DOI:
10.1039/C7RA06327J
(Paper)
RSC Adv., 2017,
7, 37261-37267
Cobalt(II)-8-hydroxyquinoline-5-sulfonic acid complex/N-(4-aminobutyl)-N-ethylisoluminol/reduced graphene hybrids as nanocatalytic reaction platforms for chemiluminescence†
Received
6th June 2017
, Accepted 23rd July 2017
First published on 27th July 2017
Abstract
In this work, we report a novel GO-based hybrid consisting of N-(4-aminobutyl)-N-ethylisoluminol (ABEI), a cobalt(II)-8-hydroxyquinoline-5-sulfonic acid complex (CoII(HQS)2) and reduced GO hybrids (CoII(HQS)2/ABEI/rGO) with good stability and easy purification via a facile strategy by virtue of π–π stacking and coordination, which could be used as an excellent nanocatalytic reaction platform for chemiluminescence (CL) when reacted with hydrogen peroxide, dissolved oxygen and periodate in alkaline solution. This was attributed to the fact that CoII(HQS)2 catalyzed the generation of reactive radicals such as HO˙, O2˙−, and periodate anion radicals (IVI) and π-conjugated carbon radicals (π–C
C˙) as well as ABEI˙− on the GO surface. GO as a reaction platform facilitated the reaction of ABEI˙− with O2˙− to produce intensive CL. It was also found that there was a dilution-initiated CL enhancement in CoII(HQS)2/ABEI/rGO–H2O2 system but not in the CoII(HQS)2/ABEI/rGO–O2 and CoII(HQS)2/ABEI/rGO–KIO4 systems. This was due to the competition of the dilution-decreased reduced GO quenching effect and dilution-decreased CoII(HQS)2 concentration. This work provides new insight into the physicochemical properties of functionalized GO hybrids and excellent nanocatalytic platforms for CL reactions, which may find future applications in bioassays, biosensors, bioimaging and microchips.
Introduction
Nanomaterials as catalysts in chemiluminescence (CL) reactions have enjoyed great attention owing to their important potential applications in sensors, catalysts, bioassays and biological imaging.1–4 Since Zhang and coworkers first reported the catalysis of gold nanoparticles (AuNPs) in luminol CL reactions,5 studies on nanomaterial-catalyzed CL reactions have been extended to various nanomaterials, such as AgNPs,6 PtNPs,7 ZnO NPs,8 etc. Such nanomaterials as nanosized platforms could effectively facilitate the formation of reactive radicals and electron transfer, leading to strong light emission.5 In the above work, CL reagents existed in liquid-phase in the CL reactions. Subsequently, it was found that CL efficiency could be improved when CL reagents were directly immobilized on the surface of nanomaterials due to the enhanced electron transfer ability on the surface of the nanointerface.9 These CL functionalized nanomaterials have been successfully used as nanoprobes and nanointerfaces for label-based and label-free bioassays.10–12 Furthermore, in order to further improve CL efficiency, both of CL reagent and catalyst (include metal ions, metal complexes and molecules) were immobilized on the surface of nanomaterials. Unique CL emission was observed on nanocatalytic reaction platform due to synergistic effect of nanomaterials, catalysts and CL reagents occurred on such heterogeneous nanointerface. For examples, CL reagent N-(4-aminobutyl)-N-ethylisoluminol (ABEI) and catalyst cobalt complexes were successfully grafted on the surface of gold nanoparticles, which exhibited excellent CL and electrochemiluminescence (ECL) activity and was used for the determination of specific DNA sequence related to diseases.13,14 Graphene and graphene oxide (GO) with a single atomic layer of sp2 carbon atoms and high surface-to-volume ratio could be also used as nanocatalytic reaction platforms for CL reactions.15,16 Later on, ABEI and catalyst bifunctionalized GO or graphene hybrids with higher CL efficiency, including ABEI/hemin bifunctionalized GO and ABEI/horseradish peroxidase bifunctionalized GO, have been developed.17,18 However, nanocatalytic reaction platforms were restricted to the reactions of luminol-type CL reagents with H2O2 and nanocatalytic reaction platforms for various CL reactions are far from fully developed.
In this work, we report a novel GO-based hybrid with good stability and easy purification consisting of ABEI, CoII(HQS)2 and reduced GO hybrids (CoII(HQS)2/ABEI/rGO) via a facile strategy. The as-prepared CoII(HQS)2/ABEI/rGO hybrids were characterized by atomic force microscopy (AFM), UV-vis absorption spectra, fluorescence spectra (FL), Raman spectra, X-ray photoelectron spectroscopy (XPS) and atomic emission spectrophotometry (ICP-AES). And the assembly mechanism was discussed. It was found that the as-prepared CoII(HQS)2/ABEI/rGO could be used as excellent nanocatalytic reaction platforms for CL when reacted with hydrogen peroxide, the dissolved oxygen and periodate in alkaline solution. The mechanism of various CL reactions was explored. Moreover, the effect of dilution on CL behavior of various CL reactions was studied and related mechanism was discussed.
