DOI:
10.1039/C7RA06291E
(Paper)
RSC Adv., 2017,
7, 36416-36420
Synthesis, structure, and photocatalytic hydrogen evolution of a trimeric Nb/W addendum cluster†
Received
5th June 2017
, Accepted 10th July 2017
First published on 21st July 2017
Abstract
We present the second example of mixed Nb/W addendum based trimer, Cs12[(SiW9Nb3O38)3WO3(OH)3]·33H2O by utilizing in situ formed Keggin-type {SiW9(NbO2)3} unit as the secondary building block. The polyanion [(SiW9Nb3O38)3WO3(OH)3]12− incorporates three saturated niobium-substituted tungstosilicate {SiW9Nb3} clusters that are linked together by three Nb–O–Nb bridges and a tungsten joint. The compound has been thoroughly characterized by single crystal X-ray diffraction, UV-Vis spectroscopy, TG analysis, PXRD and FTIR spectra. In addition, the photocatalytic activities of the title compound and the two precursors A-α-Na10[SiW9O34]·23H2O and K7HNb6O19·13H2O for H2 evolution were also evaluated.
Introduction
Polyoxoniobates (PONbs)1,2 are a unique family of polynuclear anionic metal oxo clusters with properties suitable for many potential applications in catalysis, magnetism, biomedicine, materials science, and nanotechnology.3–6 This family has accelerated dramatically over the last 15 years, and a vast expansion of available PONbs has been reported.7–14 In this context, the class of Nb/W addendum heteropolyoxometalates develops slowly since the pioneering work reported by Finke and Droege in 1984.15 Recently, some other Nb/W addendum heteropolyoxometalate clusters have been communicated.16–37 However, most of them comprise a monomeric,16–23 dimeric18,24–27 or tetrameric18,19,27–29 structure. Key efforts include the synthesis and physical characterization of the above-mentioned Keggin-type monomeric triniobium-substituted polytungstosilicate [SiW9Nb3O40]7− and its dimeric form [Si2W18Nb6O77]8− by Finke et al.15 and the extensive efforts with the preparation and single-crystal X-ray structure of the Keggin-type monomeric triperoxoniobium-substituted clusters [XW9(NbO2)3O37]7− (X = P,16 Si,17 Ge,18 As19) and its peroxo-free clusters [XW9Nb3O40]7− (X = Si,15 Ge,18 As19), dimeric tungstoniobates [Si2W18Nb6O77]8−,15,24 [Si2W18Nb6O78]10− (ref. 25) and [Ge2W18Nb8O88]20−,18 supramolecular tetra-Keggin clusters [Nb4O6(Nb3XW9O40)4]20− (X = Si,29,30 Ge,18 As19) by Hill and Liu group, respectively. In 2012 and 2013, Liu and Su et al. reported the self-assembly of W/Nb mixed-addendum polyoxometalate and lanthanide/transition-metal-containing Keggin-type Nb/W mixed species, respectively.31,32 On the other hand, several Wells-Dawson-type niobium-substituted tungstophosphates, (P2W15Nb3O62)9−,20,21 [P2W17NbO62]7− (ref. 22) and [P2W12(NbO2)6O56]12−,23 [H6P2W12Nb4O59(NbO2)2]28− (ref. 26) were successively communicated by Finke, Hill and Yue group, respectively. In 2012, Liu group also reported two Wells-Dawson-type Nb/W-based lanthanide derivatives, [Ln6(H2O)38(P2W15Nb3O62)4]18− (Ln = Ce, Eu).33
It should be noted that our group has developed a new synthetic strategy, utilizing peroxo-niobium-substituted POM formed in situ as a secondary building block, to construct giant Nb/W addendum polyoxoanions and their transition-metal derivatives in recent years.27,28,34–36 In particular, the unprecedented hexameric cluster {Mn15(Nb6P2W12O62)6}, in which the six polyanions [Nb6P2W12O61]10− are alternately connected by four intriguing trinuclear {MnIII3} moieties and four {MnII} linkers, represents the first example of niobotungstate with single-molecule magnet and also the largest cluster in niobotungstate chemistry.35 The successful synthesis of these compounds has been thoroughly demonstrated that the NbO2 groups are actually more basic and reactive than their oxotungsten counterparts. Therefore, {SiW9(NbO2)3} or {P2W12(NbO2)6} formed in situ can indeed be regarded as a saturated building block to generate gigantic POM assemblies.
