DOI:
10.1039/C7RA04813K
(Paper)
RSC Adv., 2017,
7, 37112-37121
Efficient decolorization of citric acid fermentation broth using carbon materials prepared from phosphoric acid activation of hydrothermally treated corncob
Received
29th April 2017
, Accepted 23rd June 2017
First published on 26th July 2017
Abstract
Conventional activated carbon used in decolorization of citric acid fermentation broth has the disadvantage of high citric acid loss. In this study, a novel biomass-based carbon material, namely HBCM, was prepared via H3PO4 activation of hydrothermally treated corncob. The material was fully characterized by SEM, BET, TG, FTIR, XPS and pHpzc. The results showed that the material's SBET was as high as 1720 m2 g−1 and several weak-acid functional groups existed on its surface, which contributed to efficient decolorization with low citric acid loss. By adjusting the solution pH value to around 7, nearly no citric acid was lost. Furthermore, the adsorption behavior of pigments on HBCM was systematically investigated under optimized pH. The results indicated that the adsorption was spontaneous and endothermic. Intra-particle diffusion was the rate-limiting step. By comparing FTIR data before and after adsorption, it was found that oxygen-containing functional groups on the HBCM surface participated in pigment adsorption. Overall, the tailor-made HBCM performed excellently with a 99% decolorization ratio and nearly no citric acid loss under optimum operating conditions. It could be a potential adsorbent in the removal of pigments from citric acid fermentation broth.
Introduction
Hydrothermal carbonization (HTC) is a thermochemical conversion technique in the presence of water, which involves a series of hydrolysis, dehydration, and decarboxylation processes.1 Compared with conventional biomass conversion techniques such as thermal pyrolysis, HTC is carried out at relatively low temperatures (160–300 °C) and produces less greenhouse emissions, and its raw materials do not require any pretreatment, all of which lead to advantages such as high conversion efficiency, convenient operating condition, more environment friendly and lower energy consumption.2–5 As a novel carbonaceous material produced from the HTC of biomass, hydrochar possesses several unique characteristics such as high density of oxygenated functional groups (OFGs) and low degree of condensation.6 To date, considerable research about the hydrochar applications has been conducted in terms of adsorption, bio imaging, catalysis, activated carbon synthesis, etc.7–11. Particularly, major attention has been focused on adsorption applications using hydrochar as an adsorbent due to its unique characteristics. For instance, Li et al.12 studied hydrochar produced via HTC of corncob residues to adsorb phenol and p-nitrophenol. Liu et al.7 explored its application as the adsorbent in the removal of lead from wastewater. Zhang et al.13 used char produced from pinewood hydrothermal carbonization as the adsorbent for copper removal. However, as an adsorbent, hydrochar has low specific surface area (10–40 m2 g−1).14 Consequently, several post-treated techniques such as thermal/physical activation and chemical activation need to be employed to obtain hydrochar-based carbon materials (HBCM) with higher specific surface area that can be used as adsorbents. Liu et al. used rice husk and pinewood as precursors to synthesize HBCMs via CO2 physical activation. The specific surface area was increased to 569 and 446 m2 g−1.15 Wang et al. produced HBCM with specific surface area of 2610 m2 g−1 by means of H3PO4 activation.16 The pore texture and specific surface area were improved significantly using post-treatment techniques. These carbon materials could be efficiently applied in adsorption. However, to date, the use of HBCM as an adsorbent to remove pigments from fermentation broth has not been reported.
Citric acid, as an important organic acid, is widely used in food, beverage, chemical and pharmaceutical fields. The worldwide annual demand of citric acid has reached several millions tonnes and nearly half of this volume is supplied by China.17 Currently, citric acid is mainly produced via fermentation of Aspergillus niger.18 In addition to citric acid, there exist several unidentified components, i.e., pigments, in the fermentation broth, which affect the quality of the final citric acid product and need to be removed. It is well known that pigments in the fermentation broth are mainly derived from the fermentation medium, Maillard reactions and the fermentation process generated by microorganisms, and they are a type of a complex mixture. At present, decolorization of citric acid fermentation broth is achieved by means of activated carbon adsorption. A relatively high decolorization ratio can be achieved. However, the selectivity between citric acid and pigments is low and the use of activated carbon is not eco-friendly due to its production process and difficult regeneration.
Recently, several alternative methods, i.e., organic solvent partitioning, sevage reagent use, and membrane and column chromatography separation, have been proposed, in which adsorption attracts the most attention due to its high decolorization efficiency, high selectivity and easy regeneration. For instance, Ana et al. used yellow passion fruit peel derived biochar as an adsorbent to remove methylene blue. Wen et al. studied the adsorption–desorption behavior of magnetic amine/Fe3O4 functionalized biopolymer resin for anionic dye adsorption from wastewater. However, the above-mentioned studies all focused on removal of known pigments. Removal of unknown pigments, particularly the adsorption of unknown complex mixtures, i.e., pigments existing in citric acid fermentation broth, has been seldom reported.
Therefore, in this study, we investigated the decolorization of citric acid fermentation broth using tailor-made HBCM via corncob hydrothermal carbonization coupled with H3PO4 activation. The HBCM was fully characterized by elemental analysis, scanning electron microscopy (SEM), N2 adsorption/desorption isotherms (BET), Fourier transform infrared spectroscopy (FTIR), X-ray photoelectron spectroscopy and pHpzc. Taking decolorization efficiency and citric acid loss into consideration, the optimal operating conditions (adsorbent dosage and pH) were selected, under which the adsorption equilibrium, thermodynamics and kinetics of pigments on the HBCM were systematically investigated. Furthermore, adsorption mechanism of the pigments on tailor-made HBCM was explored by comparing FTIR results before and after adsorption. Overall, the synthesis procedure of tailor-made HBCM was simple and eco-friendly. The obtained adsorbent had good adsorption selectivity to pigments and almost no adsorption to citric acid under optimal operating conditions. It could be a potential adsorbent for decolorization of citric acid fermentation broth.
