DOI:
10.1039/C7RA05854C
(Paper)
RSC Adv., 2017,
7, 31212-31220
Self-healable hydrogels with NaHCO3 degradability and a reversible gel–sol–gel transition from phenolic ester containing polymers†
Received
25th May 2017
, Accepted 9th June 2017
First published on 19th June 2017
Abstract
Due to their fantastic self-healing property, similar to that of organisms, self-healable hydrogels have attracted considerable attention in recent years. Herein, self-healable hydrogels with degradability in NaHCO3 were prepared and showed a reversible gel–sol–gel transition under a variety triggers. The hydrogel was prepared from poly(N,N-dimethylacrylamide-stat-4-formylphenyl acrylate), P(DMA-stat-FPA), and dihydrazide with dynamic acylhydrazone and disulfide cross-linking. With base labile phenolic ester bond connections, the hydrogel can be degraded by the extremely mild base, NaHCO3 solution. With a dihydrazide compound as a cross-linker, the hydrogel formed and self-healed without any additional catalysis or stimulus. Moreover, the self-healable hydrogels showed a reversible gel–sol–gel transition under various conditions, including pH, redox and group ratios, based on the reversible characteristics of acylhydrazone and disulfide bonds. With these superior properties, the developed hydrogel holds great potential for applications in many biomedical fields, including tissue engineering, drug delivery carriers and biosensors.
Introduction
The self-healing and self-repairing property is the ability to heal spontaneously after damage, and is one of the most fascinating characteristics of living creatures compared to their non-living counterparts. Accordingly, living creatures can repair damage to their organs when alive, and the self-healing property vanishes after their death. This fascinating self-healing feature has inspired researchers to design self-healable artificial materials to mimic the self-healing process of living creatures. As a result, the study of self-healable materials has made great progress based on both chemical and physical cross-linking in the past decade.1–7 Accordingly, these materials are defined as self-healable chemical6 and physical materials8–15 respectively.
Besides physical cross-linked ones, self-healable materials based on dynamic chemical bonds have improved dimensional stability and solvent resistance. The reversible Diels–Alder reaction under heating, the cycloaddition reaction of cinnamate and reshuffling reaction of trithiocarbonates under UV triggers are used to prepare self-healable polymer materials.2,16,17 The Cheng group designed dynamic urea bonding for reversible cross-linking to prepare self-healable polymer materials, and a self-healable polymer material containing carboxylate/amine bonds and bulky N-substituents was designed.5 Imato et al. had reported dynamic materials with diarylbibenzofuranone employed as a dynamic covalent unit, and the polymer material can self-heal its cracks at room temperature.18 A series of imine bonds (Schiff-base) based on reversible reaction of amido group and carbonyl group were also applied to prepare self-healable materials and gels as reported.19–21 The self-healable hydrogel containing reversible oxime bond was prepared from P(DMA-stat-DAA) with propanediylbishydroxylamine dihydrochloride as cross-linker, which can be degraded by monofunctional alkoxyamine.22 Deng et al. designed novel self-healable polymeric gels based on dynamic covalent bond of acylhydrazone, the gels showed gel–sol–gel transition under various acidity and drawn great attentions.23–25 Thermo-responsive hydrogel based on acylhydrazone were also reported in our previous publication.26 Other self-healable polymer materials and gels with disulfide bond with redox reversibility,27 boronic esters bond were also published in the past few years.28,29 Among above self-healable materials and gels, the real “self-healable” property without any additional stimulus or triggers similar to that of organisms are greatly preferred for bio-applications, and this property can help us to understand the self-healing mechanism of living creatures. Hydrogels based on acylhydrazone can be formed and self-healed without any stimulus in water; on the other hand, the hydrazide group would not react with the ester group. These properties endows the acylhydrazone containing self-healable materials with great potential application in bioscience and biotechnology.
Besides self-healing property, degradability is a very important property for synthetic polymer materials, especially for cross-linked materials. The degradation of the materials can avoid by-effects after their tasks are completed. Although the dynamic covalent bonds and the active ester bonds can be degraded under certain stimulus by their nature,24,30–32 the conditions are not mild or bio-compatible enough. As a result, degradable self-healable material are rarely reported. There were some publications about degradable self-healable polymer material reported recently, but the degradation of the network is still based on the reversible reaction of Schiff-base.19,33 As a result, large amount of solvent or acid is required to dilute the gelator of consume the amine. Based on our previous study, the phenolic ester bond is extremely basic labile that can be cleaved by NaHCO3.34 When the phenolic ester was imported into the self-healable hydrogel, it is possible to prepare novel self-healable hydrogel that can be degraded under extremely mild base. We have reported self-healable organic polymer gels that can be degraded in Na2CO3 solutions,35 but the self-healable hydrogel that can be degraded by NaHCO3 has not studied up to now.
In this work, self-healable hydrogels were prepared based on water soluble P(DMA-stat-FPA) with pendant aldehyde group via phenolic ester connection. Result showed that self-healable hydrogels with reversible gel–sol–gel transition were prepared successfully based on dynamic acylhydrazone bond. Moreover, the hydrogels can be degraded by extremely mild base of NaHCO3. To the best of our knowledge, there is no precedent report on the degradation of self-healing hydrogels based on cleavage of phenolic bond in extremely mild base of NaHCO3. This kind of degradable self-healable hydrogel without addition of catalyst have improved potential applications and bio-safety in bioscience fields, such as tissue engineering, drug delivery carriers, biosensors, etc.