As our continuous work, herein we present the synthesis, structure of the second example of trimeric Keggin-type Nb/W mixed compound Cs12[(SiW9Nb3O38)3WO3(OH)3]·33H2O (1, Cs12-1a·33H2O), which has been characterized by single crystal X-ray diffraction, UV-Vis spectroscopy, TG analysis, PXRD and FTIR spectra. In addition, the photocatalytic H2 evolution activity of compound 1 was also investigated.
Results and discussion
Synthesis
Compound 1 was obtained by the addition of Fe(NO3)3·9H2O into [SiW9(NbO2)3O37]7− that was in situ formed from K7HNb6O19·13H2O (abbreviated as Nb6)37 and trilacunary A-α-Na10[SiW9O34]·23H2O (abbreviated as SiW9)38 in the presence of H2O2. The solution was adjusted to pH 2.3 and heated to 90 °C for 5 h, followed by the addition of CsCl (Scheme 1a). Interestingly, polyanion 1a can be only crystallized with the need for Cs+ cation (Scheme 1b). This is in agreement with the original report indicating an essential templating role of Cs+ in the formation of Keggin-based analogues.17–19,24,25 In addition, Fe(NO3)3·9H2O is essential for the formation of 1, although it does not appear in the structure (Scheme 1c). Such observations have also been observed in the formation of previous POM clusters.39,40
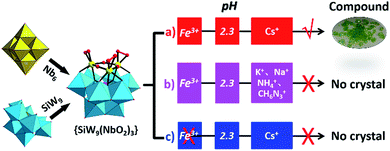 |
| Scheme 1 Synthetic routes leading to the isolation of polyanion 1a, highlighting the effect of cesium countercation and Fe(NO3)3·9H2O. | |
Structural analysis
Single crystal X-ray diffraction analysis reveals that 1 crystallizes in the trigonal space group R3m, exhibiting a trimeric cluster based on the classic Keggin-type unit {SiW9Nb3} (Fig. 1a and b). As expected, the structure of {SiW9Nb3} unit (Fig. 1c) comprises a trilacunary SiW9 fragment (Fig. 1d) with the vacant sites occupied by three NbO6 groups. The three {SiW9Nb3} units are linked to each other via two Nb–Ob–Nb (Ob: bridging oxygen atom) bridges and capped by an extra WO6 octahedron, resulting in a rare trimeric assemble. Alternatively, 1a can be viewed as three SiW9 fragments supporting an unprecedented {WNb9} core (Fig. 1e).
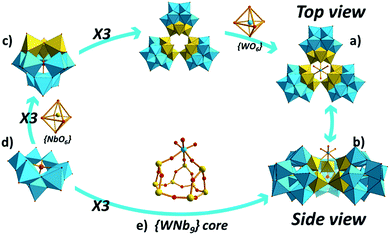 |
| Fig. 1 Combined polyhedral/ball-and-stick representation of polyanion 1a in different direction (a and b); the {SiW9Nb3} unit (c); the {SiW9} fragment (d); the {WNb9} core (e). All cations and solvent water molecules have been omitted for clarity. WO6 octahedra (blue), NbO6 octahedra (yellow), Si balls (pink), Nb balls (yellow), and O balls (red). | |
In 1a, each of the Nb and W atoms is coordinated by six oxygen atoms, resulting in an octahedral coordination geometry, whereas all the Si atoms exhibit conventional tetrahedral coordination polyhedra. In 1a, the W–O, Nb–O and Si–O bond lengths are in the range of 1.70(2)–2.368(19), 1.82(3)–2.32(3) and 1.61(2)–1.65(3) Å, respectively.
As shown in Table S1,† bond valence sum (BVS) calculations for 1a are consistent with Si, W and Nb being in the +4, +6 and +5 oxidation states, respectively.41 BVS calculations for all oxygen atoms in 1a indicate that three terminal oxygen atoms (O25) on the W6 site (Fig. S1†) are mono-protonated with the value of 1.27, resulting in an {WO3(OH)3} unit.