Materials and methods
Chemicals and equipment
All chemicals NaOH (≥99%), NaCl (≥99%), HCl (37%), and H3PO4 (85%) used in this study were of analytical grade purchased from Sigma Aldrich Co. The citric acid fermentation broth was self-made in laboratory. The equipment used in the study included a drying oven (GZX-9240), Spectrumlab 752S, oscillation incubator (MQD-A2), tube furnace (QTF-1200X-80) and high-performance liquid chromatograph (AGILENT 1200) etc.
Preparation of adsorbent
The adsorbent used in the study was manufactured by a two-step process, namely hydrothermal carbonization and H3PO4 activation. During the hydrothermal carbonization step, 3.5 g of raw corncob and 35 mL of de-ionized water were placed into a vertical stainless steel reactor. The reactor was heated to 473 K and kept for 24 h. After the reaction, the solid products were washed by de-ionized water, dried at 373 K for 12 h, and ground with a 0.25 mm sieve. Hydrochar was then obtained. H3PO4 activation was performed as follows: the obtained hydrochar was soaked in 30% (mass fraction) phosphate solutions of ratio of 1
:
2 (biochar mass
:
phosphate solution volume) and impregnated and at room temperature for 10 min. For thermal activation, the carbon material was loaded in a horizontal stainless reactor and heated in a tube furnace at the rate of 10°C min−1 from room temperature to 723 K and maintained at this temperature for 1 h under N2 flow. After activation, the resulting materials were ground and washed using hot de-ionized water to the activation agent residue and byproducts formed during the process. Finally, the self-produced hydrochar and HBCM were stored in anhydrous conditions for further analysis.
Preparation of citric acid fermentation broth
The citric acid fermentation broth was obtained by Aspergillus niger's submerged fermentation. The detailed preparation process is provided in ref. 19. Then, the ultimately obtained fermentation broth was pre-treated by centrifugal separation and refrigerated before further use during the following adsorption experiment.
Determination of decolorization ratio and citric acid recovery ratio
It is impossible to determine the exact concentrations of pigments existing as complex mixtures in the citric acid fermentation broth whose compositions are still unknown. In our previous experiments, we found that the absorbance of citric acid at 260 nm is nearly zero and the pigment concentration is proportionate to the absorbance at 260 nm. Therefore, OD260 (absorbance of sample solution at 260 nm on a spectrophotometer) was selected to represent pigment concentration. Consequently, the decolorization ratio is defined by the following equation: |
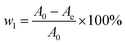 | (1) |
where w1 is the decoloration ratio and A0 and Ae are the measured absorbance of the samples at 260 nm before and after adsorption on HBCM, respectively.
The concentration of citric acid was measured through the reflective index detector of the high-performance liquid chromatography system. Then, through the standard curve of citric acid, the real concentration of citric acid was obtained. The following equation was used to quantify the citric acid loss ratio during the batch experiments:
|
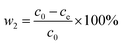 | (2) |
where
w2 is the adsorbed ratio of citric acid and
c0 and
ce are the concentration of citric acid initially and at adsorption equilibrium, respectively.
Batch adsorption studies
For batch adsorption studies, a certain volume of citric acid fermentation broth (10 mL) was taken in 50 mL conical flasks containing corresponding quantity of the self-made adsorbent. Then, hermetically sealed flasks containing these mixtures were placed on a shaker and stirred at a constant shaking rate of 100 rpm for a predetermined temperature and time. After adsorption equilibrium, the suspensions were filtered using membrane filters of 0.22 μm pore size. Thereafter, the remaining contents of pigments and citric acid were determined by a spectrophotometer (Spectrumlab 752S) at a wavelength of 260 nm and refractive index detector (RID) of the high-performance liquid chromatograph using a calibration curve, respectively.
The effect of operating conditions (adsorbent dosage, solution pH and temperature) on pigment adsorption process was analyzed. The adsorbent mass added into solution was in the range of 0.1–0.4 g. The solution pH was selected between 1–7 adjusted by 0.1 mol L−1 NaOH and HCl. The surrounding temperature influences were evaluated to be between 298 and 318 K.
In order to investigate the adsorption kinetics of the pigments on the adsorbents, the 50 mL conical flasks containing fixed adsorbent mass and solution volume were placed on a shaker and stirred for times ranging from 0.5 to 240 min under optimal conditions obtained via the abovementioned experiments. In addition, equilibrium adsorption isotherm experiments were performed using the conventional conical flask shaking method at three different temperatures: 298, 308 and 318 K. Furthermore, all adsorption experiments were carried out in triplicate to confirm the reproducibility of the results, and the average of these measurements was used to express each assessment.