Experimental section
Chemicals and materials
Graphite oxide was purchased from XFNANO Materials Tech Co., Ltd. (Nanjing, China). N-(4-Aminobutyl)-N-ethylisoluminol (ABEI) (TCI, Japan) without further purification was dissolved in 0.1 M NaOH solution to prepare a 10 mM stock solution of ABEI. 8-Hydroxyquinoline-5-sulphonic (HQS) was purchased from J&K Scientific Ltd. (Shanghai, China). Cobalt(II) chloride hexahydrate, ammonia, hydrazine, and potassium periodate (KIO4) were purchased form Sinopharm Chemical Reagent Co. Ltd (Shanghai, China). Working solutions of H2O2 were prepared fresh daily from 30% (v/v) H2O2 (Xinke Electrochemical Reagent Factory, Bengbu, China). All other reagents were of analytical grade. Ultrapure water was prepared by a Milli-Q system (Millipore, France) and was used throughout.
Preparation of CoII(HQS)2/ABEI/rGO
Firstly, 0.1 mg mL−1 GO aqueous dispersion was obtained from graphite oxide by ultrasonic for 2 hours. Then 1 mL of 10 mM ABEI in NaOH solution was added to 100 mL of 0.1 mg mL−1 GO aqueous dispersion. After sonication for 10 min, the mixture was vigorously stirred at 80 °C for 24 h to obtain the ABEI/GO. For the preparation of CoII(HQS)2 complex, 23.8 mg CoCl2·6H2O was added into 100 mL of 2 mM HQS alkaline solution containing 0.005 M NaOH. The above solutions were mixed, stirred and heated for about an hour at 60 °C to form a yellow and transparent solution. Next, 1 mL of CoII(HQS)2 complex solution and 200 μL of ammonia solution were added into 100 mL of the as-prepared ABEI/GO solution, followed by the addition of 30 μL of hydrazine solution. Then the mixture was vigorously stirred at 60 °C for 4 h. After twice centrifugation and redispersion, CoII(HQS)2/ABEI/rGO hybrids were obtained.
Characterization of CoII(HQS)2/ABEI/rGO hybrids
The morphology and surface composition of as-prepared CoII(HQS)2/ABEI/rGO hybrids were characterized by UV-visible spectra (Agilent 8453 UV-visible spectrophotometer, USA), Raman spectra (LabRamHR, JobinYvon, France), AFM (Multimode V, Veeco), XPS (VG Scientific, UK) with Al Kα radiation as the X-ray source and ICP-AES (Optima 7300 DV, PerkinElmer, US).
CL measurements
The CL property of CoII(HQS)2/ABEI/rGO hybrids was studied by static injection CL system with a centro LB 960 microplate luminometer (Berthold, Germany). For the CL reactions of CoII(HQS)2/ABEI/rGO hybrids with oxidant H2O2, O2 and KIO4, 50 μL of purified CoII(HQS)2/ABEI/rGO was first added into the wells of 96-well plates and then 50 μL of oxidant was injected into the wells, CL signals were produced and recorded. CL spectra were measured on a FL spectrometer (HITACHI F-7000, Hitachi, Japan) operated with the lamp off.
Results and discussion
Preparation and characterization of CoII(HQS)2/ABEI/rGO
The preparation of CoII(HQS)2/ABEI/rGO hybrids is depicted in Scheme 1. First, ABEI molecules were mixed with GO at 80 °C for 24 hours to produce ABEI/GO hybrids. Second, CoII(HQS)2 complex reacted with ABEI/GO hybrids in the presence of ammonia and hydrazine to obtain CoII(HQS)2/ABEI/rGO hybrids. The as-prepared CoII(HQS)2/ABEI/rGO hybrids easy separated from precursor exhibited good stability and water-solubility.
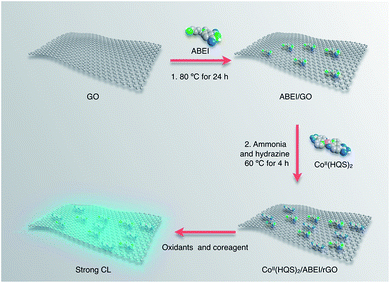 |
| Scheme 1 Illustration of preparation of CoII(HQS)2/ABEI/rGO hybrids. | |
The morphology of the as-prepared CoII(HQS)2/ABEI/rGO hybrids was investigated by AFM. The AFM images with a height profile are shown in Fig. 1. The morphology of CoII(HQS)2/ABEI/rGO is the same with that of GO and ABEI/GO, which is cast on a mica wafer with essentially single-layered carbon structure. These results indicate that the as-prepared CoII(HQS)2/ABEI/rGO hybrids remain dispersive. The thickness of GO measured from the height profile of the AFM image is about 0.76 nm, which is consistent with the data reported in the literature.19 After functionalized with ABEI, the height of ABEI/GO sheet shows a 0.14 nm increment. The thickness of CoII(HQS)2/ABEI/rGO is about 1.15 nm, indicating that ABEI molecule and CoII(HQS)2 complex are assembled on the surface of graphene sheet.