Experimental
Materials
N,N-Dimethylacrylamide (DMA), dimethyl 3,3′-dithiodipropionate, DL-dithiothreitol (DTT) and adipic dihydrazide (ADH) were purchased from Macklin biochemical Co Ltd. DMA was passed through a short column filled with basic alumina to remove inhibitor prior to copolymerization. 3,3′-Dithiobis(propionohydrazide)dithiodipropionic acid dihydrazide (DTDPH) was synthesized from dimethyl 3,3′-dithiodipropionate according to literature.24 4-Hydroxybenzaldehyde, acryloyl chloride, tosyl chloride were purchased from Maya Reagent Co. Poly(ethylene oxide) (PEO, DP = 45, 90 and 227 separately) were supplied by Guangfu Fine Chemical Research Institute and used to prepare PEO dihydrazide (PEO DH) according to literature.23 The chain transfer agent S-1-dodecyl-S′-(R,R′-dimethyl-R′′-acetic acid) trithiocarbonate (DDMAT) was synthesized according to previous report.36 2,2′-Azobisisobutyronitrile (AIBN, Kermel, 99%) was recrystallized in ethanol twice before use. Other chemicals including hydrazine hydrate, 80%, H2O2, HCl, triethylamine, NaHCO3, Na2CO3, anhydrous, MgSO4 and solvents (THF, CH2Cl2, toluene, dioxane, DMF, ethyl acetate, petroleum ether, etc) were supplied by Kermel Chemical Reagent Co. and used as received.
Characterizations
1H NMR characterization were carried out on a Bruker 600 MHz spectrometer (Avance III, Bruker); the samples were dissolved in CDCl3 and the characterization was performed at room temperature. A Varian 600 IR spectrometer was used to obtain the Fourier-transform infrared (FT-IR) spectra. The samples were dissolved in solvents and casted on a KBr plate for characterization. Gel permeation chromatography (GPC) characterization was performed with THF as the eluent and PS as the standard. The Tg of the copolymer was determined by differential scanning calorimetry (Diamond DSC, Perkin Elmer), the sample was heated to 200 °C and then cooled to 0 °C, the Tg value was collected from the second run at a heating rate of 20 °C min−1. Thermogravimetric analysis (TGA) was carried out on a Pyris 1 instrument (Perkin Elmer), the samples were heated from 30 °C to 600 °C with a heating rate of 20 °C min−1 under N2 atmosphere. The lyophilized hydrogel samples were observed on a JSM-7500 field-emission scanning electron microscope (FE-SEM) with operating voltage of 10 kV and the images were recorded by a CCD camera. The samples were mounted on an aluminum specimen mounts and coated with Au for observations. The UV spectra of the monomer, copolymer and degraded products were scanned with a Shimadzu WV-2550 UV-vis spectrophotometer to compare the structure change under various conditions. Rheological properties of the hydrogels were measured on a TA AR2000ex rheometer with oscillatory mode at 25 °C.
Synthesis of FPA and preparation of P(DMA-stat-FPA) through RAFT polymerization
The 4-formylphenyl acrylate (FPA) was prepared by the reaction of 4-hydroxybenzaldehyde with acryloyl chloride and purified according to our previous report and illustrated in Scheme 1 (top).4,35 4-Hydroxybenzaldehyde and acryloyl chloride were reacted in anhydrous CH2Cl2 (DCM) with triethylamine as catalyst, the product of FPA was purified by silica gel chromatography and used for synthesis of functional copolymers.
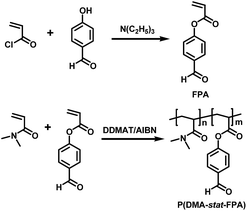 |
| Scheme 1 Synthesis of FPA and preparation of P(DMA-stat-FPA) through RAFT polymerization. | |
The P(DMA-stat-FPA) with different compositions were prepared through RAFT copolymerization mediated by DDMAT with AIBN initiation. The procedure of the copolymerization is illustrated in Scheme 1 (bottom) and described as follows. DDMAT (72.8 mg, 0.2 mmol), DMA (2.18 g, 22 mmol), FPA (0.39 g, 2.2 mmol) and AIBN (4.9 mg, 0.06 mmol) were dissolved in 3 mL dioxane in a 25 mL reaction tube. The tube was sealed with a rubber plug and the oxygen in the mixture was removed through three freeze–pump–thaw cycles. Then the tube was immersed in a 60 °C oil bath and the polymerization was performed for 24 h under N2 with continuous stirring.10,37–39 The polymerization was stopped by opening the tube to air and cooled to room temperature, the product was precipitated in petroleum ether/ethyl acetate (8/2) three times and dried under vacuum. The molecular weight of the polymer was calculated based on the monomer conversion by weighing the product. The composition of P(DMA-stat-FPA) was calculated by comparing the corresponding peak areas on 1H NMR spectrum.
Thermal properties of the copolymer and the hydrogel
The DSC characterization was carried out to investigate the influence of the composition on Tg of the copolymers. The samples were heated to 200 °C at 20 °C min−1 and cooled to 0 °C, the heating curve of the second run was collected to obtain the Tg value of the copolymers. The TGA characterizations were carried out to compare the stability of the copolymer and the strength of the chemical bonds, the samples were heated from room temperature to 600 °C at 20 °C min−1 heating rate. The copolymer and freeze dried hydrogels were characterized to determine the decomposition temperature of the phenolic ester bond.