Characterization of the adsorbents
Hydrochar and HBCM were characterized by elemental analysis, SEM, FTIR, N2 adsorption/desorption and pHpzc. The proximate content was obtained using a Perkin Elmer CHN element analyzer (Arvato EA 300; Italy), and the oxygen content was calculated by the balance. The surface images of hydrochar and HBCM were obtained via SEM. The experiments of N2 adsorption/desorption at 77 K were performed by a surface area and porosity analyzer (ASAP 2460, USA) to get the specific surface and pore size distribution. Briefly, the surface area was calculated by the Brunauer–Emmett–Teller (BET) model from relative pressures (P/P0) in the range of 0.01–0.1 with a correlation coefficient high than 0.9999;20 the total pore volume was obtained by single-point adsorption of N2 at a high relative pressure (0.99); micropore area and micropore volume were determined by t-plot method; mesoporous area and mesoporous volume were calculated using the BJH model;21 pore size distribution was auto-generated by applying density functional theory (DFT) to the N2 adsorption isotherms using the software supplied by ASAP 2460. Thermogravimetric analyses (TGA) were performed on HBCM. A TGA Q500 instrument was used for TGA. The mass was recorded at an increased temperature under a flow of N2. The functional groups existing on the surface were analyzed through a Fourier transform infrared spectrometer. The specific process was shown as follows: the samples were mixed with KBr and pressed into pellets; then, the FTIR spectra were recorded at a range of 400–4000 cm−1 at a resolution of 4 cm−1 and acquisition rate of 20 scans min−1. Furthermore, X-ray photoelectron spectroscopy (XPS) was used to quantitatively analyze specific bonding styles between carbon and oxygen, as well as the corresponding ratios. In addition, the surface charges of the adsorbent under various pH values were obtained by exploring the pH at the point of zero charge (pHpzc) of the sample, which was carried out by solid addition method.22 Briefly, 50 mL, 0.1 mol L−1 NaCl was placed in a series of 100 mL conical flasks, in which the solution pH was adjusted from 2 to 12 by adding either 0.1 mol L−1 HCl or NaOH. Then, 0.2 g of each sample was added and agitated for 24 h at 308 K; the final pH was noted. The pHpzc is the intersection point of pHinitial − pHfinal versus pHinitial.
Results and discussion
Characterization of the adsorbent
Morphology and structural properties of hydrochar and HBCM samples. The SEM images of the tailor-made hydrochar and HBCM samples are shown in Fig. 1. Both the carbon materials have irregular surfaces. Hydrochar consists of some microspheres and irregular structures, which originated from the cellulose and lignin components existing in the corncob can't be decomposed during the hydrothermal carboniztion process and their structure remain the origin state. On further activation treatment, the morphology of HBCM is relatively stable and consists of considerable number of microspheres, which is beneficial for pigment adsorption in the following study.
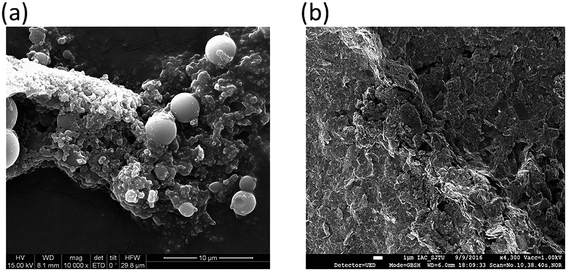 |
| Fig. 1 SEM images for (a) hydrochar and (b) HBCM. | |
The porous textural properties of hydrochar and HBCM samples are analyzed by N2 sorption measurements at 77.15 K. The results show that specific surface area of the samples increases from 7.20 m2 g−1 to 1720 m2 g−1 by activation treatment. The low specific surface area of hydrochar can be attributed to dehydration, polymerization and condensation reaction in the hydrothermal process, hindering pore formation. N2 adsorption–desorption isotherms of HBCM are shown in Fig. 2(a), which is in line with type I isotherm, suggesting that the material possesses a developed micropore structure. The pore size distribution figure also indicates that pores are mostly located in the microporous region, nearly 90% of the total surface area of HBCM is occupied by micropores, whereas there are nearly no pores in hydrochar.
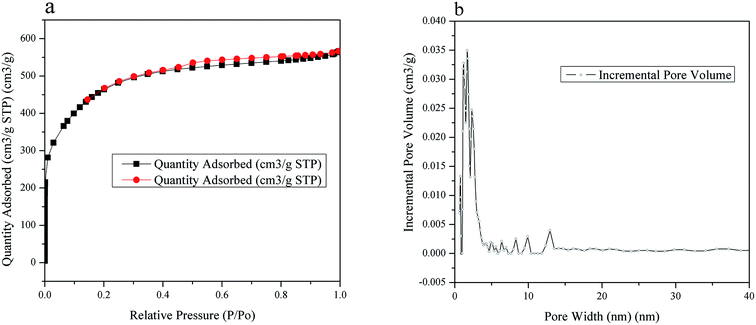 |
| Fig. 2 (a) N2 adsorption/desorption isotherms at 77 K of HBCM. (b) Pore size distribution of HBCM. | |
Proximate and elemental analysis. According to the data of proximate and elemental analysis in Table 1, the two carbon materials are dominantly composed of carbon, oxygen, and hydrogen elements. After H3PO4 activation, the carbon content increases and oxygen content decreases. Moreover, the O/C, (O + N)/C and H/C atomic ratios are calculated to evaluate the polarity and aromaticity. The results indicate that H3PO4 activation improves aromatic and hydrophobic ratios of the carbon materials due to the higher carbonization extent and loss of polar functional groups by activation. This would facilitate the adsorption of non-polar substances in citric acid fermentation broth.
Table 1 Chemical composition (wt%) of hydrochar and HBCM
Type |
C (%) |
O (%) |
H (%) |
N (%) |
H/C |
O/C |
(O + N)/C |
Before activation |
60.84 |
32.78 |
5.35 |
0.15 |
1.04 |
0.40 |
0.407 |
After activation |
89.83 |
7.7 |
2.22 |
0 |
0.29 |
0.08 |
0.086 |
TG analysis. Fig. 3 shows the TG results of HBCM. It can be observed that its weight nearly remains constant at low temperature (≤450 °C), while a significant weight loss occurred in a temperature range of 450–700 °C; finally, a small quantity remained. This phenomenon can be described as follows: during the previous activation step, the lignin-like compound or thermally produced carbonized/aromatic compound is thermally degraded24 and H3PO4 combined with organic species to form phosphate and polyphosphate bridges that connect and cross-link with biopolymer fragments25 at 450 °C. When the temperature exceeds 450 °C, these formed compounds start degrading, as only a small mass of HBCM remains at temperatures >700 °C.