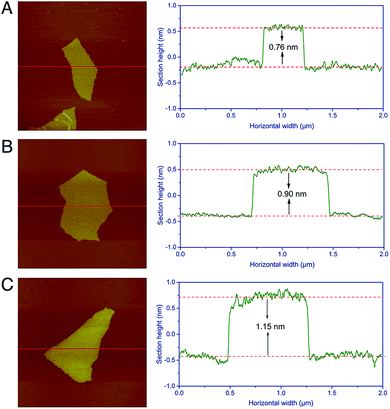 |
| Fig. 1 Tapping mode AFM images with height profile (green curves) taken along the red line of (A) exfoliated GO, (B) ABEI/GO and (C) CoII(HQS)2/ABEI/rGO. | |
The composition of the as-prepared CoII(HQS)2/ABEI/rGO was studied by XPS, ICP-AES, UV-vis and Raman spectra. XPS of CoII(HQS)2/ABEI/rGO is shown in Fig. 2, C 1s/O 1s ratio obtained from the XPS survey spectrum increased after ABEI and CoII(HQS)2 were connected to the surface of GO successively. Compared with GO, the C 1s spectrum of CoII(HQS)2/ABEI/rGO showed a great decrease at 286.4 eV and 287.2 eV. The results strongly supported that the GO in CoII(HQS)2/ABEI/rGO was partially reduced. The N 1s spectrum of CoII(HQS)2/ABEI/rGO was curved-fitted into three components at 399.5, 400.7 and 401.8 eV, which were contributed to the –N–sp3C, –N–sp2C and quaternary N, respectively.20 Compared with the N 1s spectrum of GO, the survey of CoII(HQS)2/ABEI/rGO and ABEI/GO showed a new peak at 400.7 eV originating from the –N–CO– group in ABEI. And the N 1s peak at 399.5 eV increased due to the –N–sp3C from ABEI. Hence, the results indicated that the ABEI existed on the surface of ABEI/GO and CoII(HQS)2/ABEI/rGO. The XPS spectra of CoII(HQS)2/ABEI/rGO demonstrated the existence of S 2p and Co 2p binding energy, which were absent in the survey spectrum of ABEI/GO (Fig. S1†). The Co 2p spectrum was curved-fitted into four components at 796.7, 781.0, 801.2 and 785.8 eV, which was attributed to the 2p1/2, 2p3/2 spin–orbit and the satellite lines for the high spin Co(II), respectively.21 The results indicated that CoII(HQS)2 complex existed on the surface of GO sheets and cobalt was mainly Co(II). Furthermore, the amount of Co(II) was calculated as 0.33 μg mL−1 by ICP-AES. As shown in Fig. S2,† UV-vis spectra also supported the presence of ABEI molecules and CoII(HQS)2 complex and on the surface of CoII(HQS)2/ABEI/rGO. Raman spectra were also used to monitor the structural changes during the synthesis of CoII(HQS)2/ABEI/rGO from GO. It was reported that the intensity of the D Raman band was a marker of the defect density and the I(D)/I(G) ratio was an important parameter to characterize graphene defect density.22 As shown in Fig. S3,† the I(D)/I(G) ratio was in the order: CoII(HQS)2/ABEI/rGO > ABEI/GO > GO. The results also indicated that GO were deoxygenated in CoII(HQS)2/ABEI/rGO, which was in good agreement with the XPS results.
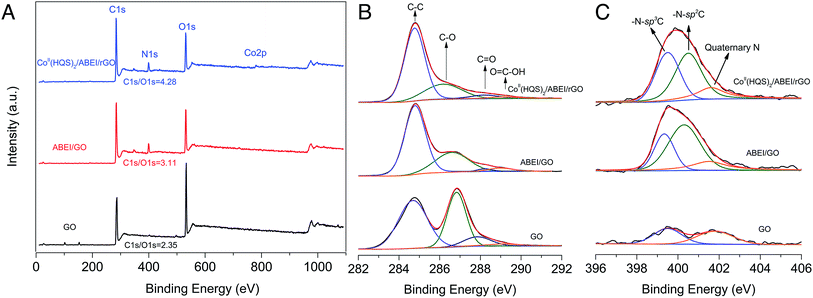 |
| Fig. 2 Survey XPS data of (A) GO, ABEI/GO and CoII(HQS)2/ABEI/rGO. XPS survey of (B) C 1s and (C) N 1s from GO, ABEI/GO and CoII(HQS)2/ABEI/rGO, respectively. | |
The assembly mechanism of CoII(HQS)2/ABEI/rGO was investigated. Our previous work suggested that ABEI was attached to graphene via π–π interaction.23 HQS was used as a ligand with great complexation constants and water solubility, which could complex with metal ion through N atoms and deprotonated quinolinol-O atoms.24,25 In this case, cobalt(II) ion could also complex with HQS to form a 1
:
2 complex with an octahedral coordination structure. In the equatorial positions, Co2+ are coordinated by the N and O atoms of the quinolinol moiety to form a planar structure. Due to the π-conjugated quinolinol group, CoII(HQS)2 might be assembled to the surface of GO via π–π stacking. On the other hand, O atom from GO might coordinate with Co2+ via the axial position.26 Accordingly, CoII(HQS)2 complex might be absorbed on the surface of GO sheets via π–π stacking and coordination between CoII(HQS)2 and GO as shown in Fig. S4.†
CL behavior in hydrogen peroxide system
