 °C min−1 and (b) isothermal rehydrogenation at 420 °C. | |
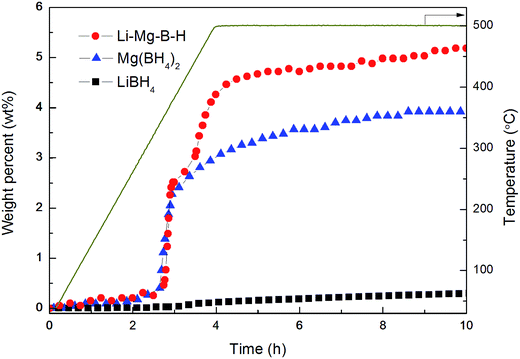 |
| Fig. 8 Hydrogen desorption curve at a heating rate of 2 °C min−1 of the rehydrogenated sample. | |
Conclusion
In summary, a new dual-cation borohydride Li–Mg–B–H has been successfully synthesized by ball-milling 3LiBH4 + MgCl2 mixture in Et2O. Further investigations show that dehydrogenation performance of the new Li–Mg–B–H was affected by initial hydrogen pressure. Under four bar initial hydrogen pressure, the onset dehydrogenation temperature of Li–Mg–B–H (250 °C) is 70 °C and 50 °C lower compared to pristine LiBH4 and Mg(BH4)2, respectively. The Li–Mg–B–H can release 12.3 wt% hydrogen from room temperature to 500 °C according to the following three steps:
(Step 1)
Li–Mg–B–H → xLiBH4 + MgH2 + B + H2 (0 < x ≤ 1) |
(Step 2)
(Step 3)
xLiBH4 + Mg → 0.5xMgB2 + xLiH + 1.5xH2 + (1 − 0.5x)Mg |
In addition, partial reversibility of Li–Mg–B–H has been demonstrated and 5.3 wt% hydrogen can be released in the second cycle. According to the experimental results of this work, the strategy of altering the χp of metal ions in LiBH4 can truly improve the hydrogen storage properties of LiBH4 and it may provide general guidance and inspiration in dual-cation borohydrides hydrogen storage materials with advanced and controllable performances.
Acknowledgements
The authors gratefully acknowledge the financial supports for this research from the National Natural Science Foundation of China (51671173, 51571179 and 51471151), the Program for Innovative Research Team in University of Ministry of Education of China (IRT13037) and the Scientific Research Starting Foundation of Jiangsu University of Science and Technology (1142931607).
References
- M. Felderhoff, C. Weidenthaler, R. von Helmolt and U. Eberle, Hydrogen storage: the remaining scientific and technological challenges, Phys. Chem. Chem. Phys., 2007, 9, 2643–2653 RSC.
- L. Zhang, L. Chen, X. Fan, X. Xiao, J. Zheng and X. Huang, Enhanced hydrogen storage properties of MgH2 with numerous hydrogen diffusion channels provided by Na2Ti3O7 nanotubes, J. Mater. Chem. A, 2017, 5, 6178–6185 CAS.
- L. Schlapbach and A. Züttel, Hydrogen-storage materials for mobile applications, Nature, 2001, 414, 353–358 CrossRef CAS PubMed.
- A. Züttel, S. Rentsch, P. Fischer, P. Wenger, P. Sudan, P. h. Mauron and C. h. Emmenegger, Hydrogen storage properties of LiBH4, J. Alloys Compd., 2003, 356, 515–520 CrossRef.
- C. Luo, H. Wang, T. Sun and M. Zhu, Enhanced dehydrogenation properties of LiBH4 compositing with hydrogenated magnesium-rare earth compounds, Int. J. Hydrogen Energy, 2012, 37, 13446–13455 CrossRef CAS.
- Y. Zhao, Y. Liu, H. Liu, H. Kang, K. Cao, Q. Wang, C. Zhang, Y. Wang, H. Yuan and L. Jiao, Improved dehydrogenation performance of LiBH4 by 3D hierarchical flower-like MoS2 spheres additives, J. Power Sources, 2015, 300, 358–364 CrossRef CAS.
- H. Liu, L. Jiao, Y. Zhao, K. Cao, Y. Liu, Y. Wang and H. Yuan, Improved dehydrogenation performance of LiBH4 by confinement into porous TiO2 micro-tubes, J. Mater. Chem. A, 2014, 2, 9244–9250 CAS.