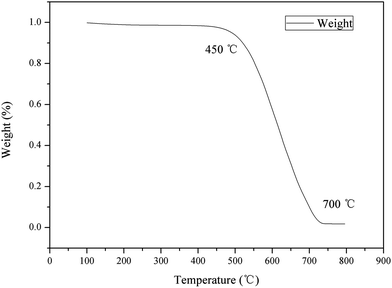 |
| Fig. 3 TGA of HBCM. | |
FTIR analysis. The FTIR spectra of hydrochar and HBCM samples are shown in Fig. 4, which are consistent with the elemental analysis results. For HBCM, a broad absorption peak around 3420 cm−1 indicates the bonded hydroxyl groups, which originate from cellulose and lignin. The peaks observed at 2917 cm−1 are assigned to the stretch vibration and bending vibration of C–H bonds in methylene group.23 The peaks located at 1640 and 1540 cm−1 are characteristics of C
O axial deformation (aldehyde, lactone, ketone and carboxyl groups) and oxygen-containing groups such as highly conjugated C
O or C–O stretching, respectively.26 The peaks at 1613 and 1701 cm−1 indicate that the HBCM adsorbent is composed mainly of aromatic cores derived from the lignin fraction in the hydrothermal treatment process. The peaks associated with the stretch vibration in aromatic rings are verified at 1603 and 1511 cm−1, while peaks due to deformations related to C–H and C–O bonds are observed from 1085 to 1040 cm−1. The peak at 1248 cm−1 may be from the stretch vibration of C–O in phenols. In addition, comparing with FTIR of the hydrochar (Fig. 4), the intensity of 3420 cm−1 and 2917 cm−1 peaks sharply decreases and peaks representing aromatic cores are strengthened. All changes are attributed to the higher carbonization extent and loss of oxygen-containing groups. The activation procedure further strengthens the hydrophobic properties of the HBCM adsorbent.
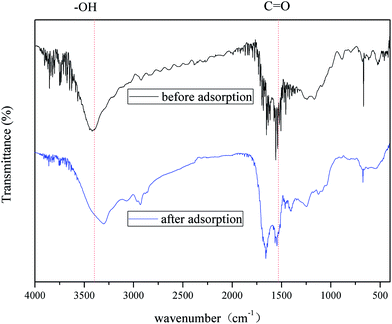 |
| Fig. 4 FTIR spectroscopy of hydrochar and HBCM before adsorption and HBCM after adsorption. | |
XPS analysis. X-ray photoelectron spectroscopy (XPS) is used to investigate surface polar/oxygen functional groups on the hydrochar and HBCM samples, and the data are shown in Fig. 5. For hydrochar, the C1S spectra has been split into four signals at 284.8, 285.7, 287.0, and 288.5 eV, which stand for C–C/C–H, C–O–H/C–O–C, C
O, and O
C–O, respectively. Different from hydrochar, the C1S spectra of HBCM are split into three signals at 284.8, 285.7, and 288.5 eV, which stand for C–C/C–H, C–O–H/C–O–C, and O
C–O, respectively. As shown in Table 2, carbonization extent is strengthened and a large amount of acid groups, namely carboxyl groups, are introduced on the surface of HBCM during phosphoric acid activation.
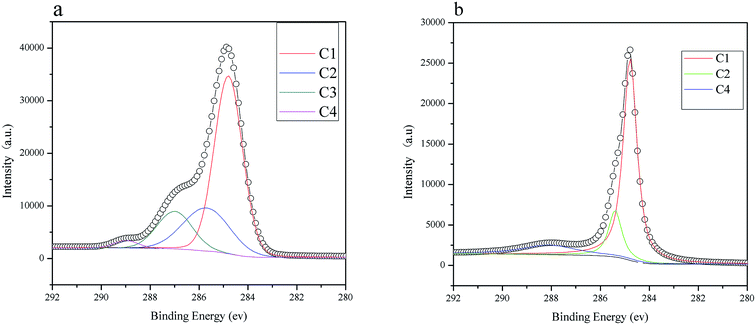 |
| Fig. 5 XPS of (a) hydrochar and (b) HBCM. | |
Table 2 Experimental C1(s), binding energy (eV) and chemical state assignment for hydrochar and HDCM
Sample |
C1 |
C2 |
C3 |
C4 |
Hydrochar |
284.8/59.70 |
285.7/23.03 |
287.0/15.22 |
288.5/2.04 |
HBCM |
284.8/72.25 |
285.7/16.83 |
× |
10.92 |
Determination of pHpzc. The point of zero charge (pHpzc) is defined as the pH value at which charge on material surface is equal to zero. Thus, for HBCM, the surface is positively charged at pH < pHpzc and negatively charged at pH > pHpzc. In the present study, pHzpc is obtained by the difference between the initial and final pH values versus initial pH values. As shown in Fig. 6, the pHpzc of HBCM is around 3, which is in accordance with the results obtained from FTIR.