The CL behavior of CoII(HQS)2/ABEI/rGO hybrids with H2O2 in alkaline solution was investigated. As shown in Fig. 3, it can be seen that CoII(HQS)2/ABEI/rGO (black line) exhibited excellent CL activity, which was ∼70 times higher than that of ABEI/GO (green line) and the CL kinetic curve showed a typical flash light emission. In addition, the synthesis dosage of ABEI in the hybrids is about 1/18 of that used in previously reported ABEI functionalized rGO hybrids.17,23 The results revealed that the incorporation of CoII(HQS)2 into ABEI/GO to form a novel bifunctionalized GO hybrids could highly improve the CL activity. As shown in Fig. S5,† the maximum CL emission wavelength of CoII(HQS)2/ABEI/rGO–H2O2 was centered at ∼440 nm, which was consistent with that of ABEI with H2O2. Thus, the CL emission was from the reaction of ABEI on the surface of CoII(HQS)2/ABEI/rGO with H2O2. As shown in Fig. S6,† the effects of pH and concentration of H2O2 on the CL reaction between CoII(HQS)2/ABEI/rGO and H2O2 were investigated. The CL intensity increased with the increase of pH. And the logarithm of integrated of CL intensity increased linearly with the logarithm of the concentration of H2O2 in the range of 0.1 μM to 0.5 mM. The results indicated that higher alkaline condition and H2O2 concentration are advantageous to the CL reaction in CoII(HQS)2/ABEI/rGO–H2O2 system, which was consistent with the CL mechanism of cyclic hydrazides.27,28 The decomposition of H2O2 would generate hydroxyl radicals (HO˙) and superoxide radicals (O2˙−), which could react with ABEI to produce CL. The higher pH could accelerate the decomposition of H2O2 and the deprotonation of the excited state oxidation product of ABEI (ABEI-OX*) and generate higher CL emission. Finally, 0.1 mM H2O2 (pH 12) was recommended as optimized conditions for the CL because higher solution pH would corrode the injector of luminometer and higher concentration of H2O2 would cause poor stability.
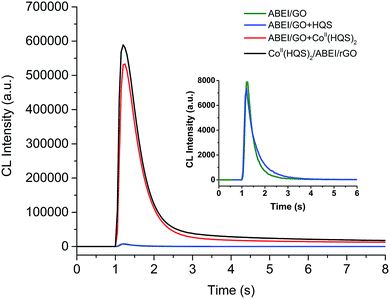 |
| Fig. 3 CL kinetic curves for reaction of ABEI/GO, ABEI/GO mixed with HQS, ABEI/GO mixed with CoII(HQS)2 and CoII(HQS)2/ABEI/rGO with H2O2. Reaction conditions: 0.1 mM H2O2 in 0.01 M NaOH. | |
Furthermore, the CL enhancement of CoII(HQS)2 and GO in ABEI–H2O2 system was investigated. The addition of HQS only affect slightly the CL kinetic curve. When CoII(HQS)2 aqueous solution was added into ABEI/GO dispersion, strong CL emission was observed as shown in Fig. 3 (red line). The result indicated that CoII(HQS)2 as an efficient catalyst could increase the rate of CL reaction and thereby greatly increased the CL intensity. Earlier studies showed that Co2+ could interact with H2O2 to rapidly generate HO˙ and O2˙−.29,30 N. V. Thakkar and coworkers reported that the complexation of Co2+ have enhanced effect on the catalytic of decomposition of H2O2.31,32 CoII(HQS)2 complex might have similar enhanced catalytic effect on the decomposition of H2O2 to facilitate the formation of HO˙ and O2˙−. Moreover, the CL intensity obviously increased in an oxygen-saturated solution and decreased in a nitrogen-saturated solution, indicating that the dissolved oxygen (O2) took part in the CL reaction (Fig. S7A†).
In view of the above discussion, the CL mechanism of CoII(HQS)2/ABEI/rGO with H2O2 is summarized in Scheme 2. The immobilized CoII(HQS)2 complex could catalyze the decomposition of H2O2 to yield highly reactive HO˙ and O2˙− radicals on the surface of GO, which reacted with GO to produce π–C
C˙. Such radicals reacted with ABEI to form ABEI radicals (ABEI˙−), which further interacted with O2˙− to generate intensive CL emission. The result demonstrated that the CoII(HQS)2 complex and the GO platform exhibited superior catalysis effect in CoII(HQS)2/ABEI/rGO–H2O2 system. The as-prepared CoII(HQS)2/ABEI/rGO could act as an effective nanocatalytic reaction platform for the heterogenous CL reaction of ABEI with H2O2.