IR spectra
The Fourier transform infrared spectra (FTIR) of 1, SiW9 and {SiW9(NbO2)3} are shown in Fig. 2 and S2.† They all show strong bands in the range of 1050–950 cm−1, as well as medium or strong bands in the range of 950–850 cm−1, associated with antisymmetric stretching vibrations of the Si–O and the terminal W
O bonds, respectively. The medium bands at 850–680 cm−1 correspond to the antisymmetric stretching vibrations of the M–Ob bridges. These results confirm that the Keggin-type framework remains intact under the condition of the synthesis. Compared with that of {SiW9(NbO2)3}, the significant changes in FTIR spectrum of 1 are the disappearance of weak intensity band between 860 and 870 cm−1 and the appearance of medium intensity band at 749 cm−1, which is characteristic of the antisymmetric stretching vibrations of peroxo groups18,29 and Nb–O–Nb bridges,15,18,19 respectively. This is in good agreement with the solid-state structure.
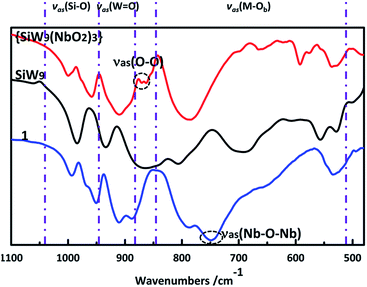 |
| Fig. 2 FTIR spectra of 1, SiW9 and {SiW9(NbO2)3} in the region between 1100 to 450 cm−1. | |
Photocatalytic studies
To demonstrate the photocatalytic H2 evolution activity of 1, 100 mg 1 and 5.2 mL H2PtCl6 (1 mM) were dissolved in 100 mL of 20% methanol–water mixed solution (4/1, volume ratio), which was irradiated under full spectrum using a 300 W Xe lamp in a quartz cell. In this system, 1 was used as light photosensitizer and catalyst in the presence of a Pt co-catalyst, while methanol was acted as a sacrificial electron donor, which is the source of the electrons required in the reduction semi-reaction of water. As shown in Fig. 3, the amount of the evolved H2 for compound 1 increased continuously, and the total evolved H2 over 5 h was 1003.0 μmol g−1 (line (a)), and the average H2 evolution rate was 200.6 μmol h−1 g−1.
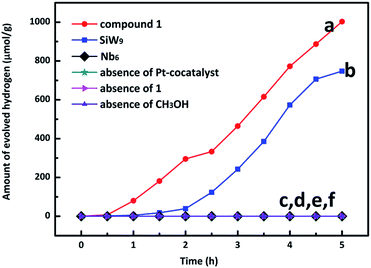 |
| Fig. 3 Time dependence of photocatalytic H2 production over different system: (line (a)) compound 1 (red), (line (b)) SiW9 (blue) and (line (c)) Nb6 (black); (lines (d–f)) the absence of Pt-cocatalyst (cyan), 1 (rose red), CH3OH (purple). | |
For comparison, the use of the precursors SiW9 or Nb6 was also investigated under otherwise identical reaction conditions (lines (b) and (c) in Fig. 3), the total evolved H2 of SiW9 over 5 h was 747.6 μmol g−1, whereas the Nb6 is almost inactive. To investigate the important roles of Pt co-catalyst, 1 and CH3OH in the photocatalytic process, three blank experiments were carried out with no H2 evolution observed (lines (d–f) in Fig. 3), indicating that Pt co-catalyst, 1 and CH3OH play an indispensable role in light harvesting for photocatalysis. On the other hand, the increase in H2 evolution of 1 compared to SiW9 may be attributed to the introduction of niobium, which is in agreement with our previous result.27 Furthermore, the band gap is reduced from 3.51 eV for SiW9 to 2.74 eV for 1, in turn, indicating a positive photocatalytic effect (Fig. S3†).
Conclusion
In summary, a new Keggin-type based trimeric Nb/W mixed cluster Cs12[(SiW9Nb3O38)3WO3(OH)3]·33H2O (1, Cs12-1a·33H2O) has been synthesized by utilizing the in situ formed saturated Keggin-type {SiW9(NbO2)3} unit as the secondary building block. Polyanion 1a incorporates three Keggin-type saturated niobium-substituted tungstosilicate {SiW9Nb3} clusters that are linked to each other by Nb–O–Nb linkages and a tungsten joint. Again, the successful synthesis of 1 demonstrated that the oxoniobium(V) surface is more basic and reactive than its oxotungsten(VI) counterpart and may reactive to transition-metal or lanthanide, providing an alternative perspective for the assemble of novel polyoxometalate derivatives. In addition, 1 exhibits photocatalytic H2 evolution activity.