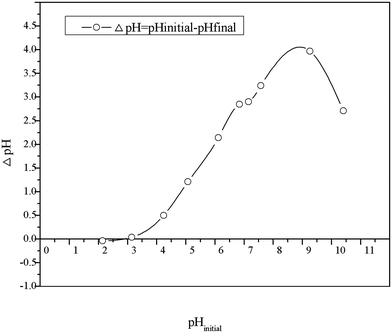 |
| Fig. 6 Point of zero charge of HBCM. | |
Adsorption studies
Effect of adsorbent dosage. The effect of HBCM adsorbent dosage on decolorization rate (w1) and citric acid loss (w2) is shown in Fig. 7. It can be observed that w1 and w2 increase with increase in added adsorbent mass. w1 nearly remains constant when the dosage exceeds 0.2 g, which indicates that pigments in citric acid fermentation broth are almost completely removed by the adsorbent. The phenomenon can be confirmed by comparison of the photos before and after adsorption (Fig. 7b). When the adsorbent dosage equals to 0.2 g, citric acid fermentation broth is nearly colorless, and turns into a clear solution. However, w2 is proportional to the adsorbent dosage added in the solution. This is because the adsorption capacity of citric acid on the tailor-made HBCM adsorbent is small and there are still large amounts of citric acid remaining in the solution. As a result, more the quantity of adsorbent added, more the citric acid adsorbed on it. Taking both w1 and w2 into consideration, the adsorbent dosage of 0.2 g/10 mL solution was selected and used in the following study.
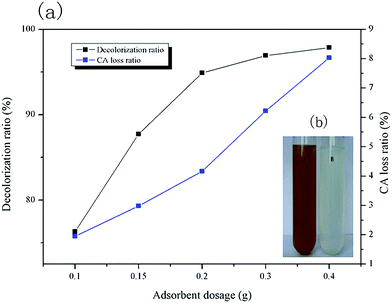 |
| Fig. 7 (a) Effects of adsorbent dosage on decolorization ratio and CA loss ratio, (b) fermentation broth images before and after adsorption. | |
Effect of pH. The solution pH is an important operating parameter that can control the adsorption behaviors of the solutes by affecting the charges of the adsorbent materials and adsorbate molecules.27 The effect of solution pH on the adsorption of pigments and citric acid are illustrated in Fig. 8. w1 nearly remains unchanged in the entire pH range. However, the adsorption percent of citric acid gradually increases in the range of pH 1–3 and decreases to zero drastically. Such a trend can be explained by the electrostatic interaction between the adsorbent and citric acid. According to the results of FTIR and pHpzc, there are several weak acid groups, namely hydroxyl groups and carboxyl groups, present in the adsorbent, which are highly dependent on the solution pH. Below pH 3, the adsorbent is positively charged, whereas above 3, the adsorbent is negatively charged. At the same time, the citric acid molecules possess three carboxyl groups. The pKa1 of citric acid is equal to 3.13, which indicates that citric acid molecules dominate at pH 1–3. However, their ionization extent is enhanced with the increase of solution pH. When pH is above 3, citric acid molecules are largely ionized. Consequently, electrostatic attraction improves citric acid adsorption capacity in the pH range of 1–3. When the solution pH is increased further, deprotonation of the surface functional groups (hydroxyl and carboxyl groups) makes the adsorbent inaccessible for citric acid ions due to electrostatic repulsion effect. Similar results were reported by K. S. Tong et al.32 They found that Cu2+ adsorption is constant when the pH is below pHpzc, while Cu2+ uptake increases significantly from 48.30% to 81.70% when pH is above pHpzc due to the electrostatic interaction. The initial solution pH is selected as 7, since at this pH, the adsorption of citric acid is nearly equal to zero. The effect of citric acid on pigment adsorption can be ignored, and the adsorption performance of pigments is rarely affected by pH.
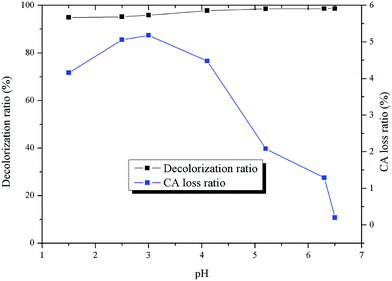 |
| Fig. 8 Effects of pH on adsorption. | |
Adsorption isotherms. The adsorption isotherms of pigments on HBCM were determined at the temperature of 298, 308, and 318 K. Several commonly used isotherm models were used to evaluate the characteristics of the adsorption process. The detailed model equations are as follows: |
 | (4) |
Eqn (3) and (4) describe the Freundlich28 and the Langmuir29 models, respectively, where qe is the equilibrium adsorption capacity (mg g−1), ce is the equilibrium concentration of pigments in solution (mg L−1), qmax is the maximum adsorption capacity (mg g−1), Kf ((mg g−1) (L mg−1)1/n) and 1/n are temperature dependent constants of the Freundlich model, and KL is the equilibrium constant (L mg−1) of the Langmuir model. Generally, the Freundlich isotherm model is an empirical equation describing adsorption on a heterogeneous surface, and the value of n indicates whether the process is favorable. The Langmuir isotherm model suggests that adsorption occurs on homogeneous sites within an adsorbent since each molecule possesses constant enthalpy and sorption activation energy. The calculated dimensionless separation factor (RL) indicates the possibility of adsorption being irreversible (RL = 0), favorable (0 < RL < 1), linear (RL = 1), or unfavorable (RL > 1).29It can be observed from the fitted curves shown in Fig. 9 that the qe value increases with increase in ce. This is attributed to the higher driving force provided by the higher initial pigment concentration to overcome mass transfer resistance between aqueous and solid phases.31 With an increase in temperature, a larger pigment adsorption capacity is observed but the change is insignificant, which indicates that the adsorption process is endothermic and temperature is not the main factor influencing it. In addition, from the isothermal parameters summarized in Table 3, the correlation coefficient indicates that both the Freundlich and Langmuir models are substantially fitted to the experimental data. The Langmuir isotherm model better fits the experimental data. It can be further suggested that there exist plenty of equivalent adsorption sites on the adsorbent, and adsorbed pigments do not interact or compete with each other. Moreover, the calculated values of RL given in Table 3 are all between 0 and 1, indicating a favorable adsorption process. All analysis results confirm that the HBCM adsorbent possesses excellent pigment adsorption performance in the fermentation broth.