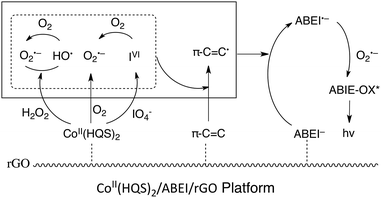 |
| Scheme 2 The CL mechanism of CoII(HQS)2/ABEI/rGO with H2O2, dissolved O2 and IO4−. | |
CL behavior in the dissolved oxygen system
It was found that as-prepared CoII(HQS)2/ABEI/rGO could directly react with 0.1 M NaOH solution to produce strong CL emission as shown in Fig. 4 (black line). Controlling experiments were also carried out when 0.1 M NaOH was added to control solutions (Fig. 4). Very weak light emission was observed when ABEI/GO (green line) or the mixture of ABEI/GO with HQS (blue line) was added to 0.1 M NaOH solution. The CL intensity from the mixture of CoII(HQS)2 with ABEI/GO enhanced by 50 times comparing with that of ABEI/GO. The CL intensity of CoII(HQS)2/ABEI/rGO was about 500 times higher than that of ABEI/GO. The immobilization of CoII(HQS)2 complexes on the surface of ABEI/GO resulted in further increase of the CL intensity. Moreover, the maximum emission wavelength of CoII(HQS)2/ABEI/rGO initiated CL was at ∼440 nm, indicating that the emitter was the excited state oxidation product of ABEI (Fig. S5C†). It was deduced that the light emission may be due to the reaction of CoII(HQS)2/ABEI/rGO with the dissolved oxygen in alkaline solution. To prove the assumption, the effects of O2 and N2 on the CoII(HQS)2/ABEI/rGO CL reaction were investigated. As shown in Fig. S7B,† the CL intensity decreased in a nitrogen-saturated solution and increased in an oxygen-saturated solution, indicating that the dissolved O2 was involved the CL reaction. CoII(HQS)2/ABEI/rGO reacted with the dissolved O2 in alkaline solution, leading to CL emission.
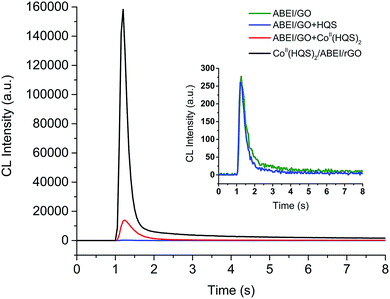 |
| Fig. 4 CL kinetic curves for reaction of ABEI/GO, ABEI/GO mixed with HQS, ABEI/GO mixed with CoII(HQS)2 and CoII(HQS)2/ABEI/rGO with the dissolved oxygen. Reaction conditions: 0.1 M NaOH. | |
The mechanism of CoII(HQS)2/ABEI/rGO–O2 system was investigated. It was reported that CoII complex could converse the dissolved oxygen to O2˙− in O2-containing and high alkaline solution.33 In this case, CoII(HQS)2 may react with the dissolved O2 in alkaline solution to form O2˙−. When the CoII(HQS)2 complexes were immobilized on the surface of ABEI/GO, the CL intensity increased significantly. This was attributed to that O2˙− could react with GO and ABEI to produce π–C
C˙ and ABEI˙− radicals. Finally, O2˙− radicals reacted with ABEI˙− to yield CL emission. Thus, the as-prepared CoII(HQS)2/ABEI/rGO could also act as an effective nanocatalytic reaction platform for the heterogenous CL reaction of ABEI with the dissolved oxygen. The possible reaction pathways are also shown in Scheme 2.
CL behavior in periodate system
Unlike H2O2, periodate is rather stable oxidant and can oxidize luminol to produce strong CL emission. It was reported that Co2+ and Mn2+ could catalyze the CL reaction between luminol and KIO4.34 Hence, the CL behavior of CoII(HQS)2/ABEI/rGO with KIO4 was investigated as shown in Fig. 5. The CoII(HQS)2/ABEI/rGO exhibited good CL emission in KIO4–NaOH system. ABEI/GO and the mixture of ABEI/GO with HQS only demonstrated weak CL emission. The mixture of ABEI/GO with CoII(HQS)2 showed significant CL emission. However, the CL intensity of CoII(HQS)2/ABEI/rGO–KIO4 was about 150 and 3 times higher than that of ABEI/GO and ABEI/GO + CoII(HQS)2, respectively. As shown in Fig. S5D,† the CL emission peak of CoII(HQS)2/ABEI/rGO–KIO4 was at ∼440 nm, indicating that the emitter was the excited state oxidation product of ABEI. As shown in Fig. S7C,† the CL intensity was positively correlated with the concentration of the dissolved O2. When CoII(HQS)2 was added into ABEI/GO–KIO4 system, the CL kinetic curve became sharp (Fig. 5, red line). The duration time of CL emission was greatly reduced to 2 seconds.