Preparation of self-healable hydrogels
To study the hydrogel formation, the P(DMA-stat-FPA) copolymers with different compositions were dissolved in glass vials to form a homogeneous solution, then stoichiometric DTDPH or PEO dihydrazide solution as cross-linker was added into the vial. The vial was shaken at room temperature until a clear solution with total concentration of 10% was obtained. Then the solution was put into the round moulds to form hydrogel for self-healing and rheology studies, hydrogels formed in vials were used to determine the gel–sol–gel transition under a variety of triggers. Gel formation was confirmed by vial inversion and characterized by rheometry. Rheology tests and self-healing properties were investigated after clear hydrogel was formed and incubated for at least 24 h.
Rheological investigation of hydrogel and mechanical properties
The rheological properties of the hydrogels were measured on a TA AR2000ex rheometer with an oscillating mode, parallel aluminum plate with 25 mm diameter was used for the characterizations. The gap was fixed between 800–1000 μm for all the measurements; the frequency scan was carried out from 0.03–100 rad s−1 and the strain of 5% was selected within the linear viscoelastic regime. All measurements were conducted under N2 protection.
Self-healing study of hydrogels
Self-healing process of the hydrogels were carried out as follows: first, hydrogel was prepared in round mould in a sealed desiccator saturated with moisture. After incubated for 24 h, the gel plate was cut into two pieces across the center and put back into the original mould with cutting surfaces contacted closely. The mould was put back into the desiccator again and incubated for 24 h in saturated moisture. Self-healing results was confirmed by subjecting to gravity and stretching the healed hydrogel with tweezers from both sides perpendicular to the cutting line. The experiment was repeated by cutting the healed hydrogel in a perpendicular direction. Each time the healed hydrogel was incubated for 24 h before being cut again.
UV-vis absorbance of the FPA, P(DMA-stat-FPA) and the degraded product
The UV spectra of the copolymer under different conditions were obtained on a Shimadzu WV-2550 UV-vis spectrophotometer with the scanning range of 200–600 nm wavelengths. To compare the cleavage of the phenolic ester bond, 10 mg of P(DMA104-stat-FPA8) was dissolved in 1 mL 2% NaHCO3 and Na2CO3 solution respectively and stirred for 24 h, then UV-vis characterization were carried out after diluted to proper concentration. The degraded hydrogel was also characterized after dilution.
Morphology of the hydrogel though SEM observation
To observe the morphology of the self-healable hydrogels, the hydrogel samples were lyophilized and fractured in liquid nitrogen to preserve the original morphology. The fracture surfaces were observed by a JSM-7500 FE-SEM after coated with Au. The morphology and the cross-linking density of the hydrogels were compared by examination of the SEM images.
Gel–sol–gel transition under a variety of reversible triggers
To investigate the acid responsiveness of the hydrogel, CF3CO2H, HCl (5 M) was dropped onto the top of the hydrogel in a vial. The appearance change was visualized at different time intervals to monitor the gel–sol transition. After the hydrogel was dissolved, triethylamine was added to neutralize the acid with shaking and the gel–sol–gel transitions were recorded with a digital camera. The other hydrogels were investigated in the same process.
The disulfide bond is reversible under redox, which can endow the hydrogel with redox induced gel–sol–gel transition and self-healing properties.27 3 equivalent of DTT based on disulfide bond was added into the hydrogel with DTDPH as cross-linker and shook continuously to visualize the gel–sol transition. After the hydrogel was transited into liquid, stoichiometric H2O2 was added to investigate the sol–gel transition and the process was recorded by a digital camera.
The reversible acylhydrazone exchange can also be performed between hydrogel and excess of dihydrazine compounds to obtain linear polymer with pendant hydrazine groups. 3 times equivalent of dihydrazine in water solution (10%) was added into the hydrogel and the vial was shaken periodically to observe the gel–sol transition. After the hydrogel was transformed into a clear solution, P(DMA-stat-FPA) in 10% solution was added to regulate the group ratio to 1
:
1, the hydrogel formation was observed and recorded with a digital camera.
Degradation of self-healable hydrogels under mild base
The degradation of the hydrogels with phenolic ester cross-linking were carried out as follows: the NaHCO3 solution (0.22 g 10%) was dropped on the hydrogel in a glass vial (1 g with 10% gelator) to regulate the resultant NaHCO3 concentration of 2%. The degradation of the hydrogel was confirmed by leaning the vial compared to as prepared hydrogel. The degraded solution was diluted and scanned by UV-vis spectrometer to compare the UV spectrum with the copolymer.
Result and discussion
Synthesis of 4-formylphenyl acrylate and polymerization of P(DMA-stat-FPA)
The FPA was synthesized by reaction of 4-hydroxybenzaldehyde and acryloyl chloride in DCM according to our previous report,35 and the structure was characterized by 1H NMR. Polymerization of P(DMA-stat-FPA) was initiated by AIBN and mediated by DDMAT (Scheme 1). The molecular weight of the copolymer was evaluated by monomer conversion and the composition of the copolymer was determined by 1H NMR. The 1H NMR spectra of FPA and P(DMA104-stat-FPA8) are shown in Fig. 1. The peak c (7.91 ppm) and e (9.97 ppm) on spectrum of P(DMA104-stat-FPA8) were originated from FPA, the peak g (2.91 ppm) was derived from DMA, which confirmed the copolymerization reaction, as shown in Fig. 1 (bottom). The composition of the copolymer was calculated by comparing the peak area of c (7.91 ppm) and g (2.91 ppm). Based on the 1H NMR results, the composition of FPA were lower than monomer feeding ratio and two samples with different compositions were synthesized for comparison, as listed in Table 1.