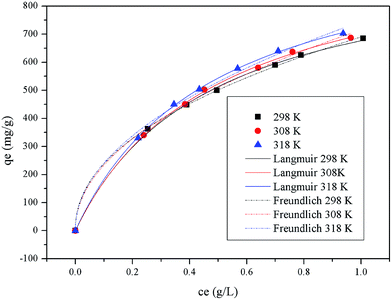 |
| Fig. 9 Adsorption isotherms of Langmuir and Freundlich models at 298, 308, and 318 K. | |
Table 3 Langmuir and Freundlich isotherm parameters for pigment removal on HBCM
T/K |
298 |
308 |
318 |
Langmuir |
KL (L−1) |
2.146 ± 0.288 |
2.170 ± 0.285 |
2.269 ± 0.231 |
qm/(mg g−1) |
976 ± 56 |
1002 ± 58 |
1026 ± 46 |
R2 |
0.994 |
0.994 |
0.997 |
RL |
0.023 |
0.023 |
0.022 |
![[thin space (1/6-em)]](https://www.rsc.org/images/entities/char_2009.gif) |
Freundlich |
KF |
679.29 ± 11.17 |
703.75 ± 12.16 |
735.38 ± 9.48 |
n |
2.177 ± 0.133 |
2.147 ± 0.127 |
2.144 ± 0.086 |
R2 |
0.996 |
0.996 |
0.998 |
Adsorption thermodynamics. In order to elucidate the adsorption mechanism and the behaviors of pigments on the HBCM adsorbent at different temperatures, thermodynamic parameters such as free energy change (ΔG), enthalpy change (ΔH), and entropy change (ΔS) were calculated using the following equations: |
 | (5) |
|
ΔG = − RT ln(Ke)
| (6) |
|
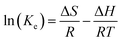 | (7) |
where Ke is the distribution coefficient between qe and ce, R is the universal gas constant (8.314 J mol−1 K−1), and T (K) is the solution temperature.According to the pigments' adsorption isotherms at different temperatures, the corresponding Ke values are calculated (Table 3). The values of ΔG are calculated by eqn (6) and ΔH and ΔS values are determined by the slope intercept of Van't Hoff plot. According to the above equations, the calculated values of ΔG at different temperatures are −0.88, −1.06 and −1.33 kJ mol−1 at the temperature of 298, 308 and 318 K, respectively. The values of ΔH and ΔS are 5.83 kJ mol−1 and 0.02247 kJ K−1 mol−1, respectively. These thermodynamic parameters confirm the spontaneous adsorption nature of the studied systems and indicate that the adsorption process is endothermic. On the other hand, the value of ΔS decreases with the loading of pigments on the external and internal surfaces of the HBCM adsorbent. The decreased randomness at the solid-solution interface can be explained by the fact that pigments in solution are adsorbed on the adsorbent, thus decreasing the randomness of pigments in the solution.
Adsorption kinetics. Commonly used adsorption kinetic models, namely the pseudo-first-order and pseudo-second-order kinetics models and the Weber and Morris model30 are used to describe the pigment adsorption process on HBCM. The corresponding model equations are shown below: |
 | (9) |
|
 | (10) |
where qt (mg g−1) represents the amount of solute adsorbed on adsorbent at time t (min), k1 and k2 are the corresponding rate constants of the pseudo-first-order and pseudo-second-order adsorption kinetics, respectively, kd (mg (g min1/2))−1 is the intra-particle diffusion rate constant, and C (mg g−1) is the adsorption constant indicating the thickness of the boundary layer, i.e., larger the value of C, greater the boundary layer effect.The adsorption of pigments on HBCM, together with the predictions of pseudo-first-order and pseudo-second-order kinetics models, is shown in Fig. 10(a). It can be observed that pigments are adsorbed on HBCM rapidly in the initial stage and ultimate equilibrium is obtained after about 60 minutes. The higher temperature is advantageous for adsorption capacity and adsorption rate. The results indicate that the adsorption process is endothermic in nature, in accordance with the above results. The pseudo-second-order model is more suitable to describe the kinetics experimental data (see R2 values given in Table 4). Moreover, comparing with the FTIR of HBCM before and after adsorption (Fig. 4), the characteristic adsorption peaks change slightly, namely, the peak at 3420 cm−1 is shifted to 3400 cm−1 with decreased intensity and the adsorption peaks representing the aromatic cores weaken. All results indicate that these groups effectively participated in pigment adsorption.
