DOI:
10.1039/C7RA03940A
(Paper)
RSC Adv., 2017,
7, 30397-30410
Transparent TiO2 nanotubes on zirconia for biomedical applications
Received
6th April 2017
, Accepted 16th May 2017
First published on 12th June 2017
Abstract
Tissue discoloration in dental implant patients with thin gingival tissue is one of the many causes of dental implants’ revision surgery. Therefore, the purpose of this study is to address this issue by developing a surface that has a “tooth like bright colored” appearance while at the same time enhancing the bone implant integration. A biomimetic surface is fabricated by forming transparent TiO2 nanotubes on zirconia (TTNZ) that can enhance the proliferation and attachment of human mesenchymal stem cells (hMSCs) as compared to roughened ZrO2. This surface treatment was aimed to resolve tissue discoloration and aesthetic appearance problems for dental implant patients, while also enhancing biocompatibility. TiO2 nanotubes (TNTs) were formed using an electrochemical anodization technique in an electrolyte comprised of NH4F, ethylene glycol and water. The presence of TNTs on the ZrO2 substrate was detected by field emission scanning electron microscopy (FESEM). Optical images of longer anodized (20 and 30 min) samples show the white colored appearance characteristic of ZrO2 and FESEM confirmed the presence of TNTs on anodized samples. Surface characteristics of all samples were analyzed using water contact angle analysis, Fourier-transform infrared spectroscopy, white light interferometry and FESEM. Quantitative and qualitative biocompatibility analysis of treated and non-treated ZrO2 surfaces were obtained by performing FESEM, 3-(4,5-dimethylthiazol-2-yl)-2,5-diphenyl-2H-tetrazolium bromide (MTT) assay and fluorescence microscopy. FESEM revealed well-elongated and well-spread cell morphology on the nanotubular surface as compared to roughened ZrO2. Additionally, MTT assay showed a significantly high cell proliferation for anodized Ti–ZrO2 surface as compared to roughened ZrO2 after 7 days of incubation.
Introduction
Titanium (Ti) metal has been widely utilized for biomedical applications for decades due to its beneficial surface properties that allow such foreign implants to survive within the complex biological environment.1 Additionally, according to American Academy of Implant Dentistry, 3 million population have dental implants with numbers increasing every year.2 However, tissue discoloration around dental implant sites in patients with thin gingival tissue is of great concern, since it results in dental implant revision surgeries.3,4 Therefore, much attention has been paid to resolve this shortcoming of titanium dental implants. ZrO2 is a potential alternative for titanium due to its high flexural strength, fracture resilience, and similar chemical properties to that of titanium;4–9 additionally, it is a ceramic of white colored appearance, which makes it a suitable material for patients with thin gingiva and metal ion sensitivity.
Various in vivo studies have been performed on understanding the tissue response surrounding ZrO2 implants as compared to standard titanium implants. Depprich et al. investigated the effect of roughened ZrO2 and Ti implants inside the tibia of minipigs in terms of their osseointegration property.12 The histology and histomorphometric analysis concludes that there is no significant difference in cellular response between ZrO2 or titanium implants.12 Moreover, Gahlert et al. investigated the effect of roughened ZrO2 vs. titanium implants inside the adult pigs by detecting peri-implant bone density and bone-implant contact ratio around the respective materials.13 This study also supported Depprich et al.’s data on the similar bone-implant interaction of ZrO2 in comparison with Ti implants.12 On the other hand, Gahlert et al. investigated biomechanical fixation of implants constructed from machined ZrO2, roughened ZrO2 and roughened Ti after 4, 8 and 12 weeks through torque removal testing.14 Significantly high removal torque values were obtained for roughened Ti implants followed by roughened ZrO2 and machined ZrO2.14 This indicates that the biomechanical fixation of ZrO2 implants is weak when compared to Ti. This suggests that further surface modification investigation is required for ZrO2 based implants to improve their biomechanical fixation, bone density and bone-implant contact ratio. Even though the benefits of ZrO2 are known, its ability to osseointegrate or create strong bone-implant contact remains somewhat uncertain; therefore, further study to enhance the success rate of ZrO2 implants appear to be necessary.10,11
Previously, studies have also been performed to enhance the properties of ZrO2 through surface modification including sandblasting, acid etching, anodization, and polymeric coating (i.e. poly(lactic acid) and poly(3/4-caprolactone)) etc.14,15 In the study performed by Maeda et al. osteoblast activity was monitored on poly(lactic acid) coating and imogolite (aluminum silicate nanotubes) treated poly(lactic acid) coating. From the results, it was observed that the polymer treated with aluminum silicate nanotubes enhances the MC3T3-E1 adhesion on the surface. The reason for the increase in cellular bioactivity is reported as due to rougher surface texture that is obtained after treating with imogolite.15 However, aluminum silicate poses the health risk of fibrosis if it detaches from the substrate, thereby interfering with bone-implant interaction, as reported by Elmore AR et al.16 Additionally, Frandsen et al. investigated the effect of osteoblasts on TiO2 nanotube coated ZrO2 vs. bare ZrO2. They also observed improved cell behavior on TiO2 nanotube coated ZrO2 compared to bare ZrO2.17 The main difference between their study and the present study is that (1) they used a sputtering technique to deposit titanium, whereas in our study an e-beam evaporation technique is used to deposit Ti, (2) they anodized Ti at 20 V for 15 min, which produces porous structure at low voltage, whereas in the present study, the Ti is anodized at 60 V for various times, producing tubular morphology as shown in FESEM images, (3) after anodization, in ref. 17, nanotubes were annealed to form anatase, whereas, no annealing was performed in our study and (4) in our study, white colored appearance of ZrO2 substrate is observed in the presence of TiO2 nanotubes on the surface.