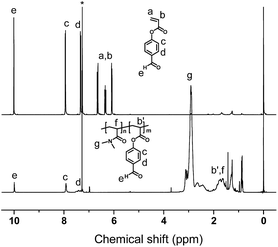 |
| Fig. 1 1H NMR spectra of FPA (top) and P(DMA104-stat-FPA8) in CDCl3 (bottom). | |
Table 1 Copolymers of P(DMA-stat-FPA) with different compositions
Sample |
Polymer |
FPA molar ratioa |
Mn,b (kg mol−1) |
Mw/Mnc |
Tg (°C) |
Calculated from 1H NMR spectroscopy. Evaluated by monomer conversion. Determined by GPC. |
P1 |
P(DMA199-stat-FPA4) |
2% |
21.8 |
1.18 |
119.1 |
P2 |
P(DMA104-stat-FPA8) |
7% |
11.7 |
1.17 |
105.6 |
The structures of FPA and copolymers were also characterized by FT-IR. Two absorbances appeared at 1744 cm−1 and 1700 cm−1 represented carbonyl groups of ester bond and aldehyde group respectively, as shown in Fig. 2 (top). The absorbance at 1600 cm−1 on the spectrum is derived from benzene ring, which proved the structure of FPA. After copolymerization, three absorbance peaks representing carbonyl groups are illustrated at 1757 cm−1, 1700 cm−1 and 1645 cm−1 separately, as shown in Fig. 2 (bottom). The absorbance at 1645 cm−1 is derived from amide group on DMA segment; while the other two peaks are come from FPA. It was also noticed that the absorbance of ester carbonyl group on FPA moved to 1757 cm−1 because the C
C bond was consumed during the copolymerization. Although the size of FPA segment is much bigger than that of DMA, the Tg of the copolymers decreased with increasing FPA composition because of decreased H-bond force, as determined by DSC (Fig. S1†), the Tg values of the copolymer are listed in Table 1.
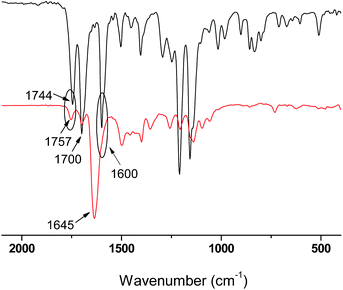 |
| Fig. 2 FT-IR spectra of FPA (top) and P(DMA104-stat-FPA8) (bottom). | |
Preparation of hydrogels from P(DMA-stat-FPA) with a variety of dihydrazine cross-linking
The reversible acylhydrazone bond can be formed automatically in water conditions without any additional catalysis or stimulus,26 which endows the acylhydrazone based self-healable hydrogels with better bio-compatibility and better persistence. On the other hand, the stimulus free self-healing process can be used in more areas without worrying about run away of catalyst. The hydrogels were prepared in deionized water to investigate the gelation reaction and the group ratio of aldehyde to hydrazide was fixed at 1
:
1, the formation of hydrogel was confirmed by tilting the vials.
The hydrogels were prepared by reaction of P(DMA-stat-FPA) with dihydrazide compounds, the dihydrazide with various molecular weights were used to regulate the cross-linking density and composition of the hydrogels, as shown in Scheme 2. It was noted the hydrogel prepared from P(DMA199-stat-FPA4) cross-linked by DTDPH is actually a viscous sol because of its low stiffness (Fig. S2†). The hydrogels prepared from P(DMA104-stat-FPA8) with DTDPH as cross-linkers formed in several minutes without any addition stimulus (Scheme 2). Moreover, the density of cross-linking point can be regulated by increasing the Mn of cross-linker. When the cross-linker was changed into PEO dihydrazide with various molecular weights, all the hydrogels formed within 1 h. The mechanical properties of the hydrogels with different cross-linking densities and composition of gelator can then be examined.
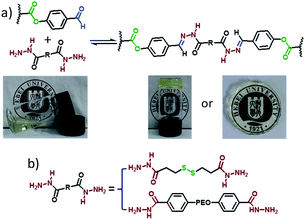 |
| Scheme 2 Schematic illustration for preparation of hydrogel from P(DMA-stat-FPA) with dihydrazide cross-linking (a) and the structure of the dihydrazide cross-linkers (b). (Credit from Hebei University). | |
Rheological properties of self-healable hydrogels
The mechanical properties of the hydrogels were determined by rheology characterization, the storage modulus (G′), loss modulus (G′′) and complex viscosity (η*) dependant on frequency were determined. The P(DMA199-stat-FPA4) cross-linked by DTDPH is actually a viscous sol. With increasing functionality, the hydrogel prepared from P(DMA104-stat-FPA8) and DTDPH showed solid characteristic that the G′ > G′′ in whole frequency range and the G′ is very low, which indicated the low strength of the hydrogel, as shown in Fig. 3a. It is surprising to notice that the G′ increased and then decreased with increasing Mn of the PEO dihydrazide cross-linkers, when the PEO45 dihydrazide (Mn = 2270) and PEO90 dihydrazide (Mn = 4270) were used as cross-linker, the G′ increased gradually rather than decreasing although cross-linking density decreased, as shown in Fig. 3(b and c). When the cross-linker was further changed to PEO227 dihydrazide (Mn = 10
270), the G′ decreased with the decrease in crosslink density (Fig. 3d). This result indicated that the strength of the hydrogel (G′) depends not only on cross-linking density, but also on composition of the gelators and the PEO can increase the strength of the hydrogel. Importantly, all samples showed solid characteristic that with G′ > G′′. This result was confirmed through cross-linking P(DMA199-stat-FPA4) by PEO90 dihydrazide, as expected, hydrogel with increased strength was obtained (Fig. S3†)
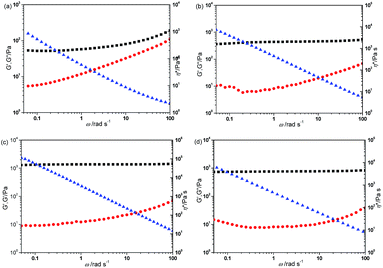 |