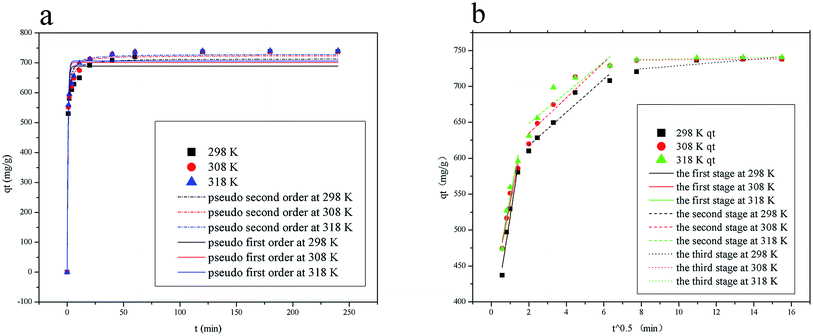 |
| Fig. 10 (a) Adsorption kinetics curve according to pseudo-first-order or pseudo-second-order equation and (b) intraparticle diffusion plots at different temperatures. | |
Table 4 R2 and constant values for different adsorption kinetics models
T/K |
298 |
308 |
318 |
Pseudo-first-order equation |
k1/(min−1) |
113 ± 0 |
131 ± 0 |
149 ± 0 |
qe(theo-1)/(mg g−1) |
689 ± 19.1 |
701 ± 15.3 |
707 ± 14.1 |
qe(exp)/(mg g−1) |
738 |
738.1 |
740.4 |
R2 |
0.944 |
0.95 |
0.958 |
![[thin space (1/6-em)]](https://www.rsc.org/images/entities/char_2009.gif) |
Pseudo-second-order equation |
k2/(g mg−1 min−1) |
0.00317 ± 0.00053 |
0.00336 ± 0.00051 |
0.00358 ± 0.00048 |
qe(theo-1)/(mg g−1) |
714 ± 10.3 |
725 ± 9.10 |
729 ± 7.75 |
qe(exp)/(mg g−1) |
738 |
738.1 |
740.4 |
R2 |
0.983 |
0.987 |
0.991 |
![[thin space (1/6-em)]](https://www.rsc.org/images/entities/char_2009.gif) |
Intra-particle diffusion model |
Kt1/(mg g−1 min−1/2) |
167 |
132 |
142 |
C1/(mg g−1) |
352 |
406 |
404 |
R2 |
0.948 |
0.936 |
0.914 |
Kt2/(mg g−1 min−1/2) |
23.1 |
24.6 |
21.4 |
C2/(mg g−1) |
572 |
586 |
606 |
R2 |
0.917 |
0.875 |
0.787 |
Kt3/(mg g−1 min−1/2) |
2.22 |
0.212 |
0.406 |
C3/(mg g−1) |
706.8 |
735 |
735 |
R2 |
0.633 |
0.536 |
0.772 |
It is well known that adsorption of adsorbate from solution to the surface of adsorbent particles include several steps, namely film or external diffusion, pore diffusion, surface diffusion and adsorption on the pore surface.29 The plot of qt versus t/2 is shown in Fig. 10(b). Multiple linear lines can be observed. In the first adsorption stage, the external mass transfer controls the adsorption process. Slow adsorption occurs in the second and third stages, which indicates that intra-particle diffusion dominates the adsorption process. The values of Kd listed in Table 4 show that pigment adsorption on HBCM is controlled by external mass transfer, followed by intra-particle diffusion mass transfer as well.
Conclusion
Tailor-made HBCM is an efficient adsorbent to decolor citric acid fermentation broth. In our experiment, most pigments were removed by use of 2% (0.2 g/10 mL) dosage, with a citric acid loss maintained below 5%. When the solution pH was adjusted to 7, the citric acid loss ratio was nearly zero. The Langmuir model better fitted the adsorption isotherms, indicating that adsorption sites on HBCM were equivalent and the adsorbed pigments did not interact or compete with each other. The calculated thermodynamic parameters revealed the spontaneity and endothermic characteristics of the adsorption process. Moreover, the adsorption process was pseudo-second order. Adsorption rate was controlled by external mass transfer and the intra-particle diffusion. In addition, the FTIR results indicated that functional groups were involved in pigment adsorption, which enhanced pigment adsorption capacity and selectivity.
Acknowledgements
Project was supported in part by the Major Research Plan of the National Natural Science Foundation of China (Grant No. 21390204). We would also like to acknowledge the financial support provided by 21306086, 2013CB733602, 21636003, IRT_14R28, PAPD, 21606128 and BK20151452.
References
- S. Kent Hoekman, A. Broch and C. Robbins, Hydrothermal Carbonization (HTC) of Lignocellulosic Biomass, Energy Fuels, 2011, 25, 1802–1810 CrossRef.
- S. Q. Guo, X. Y. Dong, K. T. Liu, H. L. Yu and C. Zhu, Chemical, energetic, and structural characteristics of hydrothermal carbonization solid products for lawn grass, BioResources, 2015, 10(3), 4613–4625 CAS.
- S. K. Hoekman, A. Broch and C. Robbins, Hydrothermal carbonization (HTC) of lignocellulosic biomass, Energy Fuels, 2011, 25(4), 1802–1810 CrossRef CAS.
- G. K. Parshetti, S. K. Hoekman and R. Balasubramanian, Chemical, structural and combustion characteristics of carbonaceous products obtained by hydrothermal carbonization of palm empty fruit bunches, Bioresour. Technol., 2013, 135, 683–689 CrossRef CAS PubMed.
- M. Azharul Islam, I. A. W. Tan, A. Benhouria, M. Asif and B. H. Hameed, Mesoporous and adsorptive properties of palm date seed activated carbon prepared via sequential hydrothermal carbonization and sodium hydroxide activation, Chem. Eng. J., 2015, 270, 187–195 CrossRef.
- A. Jain, R. Balasubramanian and M. P. Srinivasan, Hydrothermal conversion of biomass waste to activated carbon with high porosity: A review, Chem. Eng. J., 2016, 283, 789–805 CrossRef CAS.
- Z. Liu and F. S. Zhang, Removal of lead from water using biochars prepared from hydrothermal liquefaction of biomass, J. Hazard. Mater., 2009, 167, 933–939 CrossRef CAS PubMed.
- B. R. Selvi, D. Jagadeesan, B. Suma, G. Nagashankar, M. Arif, K. Balasubramanyam, M. Eswaramoorthy and T. K. Kundu, Intrinsically fluorescent carbon nanospheres as a nuclear targeting vector: delivery of membraneimpermeable molecule to modulate gene expression in vivo, Nano Lett., 2008, 8, 3182–3188 CrossRef CAS PubMed.
- X. Wang, C. Hu, Y. Xiong, H. Liu, G. Du and X. He, Carbon-nanosphere-supported Pt nanoparticles for methanol and ethanol electro-oxidation in alkaline media, J. Power Sources, 2011, 196, 1904–1908 CrossRef CAS.