Experimental section
Materials and methods
All the reagents were obtained from commercial sources and used as received. All solvents were used without further purification. K7H[Nb6O19]·13H2O37 and A-α-Na10[SiW9O34]·23H2O38 were prepared using literature methods. The IR spectra (using KBr in pellets) were recorded on a Bruker VERTEX 70 IR spectrometer (4000–450 cm−1). X-ray powder diffraction (PXRD) spectral data was recorded on a Bruker AXS D8 Advance diffractometer with Cu Kα radiation in the angular range 2θ = 5–45° at 293 K. UV-Vis spectra were obtained with a U-4100 spectrometer at room temperature. Thermogravimetric (TG) analysis was performed under N2 atmosphere on a NETZSCH STA 449 F5 Jupiter thermal analyzer with the heating rate of 10 °C min−1 from 30 to 600 °C. Photocatalytic reactions were carried out in a CEL-SPH2N reaction vessel with a magnetic stirrer at room temperature. The produced H2 was analyzed by a GC-7920 instrument with N2 as a carrier gas.
Synthesis of 1. K7H[Nb6O19]·13H2O (0.5 g, 0.36 mmol) was dissolved in a solution consisting of 7.5 mL of 30% aqueous H2O2 and 60 mL of water. Under rapidly stirring, 6 mL HCl (1.0 M) was added dropwise, while powdered A-α-Na10[SiW9O34]·23H2O (3.44 g, 1.20 mmol) was added in a single step. Ten minutes later, a solution of Fe(NO3)·9H2O (0.31 g, 1.1 mmol) in H2O (2 mL) was added. The pH of the resulting mixture was adjusted to 2.3 and then heated to 90 °C for 5 hours. After this period, the mixture was cooled to room temperature and filtered, followed by the addition of CsCl (0.3 g, 1.9 mmol). The solution was then stirred for 35 minutes and filtered. The resulting filtrate was kept at room temperature to allow slow evaporation for about five weeks (yield 0.29 g, 12% based on Nb). IR (KBr, cm−1): ν = 997 (s), 950 (m), 910 (w), 881 (m), 749 (m).
X-ray crystal-structure analyses
Suitable single crystals were selected from their respective mother liquors and placed in a thin glass tube. X-ray diffraction intensity was recorded on a Bruker Apex-II CCD diffractometer at 296(2) K with MoKα monochromated radiation (λ = 0.71073 Å). Structure solution and refinement were carried out by using the SHELXS-97 and SHELXL-2014 program packages42,43 for 1. Selected details of the data collection and structural refinement of compound 1 can be found in Table 1. Further details of the crystal structure investigation can be obtained from the Fachinformationszentrum Karlsruhe, 76344 Eggenstein-Leopoldshafen, Germany (fax: +49-7247-808-666; e-mail: E-mail: crysdata@fiz-karlsruhe.de) on quoting the depository number CSD 433156.
Table 1 Crystal data and structure refinement
|
1 |
R1 = ∑||Fo| − |Fc||/∑|Fo|. wR2 = {∑[w(Fo2 − Fc2)2]/∑[w(Fo2)2]}1/2. |
Empirical formula |
Cs12H69Nb9O153Si3W28 |
Formula weight |
10 180.26 |
Crystal system |
Trigonal |
Space group |
R3m |
a/Å |
30.4555(13) |
b/Å |
30.4555(13) |
c/Å |
15.9433(13) |
γ/deg |
120 |
V/Å3 |
12 806.8(15) |
Z |
3 |
Dc/g cm−3 |
3.798 |
μ/mm−1 |
21.980 |
F000 |
12 609 |
Crystal size/mm3 |
0.37 × 0.25 × 0.21 |
Reflns collected |
21 257 |
Indep reflns |
5324 |
Rint |
0.0913 |
Goodness-of-fit on F2 |
1.058 |
R1[I > 2σ(I)]a |
R1 = 0.0473, wR2 = 0.1173 |
wR2 (all data)b |
R1 = 0.0543, wR2 = 0.1213 |
Flack parameter |
0.535(19) |
Acknowledgements
This work was supported by the National Natural Science Foundation of China (Grant Numbers 21371048 and 21671056), the NSF from Henan Province (grant number 152102410027) and 2015 Young Backbone Teachers Foundation from Henan Province (2015GGJS-017). D. Z. gratefully acknowledges financial support from China Scholarship Council (201608410252).