| Fig. 3 Rheological experiments of P(DMA104-stat-FPA8) copolymers with various cross-linking agent. The gelator concentrations are 10% and the group ratio of 1 : 1 (5% strain, and 25 °C). (a) DTDPH; (b) PEO45 dihydrazide; (c) PEO90 dihydrazide; (d) PEO227 dihydrazide. (Black: G′; red: G; blue: η*). | |
Self-healing study of the hydrogels with various cross-linking densities
The self-healing properties of hydrogels with various compositions and cross-linking agents were studied, however, the hydrogels prepared form P(DMA104-stat-FPA8) cross-linked by DTDPH with the highest cross-linking density was sticky and soft as characterized by rheology; as a result, the self-healing experiment can not be carried out. When PEO45 dihydrazide was used as cross-linker, although the cross-linking density decreased, the hydrogel was no longer sticky and the self-healing performed perfectly. The self-healing process of this hydrogel is shown in Fig. 4-1, when the hydrogel (Fig. 4-1a) was cut into two halves across the center (Fig. 4-1b) and put back into the original mould with close contact, the hydrogel self-healed via acylhydrazone exchange without any stimulus (Fig. 4-1c) and cannot split under stretching (Fig. 4-1d). Moreover, the self-healing can be repeated with no difference noticed (Fig. S4†). However, with further increasing Mn of cross-linker, the hydrogel cross-linked by PEO90 dihydrazide did not self-heal in 24 h (Fig. 4-2(a–d), and even in 72 h). This result is surprising because the lower cross-linking density should facilitate the movement of the polymer chains. Based on the rheological study, the G′ of this hydrogel is higher than that with PEO45 dihydrazide cross-linking. This result indicated that the reversible reaction depends on stiffness of hydrogel besides cross-linking density, and high stiffness restricted the reversible reaction of the acylhydrazone along the cut line. When the molecular weight of the cross-linker further increased, the hydrogel with PEO227 dihydrazide cross-linking become self-healable again, as shown in Fig. 4-3(a–d).
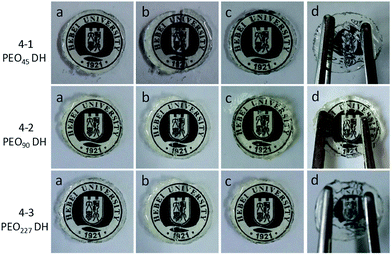 |
| Fig. 4 Self-healing study of the hydrogels prepared from P(DMA104-stat-FPA8) with various PEO dihydrazide (PEO DH) cross-linking. (Credit from Hebei University). | |
Morphology of the hydrogels
The cross-linking density of the hydrogels was compared by SEM. The hydrogels were freeze dried directly to preserve the original morphology. The SEM images of the hydrogels are shown in Fig. 5. As shown in Fig. 5a, although the stiffness of the hydrogel with DTDPH cross-linking was comparatively low, the regular micro pores with uniform pore size of 3–5 μm are illustrated in the picture. When the cross-linker was changed to PEO dihydrazide, the pore size increased with increasing Mn of the cross-linker and the pore walls collapsed, as shown in Fig. 5b and c. The possible reason for this result is the morphology changed after fracture since the observation temperature was much higher than Tg of PEO and the PEO ratio in the hydrogels were pretty high.
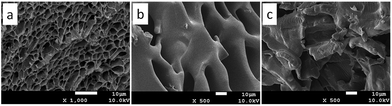 |
| Fig. 5 SEM photographs of hydrogels after freeze dried from P(DAM104-stat-FPA8) cross-linked by DTDPH (a), PEO45 dihydrazide (b) and PEO90 dihydrazide (c). | |
Gel–sol–gel transition and degradation of the hydrogels in mild base
The acylhydrazone bond can go reversible coupling and de-coupling reaction under pH triggers, so the hydrogel can go gel–sol transition by regulating the pH of the water. When CF3COOH was added into the hydrogel, all hydrogels were dissolved into liquid fluid overnight, the same result was obtained with addition of 5 M HCl to change the pH to 3. The pH triggered gel–sol transition of hydrogel with PEO45 dihydrazide cross-linking is shown in Fig. 6. When the pH of the solution was changed to 6.5 by addition of triethylamine, the hydrogel was obtained again, as shown in Fig. 6(a and b). Above result confirmed that the phenolic ester is pretty stable under mild acid at room temperature. With base labile phenolic ester bond on the copolymer structure, the hydrogel also showed gel–sol transition in mild base, when Na2CO3 solution was added into the self-healable hydrogel to 2% concentration, the hydrogel degraded gradually within 24 h and the resultant solution became brown, as shown in Fig. 6c. The NaHCO3 can also degrade the hydrogel, but because of low reactivity of NaHCO3, the degradation of the hydrogel need pretty long time scale. The NaHCO3 solution was absorbed and the hydrogel turned brown after 3 days, and the hydrogel degraded gradually into dark brown viscous liquid in two weeks, as shown in Fig. 6 (bottom), this result also proved the phenolic ester bond in conjugated structure can still be cleaved by NaHCO3, but the degradation rate is very slow. Further experiments showed that excess triethylamine can also degrade the hydrogels (Fig. S5†). It is easy to understand the hydrogel cannot be obtained by regulating the pH of the solution to neutral since the phenolic ester connection was cleaved, as shown in Scheme 3. Other hydrogels also showed the same result because of same cross-linking structure.