It is well established that TiO2 nanotubes (TNTs) enhance the implant’s surface properties by providing nanoscale roughness for increased cellular adhesion thereby improving osseointegration compared to acid etched or sandblasted surfaces.18–21 TNTs also improve the physiochemical characteristics of surfaces as shown through their ability to maintain surface wettability for a period of at least one month compared to smooth or rough Ti surfaces.22–24 Hydrophilic surfaces attract more fibronectin (FN) and vitronectin (VN) protein from the body fluids, which are essential sites for cellular attachment and proliferation.21,25,26 Additionally, TNTs have the capability to store drugs of interest such as BMP2, TGF-beta etc. for patients suffering from osteoporotic bone and being treated with growth factors to stimulate bone-ingrowth onto the implants.27,28 These nanotubes may also act as a drug reservoir for anti-infection or anti-inflammatory drugs whose controlled and systematic release to the implant site may prevent complications due to post-surgery biological reactions.29–32 To-date, these aforementioned benefits of TNTs have been introduced on CP-Ti or Ti-alloy surfaces. In this study, a hybrid surface is prepared with transparent TNTs on ZrO2 substrates that possess white colored appearance, alleviate the possibility of metal ion allergic reactions, and provide benefits of nanotubular features.
The objectives of this study were to (1) fabricate surfaces with transparent titania nanotubes on zirconia substrates (TTNZ), (2) characterize their physiochemical and chemical surface characteristics with water contact angle analysis, Fourier transform infrared spectroscopy, white light interferometry, field emission scanning electron microscopy (FESEM) and optical appearance, and (3) investigate the biocompatibility of each surface using MTT assay, fluorescence microscopy and FESEM.
Methods and materials
Sample preparation
ZrO2 discs of 15 mm diameter and 1 mm thickness were employed for the experiments. All discs were roughened by sandblasting with 50 μm Al2O3 particles (Trinity Tool Company, Fraser MI, USA) at 517 kPa for 10 s. Following this sandblasting procedure, all discs were cleaned by sequential sonication in ethanol and de-ionized (DI) water for 15 min. Cleaned ZrO2 discs were then coated with a 500 nm thick titanium film using e-beam evaporation employed at Nanotechnology Core Facility (NCF) at University of Illinois at Chicago (UIC). Ti deposited ZrO2 samples are designated as Ti–ZrO2. The base pressure of reactor was maintained at 5 × 10−7 Torr with a rotating sample holder to obtain even film growth. Titanium evaporation current was maintained at 47 mA and the voltage was 10 V. The growth rate of titanium on ZrO2 was measured to be ∼1 Å s−1. Ti–ZrO2 samples were then anodized using an electrochemical anodization technique, where Ti–ZrO2 was the working electrode (WE) and graphite was the counter electrode (CE). Electrodes were connected to the voltage source (Keithley 2400 SourceMeter, Cleveland, OH, USA), which supplied a constant voltage of 60 V for 10, 20 and 30 min. The anodization electrolyte was comprised of 2 vol% H2O and 0.25 wt% NH4F (Sigma-Aldrich, St. Louis, MO, USA) in ethylene glycol (Fisher Scientific, Pittsburgh, PA, USA) and it was continuously stirred with a magnetic stirrer to maintain a homogeneous environment throughout anodization. A total of four groups were investigated in this study: roughened ZrO2, 10TNZ (10 min anodized sample with TiO2 nanotube coated ZrO2), 20TTNZ (20 min anodized samples with transparent TiO2 nanotubes coated zirconia), and 30TTNZ (30 min anodized samples with transparent TiO2 nanotubes coated zirconia).
Surface characterizations
Field emission scanning electron microscopy (FESEM, JSM-6320F, JEOL, Musashino 3-chome Akishima Tokyo, Japan) was used to analyze the external surface morphology of roughened ZrO2, 10TNZ, 20TTNZ and 30TTNZ samples. The sample of interest was mounted on an aluminum stub with the help of double sided conductive carbon tape for imaging. FESEM images were obtained with acceleration voltages of 3.8 kV, and specimens were observed with magnifications of 10
000× and 50
000×. White light interferometry (WLI, NewView 6300, Zygo Corporation, Middlefield, Connecticut, USA) in Tribology Lab at Rush University Medical Center was used to measure surface roughness of ZrO2, and anodized Ti–ZrO2 samples. Transparency of the anodized samples were observed by holding the samples against white light where the top surface of the samples faced the light and the optical image was captured from the back side, as shown in graphical abstract. This allows the light to pass through if the sample is transparent and no light passes through if the sample is non-transparent. Diffuse reflectance Fourier-transform infrared spectrometry (FTIR, Nicolet, Madison, WI, USA) was used to probe the surface composition of ZrO2 and anodized Ti–ZrO2 samples. ZrO2 was used as a background for ZrO2 sample scans; moreover, non-anodized Ti–ZrO2 was used as a background for anodized Ti–ZrO2 sample scans. Before running the FTIRS experiment, each sample was kept inside the FTIRS chamber for 1 h in order to stabilize the internal conditions and optimize the signal to noise ratio. FTIRS spectra were obtained with 2 cm−1 resolution and 512 scans over the wavenumber range of 400–4000 cm−1. Qualitative analysis by de-convoluting FTIR spectra was performed using spectral peak-fitting software with a linear background and Gaussian–Lorentian peak shape function.