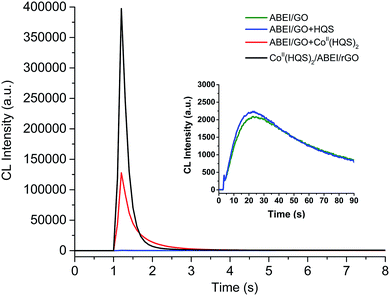 |
| Fig. 5 CL kinetic curves for reaction of ABEI/GO, ABEI/GO mixed with HQS, ABEI/GO mixed with CoII(HQS)2 and CoII(HQS)2/ABEI/rGO with periodate. Reaction conditions: 0.05 mM KIO4 in 0.05 NaOH. | |
The CL mechanism of CoII(HQS)2/ABEI/rGO with KIO4 was investigated. As shown in Fig. S7C,† the CL intensity was positively correlated with the concentration of the dissolved O2. It was reported that periodate anion (IO4−) could react with the dissolved oxygen in alkaline solution to produce O2˙−.35 When CoII(HQS)2 was added into ABEI/GO–KIO4 system, the CL kinetic curve became sharp (Fig. 5, red line). The duration time of CL emission was greatly reduced to 2 seconds. Periodate anion could coordinate with the cobalt(II) complex and accompanied by the intramolecular electron transfer to produce cobalt(III) complex, which decomposed to reactive periodate anion radicals (IVI).36 In this case, it is suggested that IO4− reacts with CoII(HQS)2 to follow similar pathways to produce IVI, which facilitated the generation of O2˙− to enhance the CL emission. Meanwhile, when the CoII(HQS)2 was functionalized on the surface of ABEI/GO to form CoII(HQS)2/ABEI/rGO, the CL intensity was further increased. The result indicated that GO also play an important role for the enhanced CL emission, which may be due to that GO stimulate the generation of O2˙−, π–C
C˙ and ABEI˙− radicals. The mechanism of CoII(HQS)2/ABEI/rGO–KIO4 CL system is also summarized as Scheme 2. Thus, the as-prepared CoII(HQS)2/ABEI/rGO could also act as an effective nanocatalytic reaction platform for the heterogenous CL reaction of ABEI with KIO4 in alkaline solution.
Dilution effect
As shown in Fig. S8,† the as-prepared CoII(HQS)2/ABEI/rGO hybrids was dark brown solution and the hybrids turn to more transparent when diluted. Usually, CL intensity would be declined with the decrease of the CL reagent concentration. However, the CL intensity increased with the increase of dilution times in the range of 1–15 times in CoII(HQS)2/ABEI/rGO–H2O2 system and decreased when dilution times further increased (Fig. 6A). The dilution-initiated CL enhancement was also observed in ABEI/GO–H2O2, ABEI/GO–O2 and ABEI/GO–KIO4 systems (Fig. 6D–F). However, the dilution-initiated CL enhancement was not observed in CoII(HQS)2/ABEI/rGO–O2 and CoII(HQS)2/ABEI/rGO–KIO4 system (Fig. 6B and C).
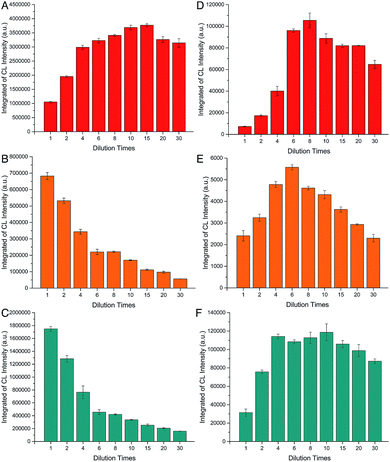 |
| Fig. 6 Effect of dilution on CL intensity of CoII(HQS)2/ABEI/rGO with (A) H2O2, (B) O2, (C) KIO4, and ABEI/GO with (D) H2O2, (E) O2, (F) KIO4. Reaction conditions: (A) and (D) 0.1 mM H2O2 in 0.01 M NaOH, (B) and (E) 0.1 M NaOH, (C) and (F) 0.05 mM KIO4 in 0.05 NaOH. | |
It is well known that GO with the sp2 domains were excellent fluorescence quenchers and the quenching efficiency of GO was significantly improved after reduction.37,38 As shown in Fig. 7, the effect of rGO formed via reduction of GO by ammonia and hydrazine on the ABEI–H2O2 CL system was investigated. The CL intensity (10 s) was quenched by 96.2% and 88.4% in the present of 0.05 mg mL−1 and 0.025 mg mL−1 rGO, respectively. The results demonstrated that reduced GO could quench effectively the ABEI CL emission and the dilution could decrease the quenching effect of reduced GO.