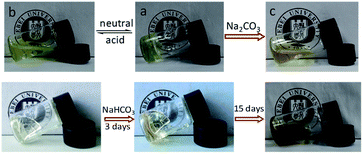 |
| Fig. 6 pH triggered gel–sol–gel transition and degradation in Na2CO3 of the self-healable hydrogel prepared from P(DMA104-stat-FPA8) with PEO45 dihydrazide cross-linking (top) and degradation process of the hydrogel in NaHCO3 (bottom). | |
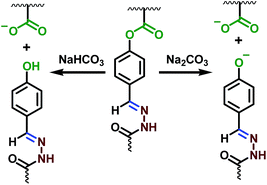 |
| Scheme 3 Schematic illustration for degradation of hydrogel in NaHCO3 and Na2CO3. | |
The degradation of phenolic ester bond was tracked and confirmed by UV characterizations. The UV spectra of the P(DMA104-stat-FPA8), its degradation products in NaHCO3 and Na2CO3 along with degraded self-healable hydrogel with PEO45 dihydrazide cross-linking are shown in Fig. 7. The copolymer showed the absorbance at 253 nm, as shown in Fig. 7 (black line). After stirred in Na2CO3 for 24 h, the peak at 253 nm disappeared completely because the phenolic ester bond was cleaved (Fig. 7 blue line). However, the phenolic ester bond was only cleaved partially in NaHCO3 in 24 h and the peak at 253 nm was still exist (Fig. 7 red line). The self-healable hydrogel can be degraded in NaHCO3 and Na2CO3, but the UV spectrum showed the phenolic ester bond in the hydrogel only degraded partially in Na2CO3 in 24 h without stirring and some absorbance was still existed at 253 nm, as shown in Fig. 7 (purple line). Moreover, the absorbance of the degradation product moved to 344 nm because the aldehyde group had reacted with hydrazide and changed the conjugated structure.
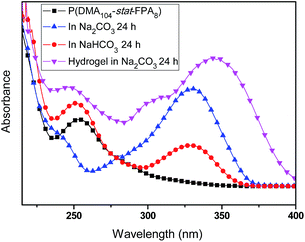 |
| Fig. 7 UV spectra of P(DMA104-stat-FPA8) and its degradation products in 2% NaHCO3, Na2CO3 and degraded hydrogel with PEO45 dihydrazide cross-linking for 24 h in Na2CO3. | |
As the disulfide bond can be cleaved by reduction, the hydrogel with DTDPH cross-linking also go redox triggered gel–sol–gel transition besides pH triggered gel–sol–gel transition, as shown in Fig. 8(a–c). When 2 equivalents of DTT was added into the hydrogel with DTDPH cross-linking, the hydrogel was degraded into a viscous solution in 24 h because the disulfide group was reduced into thiol group. When same equivalent of H2O2 was added to oxidize the thiol group, hydrogel was re-obtained since the disulfide group was formed again. With phenolic ester bond connection, this hydrogel was also degraded into a dark brown liquid by NaHCO3, as shown in Fig. 8(d). However, although the hydrogel cross-linked by DTDPH showed multi-triggered gel–sol–gel transitions and NaHCO3 degradation, the low strength of this sample could limit its application in wide areas, multi-responsive self-healable hydrogel with improved strength and degradability in NaHCO3 is under intensive study.
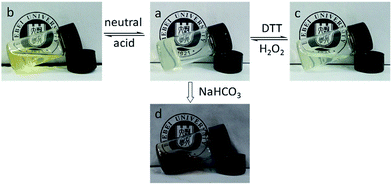 |
| Fig. 8 Gel–sol–gel transition and degradation of DTDPH cross-linked hydrogel. | |
It was reported that the self-healable hydrogels can be cleaved by group exchange with excess of mono-functional compound22 and cross-linkers. The gel–sol–gel transition regulated by excess of dihydrazide compounds was investigated. When 3 equivalents excess of cross-linker was added into the hydrogel, the hydrogel was dissolved slowly in 24 h; moreover, when same equivalent of P(DMA104-stat-FPA8) was added to regulate the group ratio to 1
:
1, hydrogel was obtained again. The mechanism of this reaction and the photograph for this process are shown in Fig. 9. More importantly, although the hydrogel with PEO90 dihydrazide cross-linking did not self-heal within 72 h, this hydrogel still showed group ratio triggered gel–sol–gel transition (Fig. S6†) and other hydrogels showed the same result. Above result indicated that although the hydrogel with PEO90 dihydrazide cross-linking did not self-heal in 24 h, the reversible characteristic of acylhydrazone was still exist. But the acylhydrazone exchange rate was comparatively low along the cut line because high stiffness of the hydrogel restricted the movement functional group and polymer chain.