Surface wettability
Water contact angle (WCA) measurements were obtained using a sessile drop method with the help of Goniometer (Rame’-Hart NRL, Succasunna, NJ, USA) to determine the surface hydrophilicity. During WCA measurements, 5 μL of DI-water was placed on the respective surfaces followed by image capturing. The WCA on each surface was analyzed using Image-J Analysis software. The wettability of all samples was monitored after 0, 1, 2, 3 and 6 days and thereafter every 7 days for a period of 1 month. Before measuring WCA on day 0, all the samples were first cleaned with methanol for 10 min followed by a DI water wash for 10 min. For the rest of the measurements over a period of one month, each sample was merely cleaned by blow-drying with N2 gas (Grade 4.8, 99.998%, NIZ300 Progressive Industries Inc., Sylacauga, Al, USA) before performing WCA. After WCA measurements, samples were stored in a plastic Petri dish until the next measurement.
In vitro cell viability tests
Human Mesenchymal Stem Cells (hMSCs) (derived from adult bone marrow, provided by Tulane University) were used for cell viability tests on each surface. Prior to culturing cells on different substrates, hMSCs were made ∼80–90% confluent in a cell culture media composed of Dulbecco’s modified Eagle medium (DMEM; Hyclone, GE Healthcare Life Sciences, PA, USA) supplemented with 10% fetal bovine serum (FBS; Gibco by Life Technologies, NY, USA) and 1% penicillin and streptomycin (Gibco by Life Technologies, NY, USA). The incubator environment was kept at 37 °C and 5% CO2. After cells reached 80–90% confluence, they were trypsinized (Hyclone, GE Healthcare Life Sciences, PA, USA) to detach them, and then centrifuged at 1200 rpm for 5 min. The supernatant fluid was discarded and cells were washed twice with phosphate buffer saline (PBS, Gibco by Life Technologies, NY, USA). Then the cells were re-suspended in culture media to obtain healthy cells for culturing. All samples were first sterilized in 70% ethanol. After sterilizing the samples, 2 × 104 cells were cultured on each substrate. The samples seeded with cells were incubated at 37 °C and 5% CO2 environment.
MTT assay. Cell viability was detected by measuring the absorbance using the MTT assay after 1 and 6 days of incubation in 37 °C and 5% CO2. First, 3-(4,5-dimethylthiazol-2-yl)-2,5-diphenyl-2H-tetrazolium bromide (MTT, Sigma Aldrich, St. Louis, MO, USA) was diluted in PBS with a concentration of 0.5 mg of MTT l−1 of solution. After preparing the MTT solution, culture media and MTT solution were mixed in 1
:
1 v/v ratio. First, the cells were washed with PBS twice. Then 500 μl of mixed MTT and culture media solution was added to the cells on the discs. The cells were again incubated for 4 h. Then 500 μl of dimethyl sulfoxide (DMSO, Fisher Scientific, Pittsburgh, PA, USA) was added to each well. Then again the cells were incubated for 24 h. The absorbance signal was measured using a fluorescence reader (SpectraMax Plus, Molecular Devices, Sunnyvale, CA, USA) with 570 nm and 600 nm wavelengths.
Fluorescence microscopy. Cell spreading morphology was observed after 3 and 24 h of seeding. Prior to fluorescence microscopy, cells were fixed with 3.7% formaldehyde in PBS for 15 min at room temperature. Cells were stained using ActinRed 555 Readyprobe (Molecular Probes by Life Technologies, Grand Island, NY, USA) for 30 min, NucBlue (Molecular Probes by Life Technologies, Grand Island, NY, USA) for 20 min, and 0.1% Triton X-100 for 15 min to probe the nucleus and actin filaments of the cells.
FESEM. Cell morphology was observed after 6 days. Prior to FESEM, cells were fixed with 3.7% formaldehyde (Fisher Scientific, Pittsburgh, PA, USA) in PBS for 15 min at room temperature. The cells were then rinsed with PBS for 15 min four times. Then the cells were dehydrated in increasing concentration of ethanol at 25, 50, 75, 90 and 100% for 10 min once except for 100% ethanol dehydration, which was performed twice. Prior to FESEM imaging, samples fixed with cells were sputter coated with ∼5–7 nm Au/Pd coating. FESEM images were obtained with acceleration voltages of 10 kV, and specimen with 2000 magnifications.
Statistical analysis
SPSS software (SPSS v. 20.0, SPSS Inc., Chicago, IL, USA) was used to perform statistical analysis. One-way ANOVA was used to determine the statistical difference in surface roughness and wettability before and after anodization, and the dimensions of the nanotubes upon different anodization times. Tukey Honest Significant Difference (HSD) post hoc analysis was used for pairwise comparison within and between each group. For all the analysis, p-values <0.05 were considered as statistically significant.