- M. Sevilla, J. A. Maciá-Agulló and A. B. Fuertes, Hydrothermal carbonization of biomass as a route for the sequestration of CO2: chemical and structural properties of the carbonized products, Biomass Bioenergy, 2011, 35, 3152–3159 CrossRef CAS.
- K. Sun, K. Ro, M. Guo, J. Novak, H. Mashayekhi and B. Xing, Sorption of bisphenol-A, 17a-ethinyl estradiol and phenanthrene on thermally and hydrothermally produced biochars, Bioresour. Technol, 2011, 102, 5757–5763 CrossRef CAS PubMed.
- B. Liu, Y. Li, X. Gai, R. Yang, J. Mao and S. Shan, Exceptional Adsorption of Phenol and p-Nitrophenol from Water on Carbon Materials Prepared via Hydrothermal Carbonization of Corncob Residues, BioResources, 2016, 11(3), 7566–7579 Search PubMed.
- Z. Liu, F. S. Zhang and J. Wu, Characterization and application of chars produced from pinewood pyrolysis and hydrothermal treatment, Fuel, 2010, 89, 510–514 CrossRef CAS.
- A. Jain, R. Balasubramanian and M. P. Srinivasan, Hydrothermal conversion of biomass waste to activated carbon with high porosity: a review, Chem. Eng. J., 2016, 283, 789–805 CrossRef CAS.
- Z. Liu and F.-S. Zhang, Removal of copper and phenol from aqueous solution using porous carbons derived from hydrothermal chars, Desalination, 2011, 267, 101–106 CrossRef CAS.
- L. Wang, Y. Guo, B. Zou, C. Rong, X. Ma, Y. Qu, Y. Li and Z. Wang, High surface area porous carbons prepared from hydrochars by phosphoric acid activation, Bioresour. Technol., 2011, 102, 1927–1950 Search PubMed.
- J. Bohdzieweicz and M. Bodzek, Ultrafiltration preparation of liquid pectinolytic enzyme solution from citric acid fermentation broth, Recents Prog. Genie Procedes, 1992, 449–454 CAS.
- J. Wang and P. Liu, Comparison of citric acid production by Aspergillus niger immobilized in gels and cryogels of
polyacrylamide, J. Ind. Microbiol., 1996, 16, 351–353 CrossRef CAS.
- P.-P. Zhou, J. Meng and J. Bao, Fermentative production of high titer citric acid from corn stover feedstock after dry dilute acid pretreatment and biodetoxification, Bioresour. Technol., 2017, 224, 563–572 CrossRef CAS PubMed.
- S. Brunauer, P. H. Emmet and F. Teller, J. Am. Chem. Soc., 1938, 60, 309 CrossRef CAS.
- E. P. Barret, L. G. Joyer and P. P. Halenda, The determination of pore volume and area distributions in porous substances: 1. Computations from nitrogen isotherms, J. Am. Chem. Soc., 1951, 73, 373–380 CrossRef.
- I. D. mall, V. C. Srivastava, G. V. A. Kumar and I. M. Mashra, Characterization and utilization of mesoporous fertilizer plant waste carbon for adsorptive removal of dyes from aqueous solution, Colloids Surf., A, 2006, 278, 175–187 CrossRef CAS.
- S. A. Torrellas, R. G. Lovera, N. Escalona, C. Sepúlveda, J. L. Sotelo and J. García, Chemical-activated carbons from peach stones for the adsorption of emerging contaminants in aqueous solutions, Chem. Eng. J., 2015, 279, 788–798 CrossRef CAS.
- H. Yang, R. Yan, H. Chen, C. Zheng, D. Ho Lee and D. T. Liang, In-Depth Investigation of Biomass Pyrolysis Based on Three Major Components: Hemicellulose, Cellulose and Lignin, Energy Fuels, 2006, 20, 388–393 CrossRef CAS.
- M. Jagtoyen and F. Derbyshire, Activated carbons from yellow poplar and white oak by H3PO4 activation, Carbon, 1998, 36, 1085–1097 CrossRef CAS.
- S.-H. Hsu, C.-S. Huang, T.-W. Chung and S. Gao, Adsorption of chlorinated volatile organic compounds using activated carbon made from Jatropha curcas seeds, J. Taiwan Inst. Chem. Eng., 2014, 45, 2526–2530 CrossRef CAS.
- S. Babel and T. A. Kurniawan, l Cr(VI) removal from synthetic wastewater using coconut shell charcoal and commercialactivated carbon modified with oxidizing agents and/or chitosan, Chemosphere, 2004, 54(7), 951–967 CrossRef CAS PubMed.
- H. Freundlich, Adsorption in solution, Z. Phys. Chem., 1906, 57, 384–470 Search PubMed.
- I. Langmuir, The constitution and fundamental properties of solids and liquids, J. Am. Chem. Soc., 1916, 38, 2221–2295 CrossRef CAS.
- P. D. Pathak and S. A. Mandavgane, Preparation and characterization of raw and carbon from banana peel by microwave activation: application in citric acid adsorption, J. Environ. Chem. Eng., 2015, 3, 2435–2447 CrossRef CAS.
- M. Ghaedi, A. G. Nasab, S. Khodadoust, M. Rajabi and S. Azizian, Applications of activated carbons as the adsorbents for methylene blue removal: kinetics and equilibrium studies, J. Ind. Eng. Chem., 2014, 20, 2317–2324 CrossRef CAS.
- W. J. Weber and J. C. Morris, Kinetics of adsorption on carbon from solution, J. Sanit. Eng. Div., Am. Soc. Civ. Eng., 1963, 89, 31–59 Search PubMed.
|
This journal is © The Royal Society of Chemistry 2017 |