- O. Friedrichs, J. W. Kim, A. Remhof, F. Buchter, A. Borgschulte, D. Wallachr, Y. W. Cho, M. Fichtner, K. H. Oh and A. Züttel, The effect of Al on the hydrogen sorption mechanism of LiBH4, Phys. Chem. Chem. Phys., 2009, 11, 1515–1520 RSC.
- G. Xia, Y. Guo, Z. Wu and X. Yu, Enhanced hydrogen storage performance of LiBH4–Ni composite, J. Alloys Compd., 2009, 479, 545–548 CrossRef CAS.
- Y. Guo, X. Yu, L. Gao, G. Xia, Z. Guo and H. Liu, Significantly improved dehydrogenation of LiBH4 destabilized by TiF3, Energy Environ. Sci., 2010, 3, 465–470 CAS.
- W. Cai, H. Wang, D. Sun and M. Zhu, Nanosize-controlled reversibility for a destabilizing reaction in the LiBH4–NaH2+x system, J. Phys. Chem. C, 2013, 117, 9566–9572 CAS.
- P. Mauron, M. Bielmann, A. Remhof, A. Züttel, J. H. Shim and Y. W. Cho, Stability of the LiBH4/CeH2 composite system determined by dynamic PCT measurements, J. Phys. Chem. C, 2010, 114, 16801–16805 CAS.
- F. C. Gennari, L. F. Albanesi, J. A. Puszkiel and P. A. Larochette, Reversible hydrogen storage from 6LiBH4–MCl3 (M = Ce, Gd) composites by in situ formation of MH2, Int. J. Hydrogen Energy, 2011, 36, 563–570 CrossRef CAS.
- F. E. Pinkerton and M. S. Meyer, Reversible hydrogen storage in the lithium borohydride-calcium hydride coupled system, J. Alloys Compd., 2008, 464, L1–L4 CrossRef CAS.
- J. J. Vajo, W. Li and P. Liu, Thermodynamic and kinetic destabilization in LiBH4/Mg2NiH4: promise for borohydride based hydrogen storage, Chem. Commun., 2010, 46, 6687–6689 RSC.
- D. Liu, Q. Liu, T. Si, Q. Zhang, F. Fang, D. Sun, L. Ouyang and M. Zhu, Superior hydrogen storage properties of LiBH4 catalyzed by Mg(AlH4)2, Chem. Commun., 2011, 47, 5741–5743 RSC.
- A. Surrey, C. B. Minella, N. Fechler, M. Antonietti, H. J. Grafe and L. Schultz, Improved hydrogen storage properties of LiBH4 via nanoconfinement in micro- and mesoporous aerogel-like carbon, Int. J. Hydrogen Energy, 2016, 41, 5540–5548 CrossRef CAS.
- P. Negen, P. Adelhelm, A. M. Beale, K. P. de Jong and P. E. de Jong, LiBH4/SBA-15 nanocomposites prepared by melt infiltration under hydrogen pressure: synthesis and hydrogen sorption properties, J. Phys. Chem. C, 2010, 114, 6163–6168 Search PubMed.
- P. Javadian, D. A. Sheppard, C. E. Buckley and T. R. Jensen, Hydrogen storage properties of nanoconfined LiBH4–Ca(BH4)2, Nano Energy, 2015, 11, 96–103 CrossRef CAS.
- P. Choudhury, S. S. Srinivasan, V. R. Bhethanabotla, Y. Goswami, K. McGrath and E. K. Stefanakos, Nano-Ni doped Li–Mn–B–H system as a new hydrogen storage candidate, Int. J. Hydrogen Energy, 2009, 34, 6325–6334 CrossRef CAS.
- B. Zhai, X. Xiao, W. Lin, X. Huang, X. Fan, S. Li, H. Ge, Q. Wang and L. Chen, Enhanced hydrogen desorption properties of LiBH4–Ca(BH4)2 by synergetic effect of nanoconfinement and catalysis, Int. J. Hydrogen Energy, 2016, 41, 17462–17470 CrossRef CAS.
- J. Y. Lee, D. Ravnsbæk, Y. S. Lee, Y. Kim, Y. Cerenius, J. H. Shim, T. R. Jensen, N. H. Hur and Y. W. Cho, Decomposition reactions and reversibility of the LiBH4–Ca(BH4)2 composite, J. Phys. Chem. C, 2009, 113, 15080–15086 CAS.