Results and discussion
Current–time behavior
Formation of TiO2 nanotubes on Ti through electrochemical anodization process is divided into three stages: (i) field assisted oxidation, where Ti oxidizes to TiO2, (ii) field assisted dissolution, where nucleation of pore formation is initiated and (iii) equilibrium between field assisted oxidation and dissolution, where formation and dissolution of TiO2 takes place at a constant rate.30–32 Fig. 1 shows the current–time transient curve of Ti–ZrO2 samples during anodization for the first 10 min. Such a graph allows extrapolating the information related to the chemical reaction on the surface that leads to the formation of well-aligned nanotubular structures.
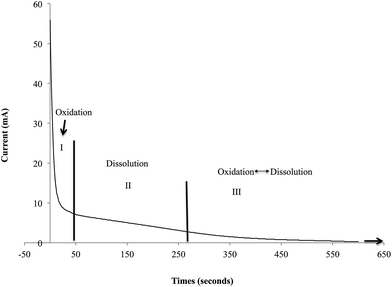 |
| Fig. 1 Current–time transient analysis of Ti–ZrO2 sample in an electrolyte composed of ammonium fluoride, ethylene glycol, and water at 60 V. This figure shows the current profile during the anodization process. In region I, a drastic drop in current is observed, which is due to the oxidation of Ti. In region II, the current stabilizes, which is due to the dissolution of TiO2 and limitation in O2− diffusion through the barrier layer. In region III, the rate of ionic exchange decreases even further, which is due to the equilibrium between oxidation and dissolution and having ZrO2 ceramic as the substrate, which further prevents ionic exchange. | |
The current–time curve of Ti–ZrO2 shows three stages. In stage 1, a drastic drop in the current is observed. This significant current drop is due to the formation of a barrier TiO2 layer on the Ti surface when 60 V is applied. Initially, mass evolution of H2 gas is observed near the cathode (graphite) due to the high electron conductivity.31 This H2 gas evolution arises from the dissociation of H2O from electrolyte that releases H+ ions along with OH− and O2− ions. H+ ions combine to form H2 at the cathode, whereas, negatively charged ions (i.e. OH− and O2−) migrate towards the anode (Ti–ZrO2) and diffuse to the Ti/TiO2 interface, where they react with Ti4+ ions and form TiO2 and/or Ti(OH)4.21,33,34 Consequently, the oxidation process of stage I is dominated on the surface initially. In stage II, the current profile is observed to steadily decrease indicating lessening of oxidation, with stabilizing of the current likely due to limitation in electron conductivity;31,33 simultaneously, this stabilization may also be resulted from the nucleation of pore formation on the surface, which explains the initiation of ionic conductivity on the surface (TiO2/electrolyte interface).31 The generation of pores increases the surface area of Ti–ZrO2 that facilitates the diffusion of ionic species (i.e. O2−, OH−, Ti4+, or F−) into/from the anode in comparatively large amounts, thereby decreasing the resistance of the film.31,34 During stage II, F− ions from ammonium fluoride, react with TiO2 and Ti(OH)4 to form TiF62−, which is soluble in electrolyte, thereby forming pores and well organized nanotubes.33,34 Finally, in stage III, a further decrease and stabilization of the current is observed. During this phase, the rate of TiO2 formation at the Ti/TiO2 interface and the rate of TiO2 dissolution at the TNTs pore bottom is in equilibrium. Because there is limited amount of Ti (500 nm-thick) on the ZrO2 substrate, once the titanium layer has fully oxidized, the dissolution reaction would be the leading reaction. In addition, although dissolution is the leading reaction, current will not increase because the underlying substrate of titanium is ZrO2 ceramic that resists ionic and electronic conduction. Due to these reasons, transparent nanotubes are obtained at longer anodization duration (20 and 30 min) as shown in Fig. 2.