References
- M. T. Pope and A. Müller, Angew. Chem., Int. Ed. Engl., 1991, 30, 34–48 CrossRef.
- D.-L. Long, E. Burkholder and L. Cronin, Chem. Soc. Rev., 2007, 36, 105–121 RSC.
- S.-S. Wang and G.-Y. Yang, Chem. Rev., 2015, 115, 4893–4962 CrossRef CAS PubMed.
- J. M. Clemente-Juan, E. Coronado and A. Gaita-Ariño, Chem. Soc. Rev., 2012, 41, 7464–7468 RSC.
- A. Proust, B. Matt, R. Villanneau, G. Guillemot, P. Gouzerh and G. Izzet, Chem. Soc. Rev., 2012, 41, 7605–7622 RSC.
- Y. Gao, J. E. S. Szymanowski, X. Sun, P. C. Burns and T. Liu, Angew. Chem., Int. Ed., 2016, 55, 6887–6891 CrossRef CAS PubMed.
- M. Nyman, F. Bonhomme, T. M. Alam, M. A. Rodriguez, B. R. Cherry, J. L. Krumhansl, T. M. Nenoff and A. M. Sattler, Science, 2002, 297, 996–998 CrossRef CAS PubMed.
- M. Nyman, Dalton Trans., 2011, 40, 8049–8058 RSC.
- H.-L. Wu, Z.-M. Zhang, Y.-G. Li, X.-L. Wang and E.-B. Wang, CrystEngComm, 2015, 17, 6261–6268 RSC.
- Z. Liang, D. Zhang, P. Ma, J. Niu and J. Wang, Chem.–Eur. J., 2015, 21, 8380–8383 CrossRef CAS PubMed.
- J.-H. Son and W. H. Casey, Chem. Commun., 2015, 51, 12744–12747 RSC.
- J.-H. Son and W. H. Casey, Chem. Commun., 2015, 51, 1436–1438 RSC.
- Y.-T. Zhang, C. Qin, X.-L. Wang, P. Huang, B.-Q. Song, K.-Z. Shao and Z.-M. Su, Inorg. Chem., 2015, 54, 11083–11087 CrossRef CAS PubMed.
- L. Jin, X.-X. Li, Y.-J. Qi, P.-P. Niu and S.-T. Zheng, Angew. Chem., Int. Ed., 2016, 55, 13793–13797 CrossRef CAS PubMed.
- R. G. Finke and M. W. Droege, J. Am. Chem. Soc., 1984, 106, 7274–7277 CrossRef CAS.
- M. K. Harrup, G.-S. Kim, H. Zeng, R. P. Johnson, D. VanDerveer and C. L. Hill, Inorg. Chem., 1998, 37, 5550–5556 CrossRef CAS PubMed.
- G.-S. Kim, H. Zeng, C. L. Hill and others, Bull. Korean Chem. Soc., 2003, 24, 1005–1008 CrossRef CAS.
- S.-J. Li, S.-X. Liu, C.-C. Li, F.-J. Ma, D.-D. Liang, W. Zhang, R.-K. Tan, Y.-Y. Zhang and L. Xu, Chem.–Eur. J., 2010, 16, 13435–13442 CrossRef CAS PubMed.
- S.-J. Li, S.-X. Liu, C.-C. Li, F.-J. Ma, W. Zhang, D.-D. Liang, R.-K. Tan, Y.-Y. Zhang and Q. Tang, Inorg. Chim. Acta, 2011, 376, 296–301 CrossRef CAS.
- D. J. Edlund, R. J. Saxton, D. K. Lyon and R. G. Finke, Organometallics, 1988, 7, 1692–1704 CrossRef CAS.
- W. W. Laxson, S. Özkar and R. G. Finke, Inorg. Chem., 2014, 53, 2666–2676 CrossRef CAS PubMed.
- D. A. Judd, J. H. Nettles, N. Nevins, J. P. Snyder, D. C. Liotta, J. Tang, J. Ermolieff, R. F. Schinazi and C. L. Hill, J. Am. Chem. Soc., 2001, 123, 886–897 CrossRef CAS PubMed.