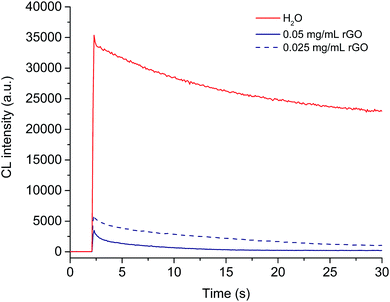 |
| Fig. 7 Effect of rGO formed via reduction of GO by ammonia and hydrazine on ABEI–H2O2 CL system. Reaction conditions: 0.2 mM ABEI dissolved in H2O (red curve), 0.05 mg mL−1 rGO (blue solid line) and 0.025 mg mL−1 rGO (blue dash line), 1 mM H2O2 in 0.1 M NaOH. | |
Therefore, in this case, the dilution of reduced GO in the hybrids to some extent was beneficial to decrease the quenching effect, leading to an increase in CL intensity. This is consistent with CL behavior of ABEI/GO–H2O2, ABEI/GO–O2 and ABEI/GO–KIO4 systems in the absence of CoII(HQS)2 when they were diluted. When the hybrids contained catalyst CoII(HQS)2, CoII(HQS)2 concentration should also decrease with the increase of dilution times, resulting in a decrease in CL intensity. On the other hand, the dilution would decrease CL quenching effect of reduced GO in the hybrids and increase the CL intensity. As a result, in CoII(HQS)2/ABEI/rGO–O2 and CoII(HQS)2/ABEI/rGO–KIO4 system, the CL signal showed a decrease with dilution times (Fig. 6B and 7C), which may be due to that dilution-initiated catalytic decrease dominated the CL behavior. Comparing CL kinetic curve of CoII(HQS)2/ABEI/rGO–H2O2 CL system (Fig. 3) with those of CoII(HQS)2/ABEI/rGO–O2 and CoII(HQS)2/ABEI/rGO–KIO4 systems (Fig. 4 and 5), the catalytic effect of CoII(HQS)2 in CoII(HQS)2/ABEI/rGO–H2O2 CL system is much stronger those in CoII(HQS)2/ABEI/rGO–O2 and CoII(HQS)2/ABEI/rGO–KIO4 systems. The dilution-initiated CL enhancement was also observed for CoII(HQS)2/ABEI/rGO–H2O2 CL system (Fig. 6A), which may be due to that dilution led to little decrease in catalytic effect and the decrease of CL quenching effect as main factor caused the CL enhancement.
Conclusions
In summary, a novel CoII(HQS)2/ABEI/rGO hybrids was synthesized via an economy, facile and simple strategy. ABEI and CoII(HQS)2 were successfully assembled onto the surface of GO by virtue of π–π stacking and coordination. It was found that the as-prepared CoII(HQS)2/ABEI/rGO hybrids could be used as excellent nanocatalytic reaction platforms for chemiluminescence (CL) when reacted with hydrogen peroxide, the dissolved oxygen and periodate in alkaline solution. In these CL reactions, CoII(HQS)2 could catalyze the generation of the reactive radicals on GO surface, such as hydroxyl radicals (HO˙), superoxide radicals (O2˙−), periodate anion radicals (IVI) and π-conjugated carbon radicals (π–C
C˙), followed by the reaction with ABEI to form ABEI˙−. ABEI˙− would further react with O2˙− to produce strong CL. It was also found that dilution-initiated CL enhancement in CoII(HQS)2/ABEI/rGO–H2O2 system but not in CoII(HQS)2/ABEI/rGO–O2 and CoII(HQS)2/ABEI/rGO–KIO4 systems. This was attributed to the competition of two processes: dilution decreased reduced GO quenching effect, leading to CL enhancement; dilution decreased CoII(HQS)2 concentration, resulting in a decrease in CL intensity. The former predominanted the CL behavior of CoII(HQS)2/ABEI/rGO–H2O2 system, whereas the later predominanted the CL behavior of CoII(HQS)2/ABEI/rGO–O2 and CoII(HQS)2/ABEI/rGO–KIO4 systems. This work provides new insight into physicochemical property of functionalized GO hybrids and excellent nanocatalytic platforms for CL reactions, which may find future applications in bioassays, biosensors, bioimaging and microchips.
Acknowledgements
The support of this research by the National Key Research and Development Program of China (Grant No. 2016YFA0201300) and the National Natural Science Foundation of China (Grant No. 21527807 and 21475120) are gratefully acknowledged.
Notes and references
- H. Kong, D. Liu, S. Zhang and X. Zhang, Anal. Chem., 2011, 83, 1867–1870 CrossRef CAS PubMed.
- N. Na, S. Zhang, S. Wang and X. Zhang, J. Am. Chem. Soc., 2006, 128, 14420–14421 CrossRef CAS PubMed.
- C. K. Lim, Y. D. Lee, J. Na, J. M. Oh, S. Her, K. Kim, K. Choi, S. Kim and I. C. Kwon, Adv. Funct. Mater., 2010, 20, 2644–2648 CrossRef CAS.
- Y. Su and Y. Lv, RSC Adv., 2014, 4, 29324–29339 RSC.
- Z. F. Zhang, H. Cui, C. Z. Lai and L. J. Liu, Anal. Chem., 2005, 77, 3324–3329 CrossRef CAS PubMed.
- H. Chen, F. Gao, R. He and D. Cui, J. Colloid Interface Sci., 2007, 315, 158–163 CrossRef CAS PubMed.
- R. Gill, R. Polsky and I. Willner, Small, 2006, 2, 1037–1041 CrossRef CAS PubMed.
- S. F. Li, X. M. Zhang, W. X. Du, Y. H. Ni and X. W. Wei, J. Phys. Chem. C, 2008, 113, 1046–1051 Search PubMed.