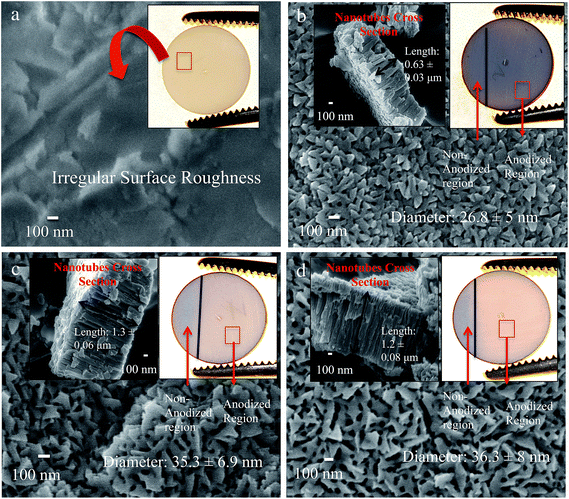 |
| Fig. 2 FESEM images for (a) ZrO2, (b) 10 min anodized Ti–ZrO2, (c) 20 minute anodized Ti–ZrO2, and (d) 30 min anodized Ti–ZrO2. Right upper insets show the optical appearance of each sample before and after electrochemical treatment and the cross section shown left displays the length of TiO2 nanotubes. Anodization was performed in 2 vol% H2O, 0.25 wt% NH4F and EG. | |
Field emission scanning electron microscopy (FESEM)
Fig. 2 shows the FESEM images of ZrO2 and anodized Ti–ZrO2 samples. Fig. 2a shows the surface morphology of untreated ZrO2 sample. It is observed that the surface possesses irregular roughness, which is from the sandblasting procedure. The upper inset on Fig. 2a shows the optical visualization of ZrO2 samples, which displays a bright “tooth-color” appearance. Fig. 2b shows the surface of e-beam evaporation deposited titanium on ZrO2 substrates (Ti–ZrO2) post 10 min of electrochemical treatment. The surface topography of 10 min anodized Ti–ZrO2 samples (10TNZ) shows that nanotubes with 26.8 ± 4.5 nm diameter are observed evenly throughout the surface with length 0.63 ± 0.03 μm (as shown in upper left inset, Fig. 2b). The optical image of 10 min anodized samples display similar gray colored appearance similar to non-anodized Ti region. This gray colored appearance of 10TNZ samples likely arises from a non-anodized titanium layer between the top TNT layer and bottom ZrO2 substrate. When anodization duration is 10 min, only some titanium might have been anodized to form TiO2 nanotubes (TNTs) leaving a layer of non-anodized Ti, thereby providing a surface with three layers (TNT layer, non-anodized Ti, and ZrO2 substrate), imparting an unfavorable gray colored appearance of Ti. This indicates that at low anodization duration (10 min), non-transparent nanotubes are obtained (schematic representation in graphical abstract).
Similarly, Fig. 2c and d show the surface topography and cross section of 20 (20TTNZ) and 30 (30TTNZ) min anodized Ti–ZrO2 samples, respectively. Compared to 10TNZ samples, the density of the pores on 20TTNZ and 30TTNZ samples has decreased. This is due to the widening of the pore diameter from 26.8 ± 4.5 nm (10TNZ) to 35.3 ± 6.9 nm (20TTNZ) and 36.3 ± 8 nm (30TTNZ). According to Tukey HSD post hoc analysis, an increase in diameter between 10 and 20 or 30 min anodized surface is significant as concluded by p < 0.05. Additionally, a significant increase in length was observed for 20TTNZ (1.3 ± 0.06 μm) and 30TTNZ (1.2 ± 0.08 μm) (Tukey HSD post hoc analysis with p < 0.05), as compared to 10TNZ samples (0.63 ± 0.03 μm), which is shown in Fig. 2c and d. This increase in length of TNTs, when anodization duration increases to 20 and 30 min, is due to the presence of non-anodized Ti layer after 10 min anodization, which can further oxidize and dissolve to form nanotubes. When the anodization duration is increased to 20 and 30 min, longer nanotubes are obtained of the order of 1 μm. On the other hand, no significant difference was observed in TNTs length when anodization duration was increased from 20 to 30 min (Tukey HSD post hoc analysis p > 0.05). This stabilization in the growth of nanotubes length after 20 min is likely due to the complete anodization of 500 nm deposited Ti within 20 min, which leaves no Ti for further oxidation and dissolution process on extension to 30 min.
Additionally, the length of the nanotubes is observed to be higher (∼1.3 μm for 20 and 30 min anodization) than the thickness of deposited titanium (500 nm-thick). There are two factors affecting the expansion of the film: (1) Pilling–Bedworth ratio and (2) flow model mechanism of the nanotubes formation. The Pilling–Bedworth ratio (PBR) is the metal expansion factor when it is converted into an oxide.33 This means that when titanium is exposed to the oxygen source, titanium splits into Ti4+ and 4e− ions, which then react with O2− to form TiO2.34 As the oxide thickness increases, the Ti4+ ions diffuse towards the surface and O2− diffuse towards the metal/oxide interface. This oxidation process introduces a volume expansion to the metal.35,36 Such volume ratio of oxide to metal is the PBR factor.35,36 The PBR of amorphous TiO2 has been reported to be 2.43, which is the ratio of amorphous TiO2 to Ti metal.33 Additionally, according to the flow mechanism of nanotubes formation, when the voltage is applied to the titanium, the strong electric field across the oxide layer induces stress in the barrier layer due to volume expansion caused by ion movements within the oxide layer. Such volume expansion and ionic transport exerts compressive stress along the tubular oxide interface due to the high electric field zone in the nanotubes, thereby facilitating the plastic flow/movement of barrier oxide towards the walls of the nanotubes.30,36,37
Optical images of 20TTNZ (Fig. 2c) and 30TTNZ (Fig. 2d) samples show that a transparent nanotubular layer is obtained when anodization is performed for 20 and 30 min. The anodized region of 20TTNZ and 30TTNZ show similar optical appearance to that of ZrO2 samples, unlike 10TNZ samples. This suggests that when Ti–ZrO2 samples were anodized for longer duration (20 and 30 min), all deposited Ti might have anodized completely, thereby providing the surface with two layers (transparent TiO2 nanotubes layer and ZrO2 layer) imparting a bright colored appearance characteristic of ZrO2. Such transparent appearance of substrate when complete anodization occurs was also observed in previous studies, however, their substrate was ITO instead of ZrO2.38–40 TNT decorated implants have shown many potential benefits for implants; however, over time, the quality of patients’ life is affected due to the tissue discoloration in patients with thin gingival tissues and metal allergic reactions. In this study, from FESEM and optical imaging, it is observed that decorating ZrO2 substrates (light colored ceramic) with TNTs provides a novel bio-implant surface that provides all the benefits of TNTs. It is light in color compared to titanium implants, and it can prevent tissue discoloration in patients with thin gingival tissue and reduces allergic reaction to metals. In order to obtain high resolution cross-section of the nanotubes, further studies are required using a focused ion beam to obtain high-resolution TEM images of nanotubes on the Ti–ZrO2 samples.