- D. A. Judd, Q. Chen, C. F. Campana and C. L. Hill, J. Am. Chem. Soc., 1997, 119, 5461–5462 CrossRef CAS.
- J. Rhule, I. Weinstock, C. Hill and others, Chem. Commun., 1999, 1651–1652 Search PubMed.
- G.-S. Kim, H. Zeng, W. A. Neiwert, J. J. Cowan, D. VanDerveer, C. L. Hill and I. A. Weinstock, Inorg. Chem., 2003, 42, 5537–5544 CrossRef CAS PubMed.
- Y. Ren, Y. Hu, Y. Shan, Z. Kong, M. Gu, B. Yue and H. He, Inorg. Chem. Commun., 2014, 40, 108–111 CrossRef CAS.
- D. Zhang, C. Zhang, P. Ma, B. S. Bassil, R. Al-Oweini, U. Kortz, J. Wang and J. Niu, Inorg. Chem. Front., 2015, 2, 254–262 RSC.
- D. Zhang, Z. Liang, S. Xie, P. Ma, C. Zhang, J. Wang and J. Niu, Inorg. Chem., 2014, 53, 9917–9922 CrossRef CAS PubMed.
- G.-S. Kim, H. Zeng, D. VanDerveer and C. L. Hill, Angew. Chem., Int. Ed., 1999, 38, 3205–3207 CrossRef CAS PubMed.
- Z.-X. Yang, P. Huang, L. Zhao, M. Zhang, Y.-T. Zhang and Z.-M. Su, Inorg. Chem. Commun., 2014, 44, 195–197 CrossRef CAS.
- S.-J. Li, S.-X. Liu, N.-N. Ma, Y.-Q. Qiu, J. Miao, C.-C. Li, Q. Tang and L. Xu, CrystEngComm, 2012, 14, 1397–1404 RSC.
- P. Huang, C. Qin, X.-L. Wang, C.-Y. Sun, Y.-Q. Jiao, Y. Xing, Z.-M. Su and K.-Z. Shao, ChemPlusChem, 2013, 78, 775–779 CrossRef CAS.
- C.-C. Li, S.-X. Liu, S.-J. Li, Y. Yang, H.-Y. Jin and F.-J. Ma, Eur. J. Inorg. Chem., 2012, 2012, 3229–3234 CrossRef CAS.
- D. Zhang, S. Li, J. Wang and J. Niu, Inorg. Chem. Commun., 2012, 17, 75–78 CrossRef CAS.
- D. Zhang, F. Cao, P. Ma, C. Zhang, Y. Song, Z. Liang, X. Hu, J. Wang and J. Niu, Chem.–Eur. J., 2015, 21, 17683–17690 CrossRef CAS PubMed.
- H. Wang, Z. Liang, Y. Wang, D. Zhang, P. Ma, J. Wang and J. Niu, Dalton Trans., 2016, 45, 15236–15241 RSC.
- C. M. Flynn Jr and G. D. Stucky, Inorg. Chem., 1969, 8, 178–180 CrossRef.
- G. Hervé and A. Tezé, Inorg. Chem., 1977, 16, 2115–2117 CrossRef.
- P. Huang, C. Qin, Z.-M. Su, Y. Xing, X.-L. Wang, K.-Z. Shao, Y.-Q. Lan and E.-B. Wang, J. Am. Chem. Soc., 2012, 134, 14004–14010 CrossRef CAS PubMed.
- P. Huang, C. Qin, Y. Zhou, Y.-M. Hong, X.-L. Wang and Z.-M. Su, Chem. Commun., 2016, 52, 13787–13790 RSC.
- I. D. Brown and D. Altermatt, Acta Crystallogr., Sect. B: Struct. Sci., 1985, 41, 244–247 CrossRef.
- G. M. Sheldrick, Acta Crystallogr., Sect. A: Found. Crystallogr., 2008, 64, 112–122 CrossRef CAS PubMed.
- G. M. Sheldrick, Acta Crystallogr., Sect. C: Cryst. Struct. Commun., 2015, 71, 3–8 CrossRef PubMed.
Footnote |
† Electronic supplementary information (ESI) available: Table, figures, FTIR spectra, UV-Vis spectroscopy, TG analysis and PXRD. See DOI: 10.1039/c7ra06291e |
|
This journal is © The Royal Society of Chemistry 2017 |