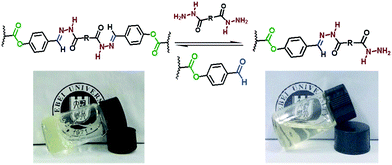 |
| Fig. 9 Mechanism for group ratio triggered gel–sol–gel transition and photograph of the hydrogel prepared from P(DMA104-stat-FPA8) and PEO45 dihydrazide. | |
As mentioned above, the hydrogels can also be degraded by triethylamine. It was also noticed the hydrogel cannot be obtained again when the concentration of HCl was higher than 0.1 mol L−1 because the ester bond can also be cleaved by acid. On the other hand, when excess of H2O2 was added to oxidize thiol group to disulfide group, the re-obtained hydrogel would degrade again. But the group ratio triggered gel–sol–gel transition never fail because no any possible side reaction involved in this process. Moreover, this process did not change any valency of the gelators nor produce any by-product. So this property provide a effective method to reuse and recycle of the self-healable hydrogel.
Thermal stability of the copolymer and the hydrogels
The phenolic ester bond are less stable than other chemical bonds in the polymer chain, this property can be determined by TGA. The TGA curves of the copolymer and the hydrogels are shown in Fig. 10. The P(DMA104-stat-FPA8) began to loss some weight at 215 °C because the phenolic ester bond was cleaved and the 4-hydroxybenzaldehyde was evaporated, as shown Fig. 10 (black line), while the decomposition temperature of the polymer chain was much higher (>360 °C). After cross-linked by DTDPH, the decomposition temperature increased to 282 °C (red line in Fig. 10). Although the decomposition temperature of the phenolic ester bond should not change obviously, but the aldehyde has reacted with hydrazide group and can only evaporate with the cross-linker. On the other hand, there is no weight loss noticed at the decomposition of copolymer, indicated that there was no free aldehyde group left after cross-linking, proved the equilibrium constant of the coupling reaction is pretty high. When the PEO dihydrazide was used as cross-linker, no weight loss was noticed until 332 °C (Fig. 10 blue and purple line). This result is reasonable because there is no small molecules evaporate even if the phenolic ester bond was cleaved. There is only one weight loss process on the TGA curve, indicated the decomposition temperature of PEO and polymer chains are at the same temperature range.
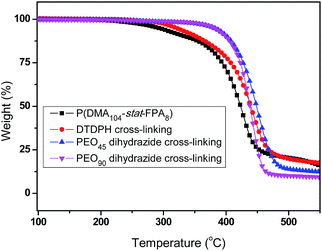 |
| Fig. 10 TGA curves of the copolymer and the hydrogels with various cross-linking agents. | |
Based on above studies, self-healable hydrogels with multi-triggered gel–sol–gel transition and basic degradation is designed, the degradation of the self-healable hydrogel is triggered by extremely mild base of NaHCO3. This kind of smart hydrogel could have great potential application in areas related to bioscience and biotechnology with enhanced persistence and can be degraded under mild conditions to avoid possible side effects. The application of this kind of self-healable hydrogel in drug loading and delivery is under investigation.
Conclusion
Self-healable hydrogels were prepared from P(DMA-stat-FPA) cross-linked by dihydrazide compounds. With aldehyde group and phenolic ester bond in the P(DMA-stat-FPA), self-healable hydrogel with NaHCO3 degradability was prepared without any additional stimulus in purified water. The self-healable hydrogel showed gel–sol–gel transition based on reversible characteristics of acylhydrazone bond. The redox triggered gel–sol–gel transition can be realized with disulfide containing cross-linker. However, the PEO dihydrazide tends to increase the strength of the hydrogel although cross-linking density decreased. The hydrogel can be cleaved into solution with excess of cross-linker, which is very useful for recycle of the self-healable hydrogel. Importantly, the self-healable hydrogel can be degraded by mild base of NaHCO3 through cleavage of the base labile phenolic ester bond.
Acknowledgements
This research was kindly supported by Natural Science Foundation of Hebei (B2015201085); the Department of Education, Hebei Province (No. QN2017014); the Returned Overseas Chinese Scholars, State Education Ministry and Challenge Cup Program of Hebei University.
References
- J. Ling, M. Z. Rong and M. Q. Zhang, Polymer, 2012, 53, 2691–2698 CrossRef CAS.
- C.-M. Chung, Y.-S. Roh, S.-Y. Cho and J.-G. Kim, Chem. Mater., 2004, 16, 3982–3984 CrossRef CAS.
- B. Ghosh and M. W. Urban, Science, 2009, 323, 1458–1460 CrossRef CAS PubMed.
- N. Kuhl, S. Bode, R. K. Bose, J. Vitz, A. Seifert, S. Hoeppener, S. J. Garcia, S. Spange, S. van der Zwaag, M. D. Hager and U. S. Schubert, Adv. Funct. Mater., 2015, 25, 3295–3301 CrossRef CAS.
- H. Ying, Y. Zhang and J. Cheng, Nat. Commun., 2014, 5, 3218 Search PubMed.
- L. Hu, X. Cheng and A. Zhang, J. Mater. Sci., 2015, 50, 2239–2246 CrossRef CAS.
- M. Vatankhah-Varnoosfaderani, S. Hashmi, A. GhavamiNejad and F. J. Stadler, Polym. Chem., 2014, 5, 512–523 RSC.
- M. Zhang, D. Xu, X. Yan, J. Chen, S. Dong, B. Zheng and F. Huang, Angew. Chem., Int. Ed., 2012, 124, 7117–7121 CrossRef.
- P. Cordier, F. Tournilhac, C. Soulie-Ziakovic and L. Leibler, Nature, 2008, 451, 977–980 CrossRef CAS PubMed.
- T. Ueki, Y. Nakamura, R. Usui, Y. Kitazawa, S. So, T. P. Lodge and M. Watanabe, Angew. Chem., Int. Ed., 2015, 54, 3018–3022 CrossRef CAS PubMed.