Fourier transform infrared spectroscopy
FTIRS data of the ZrO2 sample is shown in Fig. 3. In the spectrum, the absorption peaks are observed for gas phase CO2 at 2200–2400 and 667 cm−1 and H2O in the ranges of 3600–3800 and 1400–1900 cm−1, which are from the residual air of FTIR purging chamber. Additionally, absorption peaks featured at the lower wavenumber of 560 and 450 cm−1 are due to Zr–O and Zr–O2–Zr vibrational stretch, respectively.41,42 Fig. 3b shows the de-convoluted spectrum of the intense peak observed in the region 755–915 cm−1. This region is the superposition of various absorption bands including Zr–O at 793 and 815 cm−1 and C–O at 854 cm−1, respectively.43–45 Absorption peaks for C–O is likely from carbon dioxide adsorbed on to ZrO2.46 Fig. 4 shows the FTIRS spectrum of anodized Ti–ZrO2 samples. An absorption peak around 535 cm−1 is observed, which is the apparent feature of a Ti–O bond stretch.47–49 Additionally, superposition of multiple peaks in the region 600–2300 cm−1 is also observed, which is de-convoluted in Fig. 4b. Previously, it has been concluded that anodizing titanium in ammonium fluoride and ethylene glycol electrolyte forms nanotubes with surface composition of TiO2 and Ti(OH)4.19,21 Therefore, the features in the region 600–2300 cm−1 for the anodized sample are likely arising due to the presence of Ti–O at 980 and 770 cm−1 from surface vibrations, Ti–O–Ti stretch at 820 cm−1, and Ti–OH at 1210 cm−1.48,50–52 There is also likelihood for the presence of anatase TiO2 at 870 cm−1, which has been observed previously under similar anodization parameters and conditions at 60 V.19,21,50 Some features of NH4+ at 1464 cm−1, and Ti–N at 1075 cm−1 are also observed, which are from remnants of electrolyte.19,53,54
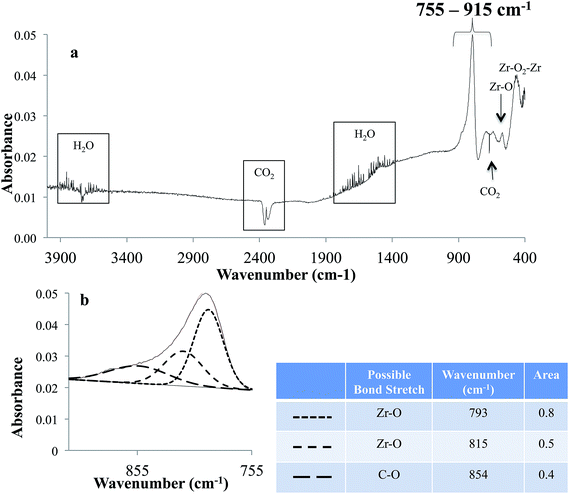 |
| Fig. 3 FTIR spectra of the ZrO2 sample: (a) whole spectrum (4000–400 cm−1) and (b) de-convolution of the region (755–915 cm−1). The legend in (b) provides the possible bond stretch assignments, peak position and area under the curves. | |
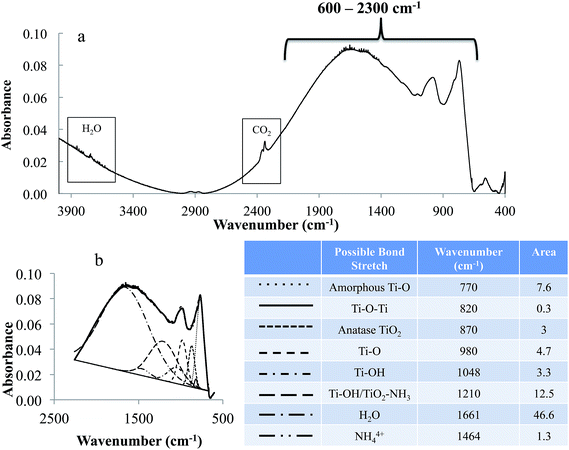 |
| Fig. 4 FTIR spectrum of anodized Ti–ZrO2 samples. (a) Whole spectrum (4000–400 cm−1) and (b) de-convolution of the region (600–2300 cm−1) from anodized Ti–ZrO2 samples. Legend in (b) provides the possible bonds stretch, peak position and area under the curves. Anodization condition is same as mentioned in Fig. 2. | |
It is likely that the de-convolution may include some deviation in the peak positions from literature values of the absorption bands due to (i) uncertainties from the sloped baseline due to irregular surface roughness, and (ii) absorption shift for a particular bond stretch due to the effect of other chemicals/molecular species present in the vicinity. Thus, study with controlled parameters is required to optimize the de-convolution of FTIRS data.