- H. Cui, W. Wang, C. F. Duan, Y. P. Dong and J. Z. Guo, Chem.–Eur. J., 2007, 13, 6975–6984 CrossRef CAS PubMed.
- Y. He and R. Peng, Nanotechnology, 2014, 25, 455502–455508 CrossRef PubMed.
- L. U. Syed, L. Z. Swisher, H. Huff, C. Rochford, F. Wang, J. Liu, J. Wu, M. Richter, S. Balivada and D. Troyer, Analyst, 2013, 138, 5600–5609 RSC.
- X. Yang, Y. Guo and A. Wang, Anal. Chim. Acta, 2010, 666, 91–96 CrossRef CAS PubMed.
- M. Liu, H. Zhang, J. Shu, X. Liu, F. Li and H. Cui, Anal. Chem., 2014, 86, 2857–2861 CrossRef CAS PubMed.
- L. Gao, X. He, L. Ju, X. Liu, F. Li and H. Cui, Anal. Bioanal. Chem., 2016, 408, 8747–8754 CrossRef CAS PubMed.
- D. R. Dreyer, S. Park, C. W. Bielawski and R. S. Ruoff, Chem. Soc. Rev., 2010, 39, 228–240 RSC.
- Y. Wang, Z. Li, J. Wang, J. Li and Y. Lin, Trends Biotechnol., 2011, 29, 205–212 CrossRef CAS PubMed.
- D. Liu, G. Huang, Y. Yu, Y. He, H. Zhang and H. Cui, Chem. Commun., 2013, 49, 9794–9796 RSC.
- X. Liu, Z. Han, F. Li, L. Gao, G. Liang and H. Cui, ACS Appl. Mater. Interfaces, 2015, 7, 18283–18291 CAS.
- S. Park and R. S. Ruoff, Nat. Nanotechnol., 2009, 4, 217–224 CrossRef CAS PubMed.
- D. Yang, A. Velamakanni, G. Bozoklu, S. Park, M. Stoller, R. D. Piner, S. Stankovich, I. Jung, D. A. Field and C. A. Ventrice, Carbon, 2009, 47, 145–152 CrossRef CAS.
- D. Frost, C. McDowell and I. Woolsey, Mol. Phys., 1974, 27, 1473–1489 CrossRef CAS.
- A. Das, B. Chakraborty and A. Sood, Bull. Mater. Sci., 2008, 31, 579–584 CrossRef CAS.
- W. Shen, Y. Yu, J. Shu and H. Cui, Chem. Commun., 2012, 48, 2894–2896 RSC.
- K. Soroka, R. S. Vithanage, D. A. Phillips, B. Walker and P. K. Dasgupta, Anal. Chem., 1987, 59, 629–636 CrossRef CAS.
- S. B. Raj, P. T. Muthiah, G. Bocelli and L. Righi, Acta Crystallogr., 2001, 57, 591–594 Search PubMed.
- G. Zhao, J. Li, X. Ren, C. Chen and X. Wang, Environ. Sci. Technol., 2011, 45, 10454–10462 CrossRef CAS PubMed.
- G. Merenyi and J. S. Lind, J. Am. Chem. Soc., 1980, 102, 5830–5835 CrossRef CAS.
- J. Lind, G. MerBnyi and T. E. Erikse, J. Am. Chem. Soc., 1983, 105, 7655–7661 CrossRef CAS.
- J. Weiss, Trans. Faraday Soc., 1935, 31, 1547–1557 RSC.
- J. M. Lin, X. Shan, S. Hanaoka and M. Yamada, Anal. Chem., 2001, 73, 5043–5051 CrossRef CAS PubMed.
- R. V. Prasad and N. V. Thakkar, J. Mol. Catal., 1994, 92, 9–20 CrossRef CAS.
- V. S. Shivankar and N. V. Thakkar, J. Sci. Ind. Res., 2005, 64, 496–503 CAS.
- L. L. Klopf and T. A. Nieman, Anal. Chem., 1983, 55, 1080–1083 CrossRef CAS.
- Q. Lin, A. Guiraúm, R. Escobar and F. Francisco, Anal. Chim. Acta, 1993, 283, 379–385 CrossRef CAS.
- N. P. Koutsoulis, D. L. Giokas, A. G. Vlessidis and G. Z. Tsogas, Anal. Chim. Acta, 2010, 669, 45–52 CrossRef CAS PubMed.
- M. A. Hussein, A. A. Abdel-Khalek and Y. Sulfab, J. Chem. Soc., Dalton Trans., 1983, 2, 317–321 RSC.
- J. Kim, L. J. Cote, F. Kim and J. Huang, J. Am. Chem. Soc., 2009, 132, 260–267 CrossRef PubMed.
- K. P. Loh, Q. Bao, G. Eda and M. Chhowalla, Nat. Chem., 2010, 2, 1015–1024 CrossRef CAS PubMed.
Footnotes |
† Electronic supplementary information (ESI) available. See DOI: 10.1039/c7ra06327j |
‡ The authors are co-first authors and contributed equally to this work. |
|
This journal is © The Royal Society of Chemistry 2017 |