- Y. Zhang, Q. Tan, H. Chu, J. Zhang, L. Sun and Z. Wen, Hydrogen de/resorption properties of the LiBH4–MgH2–Al system, J. Phys. Chem. C, 2009, 113, 21964–21969 CAS.
- P. Ngene, M. van Zwienen and P. E. de Jongh, Reversibility of the hydrogen desorption from LiBH4: a synergetic effect of nanoconfinement and Ni addition, Chem. Commun., 2010, 46, 8201–8203 RSC.
- Z. Zhao-Karger, R. Witter, E. G. Bardaji, D. Wang, D. Cossement and M. Fichtner, Altered reaction pathways of eutectic LiBH4–Mg(BH4)2 by nanoconfinement, J. Mater. Chem. A, 2013, 1, 3379–3386 CAS.
- P. Choudhury, S. S. Srinivasan, V. R. Bhethanabotla, Y. Goswami, K. McGrath and E. K. Stefanakos, Nano-Ni doped Li–Mn–B–H system as a new hydrogen storage candidate, Int. J. Hydrogen Energy, 2009, 34, 6325–6334 CrossRef CAS.
- H. W. Li, S. Orimo, Y. Nakamori, K. Miwa, N. Ohba, S. Towata and A. Züttel, Materials designing of metal borohydrides: Viewpoints from thermodynamical stabilities, J. Alloys Compd., 2007, 446, 315–318 CrossRef.
- Y. Nakamori, K. Miwa, A. Ninomiya, H. W. Li, N. Ohba, S. Towata, A. Züttel and S. Orimo, Thermodynamical stabilities of metal-borohydrides, Phys. Rev. B, 2006, 74, 045126 CrossRef.
- K. Jiang, X. Xiao, S. Deng, M. Zhang, S. Li, H. Ge and L. Chen, A Novel Li–Ca–B–H Complex Borohydride: Its Synthesis and Hydrogen Storage Properties, J. Phys. Chem. C, 2011, 115, 19986–19993 CAS.
- C. Kim, S. Hwang, R. C. Bowman Jr, J. W. Reiter, J. A. Zan, J. G. Kulleck, H. Kabbour, E. H. Majzoub and V. Ozolins, LiSc(BH4)4 as a hydrogen storage material: multinuclear high-Resolution solid-state NMR and first-Principles Density functional Theory Studies, J. Phys. Chem. C, 2009, 113, 9956–9968 CAS.
- Z. Fang, X. Kang, P. Wang, H. Li and S. I. Orimo, Unexpected dehydrogenation behavior of LiBH4/Mg(BH4)2 mixture associated with the in situ formation of dual-cation borohydride, J. Alloys Compd., 2010, 491, L1–L4 CrossRef CAS.
- E. G. Bardají, Z. Zhao-Karger, N. Boucharat, A. Nale, M. J. van Setten, W. Lohstroh, E. Röhm, M. Catti and M. Fichtner, LiBH4–Mg(BH4)2: a physical mixture of metal borohydrides as hydrogen storage material, J. Phys. Chem. C, 2011, 115, 6095–6101 Search PubMed.
- G. L. Soloveichik, M. Andrus, Y. Gao, J. Zhao and S. Kniajanski, Magnesium borohydride as a hydrogen storage material: synthesis of unsolvated Mg(BH4)2, Int. J. Hydrogen Energy, 2009, 34, 2144–2152 CrossRef CAS.
- K. Chłopek, C. Frommen, A. Léon, O. Zabara and M. Fichtner, Synthesis and properties of magnesium tetrahydroborate, Mg(BH4)2, J. Mater. Chem., 2007, 17, 3496–3503 RSC.
- H. Kou, X. Xiao, L. Chen, S. Li and Q. Wang, Formation mechanism of MgB2 in 2LiBH4 + MgH2 system for reversible hydrogen storage, Trans. Nonferrous Met. Soc. China, 2011, 21, 1040–1046 CrossRef CAS.
Footnotes |
† Electronic supplementary information (ESI) available. See DOI: 10.1039/c7ra06599j |
‡ Liuting Zhang and Jiaguang Zheng contributed equally. |
|
This journal is © The Royal Society of Chemistry 2017 |
Click here to see how this site uses Cookies. View our privacy policy here.