White light interferometry
White-light interferometry (WLI) was employed to probe the changes in surface roughness after each surface treatment on ZrO2 substrate. The average surface roughness and root mean square of the samples are shown in Table 1. A significant increase in surface roughness is observed after anodization compared to roughened ZrO2 samples. This is due to the increase in surface area owing to the formation of nanotubes on the Ti–ZrO2 substrate; additionally, a further increase in surface roughness is observed after 20 and 30 min of anodization compared to the samples anodized for 10 min (p < 0.05; Tukey HSD post hoc analysis). This increase in surface roughness is likely due to the increase in length and diameter of the nanotubes compared to 10TNZ samples. FESEM images of the samples corroborate the surface roughness results, which shows that TNTs length and diameter increases when anodization duration increases. Finally, the surface roughness of 20 and 30 min anodized samples show no statistically significant difference, which is corroborated by the FESEM results (shown in Fig. 2c and d). This similar length nanotubes for 20 and 30 min anodization is due to the limited availability of the titanium layer on ZrO2. Due to this limited titanium thickness, lengthening of the TNTs are hindered by the ZrO2 substrates; therefore, nanotubes length remains stable when anodization duration was increased from 20 to 30 min. Upon further increase in anodization duration to greater than 30 min, there is a possibility of shortening of nanotubes, as the etching of nanotubes walls will dominate over oxidation due to the ceramic substrate. This behavior is not investigated in this study.
Table 1 Quantitative comparison of average surface roughness and root mean square of the samples with different treatments; a: p < 0.05 compared to b and c; b: p < 0.05 compared to c
Surface topography |
Average roughness (Ra)/μm |
Root mean square (rms)/μm |
ZrO2 |
0.71 ± 0.13a |
0.91 ± 0.16a |
10 min anod. Ti–ZrO2 |
0.84 ± 0.04b |
1.04 ± 0.05b |
20 min anod. Ti–ZrO2 |
1.26 ± 0.04c |
1.46 ± 0.09c |
30 min anod. Ti–ZrO2 |
1.18 ± 0.04c |
1.42 ± 0.06c |
Water contact angle
Water contact angle (WCA) of ZrO2, and 10, 20 and 30 min anodized Ti–ZrO2 samples were monitored over a period of one month. Hydrophilic surfaces enhance osteoconduction, which improves the bone formation at the interface.55 High surface wettability is required for improved fibronectin and vitronectin adsorption on the surface that makes focal complexes, which allows cells to make strong actin mediated anchorage with the implant, thereby improving bone-implant contact.22,56,57
Fig. 5 shows the WCA results of each surface over a period of 27 days. WCA of ZrO2 samples on day 0 was hydrophobic compared to all other surfaces with WCA of 35.1 ± 5.6°, which increases to 59.8 ± 3.2° by day 27. Such WCA of ZrO2 is corroborated by González-Martín et al.58 Their study focused on investigating the surface free energy and wettability of zirconia based ceramics, which shows that the WCA of 5% yttria stabilized ZrO2 is about 72°.58 Akio Noro et al. also reported an increase in WCA of sandblasted ZrO2 samples to ∼80° after 21 days.59 This increase in WCA over time when samples are stored in air is due to carbon contamination from the environment, as shown by the X-ray photoelectron spectroscopy data with carbon content increasing from ∼20% (day 0) to ∼45% (day 21).59 After anodizing Ti on ZrO2, the surface becomes super-hydrophilic with WCA of <5°, 10.8 ± 1.8°, and 12.9 ± 1.9° for 10TNZ, 20TTNZ, and 30TTNZ, respectively. For day 0, the difference in surface wettability between 10TNZ and 20TTNZ or 30TTNZ samples is likely due to the difference in surface roughness. There is a decrease in wettability for 10TNZ (14.4 ± 2.4°) and 20TTNZ (20.6 ± 0.5°) samples by day 27 compared to their day 0 wettability; moreover, a non-significant decrease was observed for 30TTNZ samples with WCA of 16.1 ± 1.4°. This decrease in surface wettability for 10TNZ and 20TTNZ samples may likely be due to the carbon contamination during the aging period. However, compared to ZrO2, anodized Ti–ZrO2 samples are able to maintain their surface wettability to less than or about 20° over a period of 27 days. This anti-aging hydrophilic property of anodized titanium surface has been corroborated by our previous studies as well as in the literature.22–24 The ability of an anodized surface to maintain their surface wettability has been extensively discussed previously by Shin et al.21 They showed that when titanium is anodized in an electrolyte composed of EG, NH4F and water, the composition of nanotube walls is Ti(OH)4 and TiO2 that has the ability to attract more water molecules making the surface hydrophilic.21 The composition of nanotubes wall of Ti(OH)4 and TiO2 is also corroborated by the FTIR results, which was provided in the previous section.