- T. Ueki, R. Usui, Y. Kitazawa, T. P. Lodge and M. Watanabe, Macromolecules, 2015, 48, 5928–5933 CrossRef CAS.
- A. Phadke, C. Zhang, B. Arman, C.-C. Hsu, R. A. Mashelkar, A. K. Lele, M. J. Tauber, G. Arya and S. Varghese, Proc. Natl. Acad. Sci. U. S. A., 2012, 109, 4383–4388 CrossRef CAS PubMed.
- J. Zhan, M. Zhang, M. Zhou, B. Liu, D. Chen, Y. Liu, Q. Chen, H. Qiu and S. Yin, Macromol. Rapid Commun., 2014, 35, 1424–1429 CrossRef CAS PubMed.
- G. Li, J. Wu, B. Wang, S. Yan, K. Zhang, J. Ding and J. Yin, Biomacromolecules, 2015, 16, 3508–3518 CrossRef CAS PubMed.
- D. L. Taylor and M. in het Panhuis, Adv. Mater., 2016, 28, 9060–9093 CrossRef CAS PubMed.
- X. Chen, M. A. Dam, K. Ono, A. Mal, H. Shen, S. R. Nutt, K. Sheran and F. Wudl, Science, 2002, 295, 1698–1702 CrossRef CAS PubMed.
- Y. Amamoto, J. Kamada, H. Otsuka, A. Takahara and K. Matyjaszewski, Angew. Chem., Int. Ed., 2011, 50, 1660–1663 CrossRef CAS PubMed.
- K. Imato, M. Nishihara, T. Kanehara, Y. Amamoto, A. Takahara and H. Otsuka, Angew. Chem., Int. Ed., 2012, 51, 1138–1142 CrossRef CAS PubMed.
- H. Li, J. Bai, Z. Shi and J. Yin, Polymer, 2016, 85, 106–113 CrossRef CAS.
- B. Maiti, B. Ruidas and P. De, React. Funct. Polym., 2015, 93, 148–155 CrossRef CAS.
- Y. Zhang, L. Tao, S. Li and Y. Wei, Biomacromolecules, 2011, 12, 2894–2901 CrossRef CAS PubMed.
- S. Mukherjee, M. R. Hill and B. S. Sumerlin, Soft Matter, 2015, 11, 6152–6161 RSC.
- G. Deng, C. Tang, F. Li, H. Jiang and Y. Chen, Macromolecules, 2010, 43, 1191–1194 CrossRef CAS.
- G. H. Deng, F. Y. Li, H. X. Yu, F. Y. Liu, C. Y. Liu, W. X. Sun, H. F. Jiang and Y. M. Chen, ACS Macro Lett., 2012, 1, 275–279 CrossRef CAS.
- F. Yu, X. Cao, J. Du, G. Wang and X. Chen, ACS Appl. Mater. Interfaces, 2015, 7, 24023–24031 CAS.
- R. Chang, X. Wang, X. Li, H. An and J. Qin, ACS Appl. Mater. Interfaces, 2016, 8, 25544–25551 CAS.
- S. Y. An, S. M. Noh, J. H. Nam and J. K. Oh, Macromol. Rapid Commun., 2015, 36, 1255–1260 CrossRef CAS PubMed.
- J. J. Cash, T. Kubo, A. P. Bapat and B. S. Sumerlin, Macromolecules, 2015, 48, 2098–2106 CrossRef CAS.
- O. R. Cromwell, J. Chung and Z. Guan, J. Am. Chem. Soc., 2015, 137, 6492–6495 CrossRef CAS PubMed.
- J. Kamada, K. Koynov, C. Corten, A. Juhari, J. A. Yoon, M. W. Urban, A. C. Balazs and K. Matyjaszewski, Macromolecules, 2010, 43, 4133–4139 CrossRef CAS.
- Y. Shi, X. F. Wang, R. W. Graff, W. A. Phillip and H. F. Gao, J. Polym. Sci., Part A: Polym. Chem., 2015, 53, 239–248 CrossRef CAS.
- R. Chang and J. Qin, Macromol. Chem. Phys., 2014, 215, 1908–1914 CrossRef CAS.
- A. Chao, I. Negulescu and D. Zhang, Macromolecules, 2016, 49, 6277–6284 CrossRef CAS.
- R. Chang, N. Li, J. Qin and H. Wang, Polymer, 2015, 60, 62–68 CrossRef CAS.
- R. Chang, H. An, X. Li, R. Zhou, J. Qin, Y. Tian and K. Deng, Polym. Chem., 2017, 8, 1263–1271 RSC.
- J. T. Lai, D. Filla and R. Shea, Macromolecules, 2002, 35, 6754–6756 CrossRef CAS.
- B. Peng, Y. Liu, Y. Shi, Z. Li and Y. Chen, Soft Matter, 2012, 8, 12002–12008 RSC.
- D. X. Wu, X. H. Song, T. Tang and H. Y. Zhao, J. Polym. Sci., Part A: Polym. Chem., 2010, 48, 443–453 CrossRef CAS.
- Y. Shi, W. Zhu and Y. Chen, Macromolecules, 2013, 46, 2391–2398 CrossRef CAS.
Footnote |
† Electronic supplementary information (ESI) available: DSC curves of copolymers, photographs of hydrogels with different component and gel–sol transitions under different conditions. See DOI: 10.1039/c7ra05854c |
|
This journal is © The Royal Society of Chemistry 2017 |