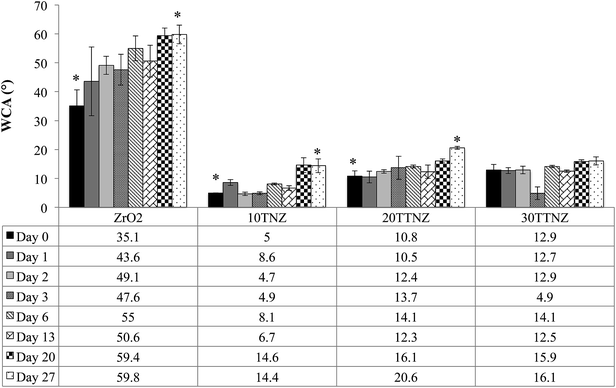 |
| Fig. 5 Surface wettability of ZrO2, 10TNZ, 20TTNZ and 30TTNZ samples over a period of one month. Wettability was measured every day for first three days followed by once every week over a period of one month. (*) Indicates a statistical significance within groups with p < 0.05. | |
The anti-aging property of TNTs is due to the surface composition of the nanotube walls, which contains Ti(OH)4 that has a higher affinity for water than for contaminants.19 Additionally, higher surface roughness of anodized Ti–ZrO2 along with Ti(OH)4 content, further improves its wettability, which can be explained by the Wenzel model. In the Wenzel model, if the WCA is between 0 < θ < 90°, then the higher the surface roughness, the higher the wettability.61
WCA of 10, 20 and 30 min anodized samples on day 0 shows that, 10TNZ samples were more hydrophilic with WCA of <5° compared to 20TTNZ and 30TTNZ with WCA of 10.8 ± 1.8° and 12.9 ± 1.9°, respectively. This difference in surface wettability between 10TNZ and 20TTNZ or 30TTNZ samples is likely due to the difference in surface roughness and presence of Ti(OH)4. WLI results show that the average surface roughness of 10TNZ samples is significantly smaller (p < 0.05: Tukey post hoc analysis) compared to 20TTNZ and 30TTNZ samples. Therefore, high initial WCA of 20 and 30 min anodized samples may be due to the higher probability of air entrapment inside the nanotubes in addition to lower Ti(OH)4 amount in TNTs walls due to conversion of Ti(OH)4 to TiO2 by condensation reaction of the hydrated oxide layer as the anodization duration increases.19,30 Dahotre et al. explain this higher WCA behavior of rougher samples using the Cassie and Baxter model.63 In their study, micro-textures of different roughness are created on Ti–6Al–4V alloy using laser-based optical interference and direct melting technique. They show that as the height of the grooves increases from 68.08 ± 0.02 nm to 208.08 ± 0.08 nm their WCA also increases from 70.7 ± 0.3° to 78.55 ± 0.45°. An increased volume of trapped air explains such increase in WCA on grooves with larger height compared to smaller height.63
Cell spreading, attachment and proliferation
Cell adhesion to the substrate is one of the determining factors for satisfactory bone–implant integration and cellular differentiation. After the initial cell attachment, they release extracellular matrix components which are collagenous and non-collagenous proteins. These proteins act as a scaffold for mineralization (calcium phosphate deposition) and thereby enhance bone formation and maturation.64–67 Higher cell spreading and attachment is essential for cell differentiation at the bone–implant interface. Studies have shown that when cells elongate, their cytoskeleton reorganizes and leads to changes in the conformation of nucleus, which triggers the cell differentiation process through DNA unfolding.68 Fig. 6 shows FESEM images of the cell shape and attachment morphology on each surface. Fig. 6a show the FESEM image of cells on roughened ZrO2 samples. Rounded cell morphology with many filopodia anchoring the surface is observed along with cell–cell interaction through filopodia. Similar cell bioactivity was observed on ZrO2 in the study performed by Depprich et al.12 They investigated the bone-to-implant contact after 1, 4, and 12 weeks of ZrO2 and titanium implants inside minipigs. Histological data showed that the bone-to-implant contact after 1 week on titanium was 47.3% ± 9.1 and for zirconia was 35.3% ± 10.8, which was not statistically significantly different (similar behavior was seen for weeks 4 and 12).12 Similarly, Pae et al. investigated the human gingival fibroblast (HGF) attachment on Ti and ZrO2 substrates. In their study, the degree of cell proliferation was measured after 24 and 48 h.69 They too found a non-statistical significant difference in HGF proliferation between Ti and ZrO2 samples.69
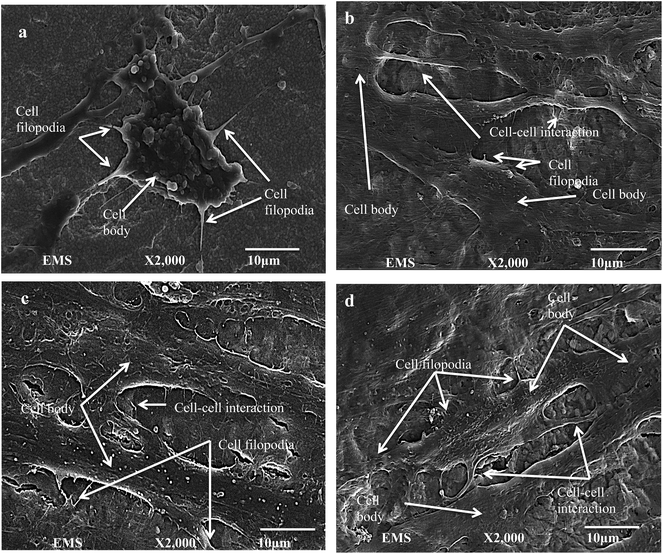 |
| Fig. 6 FESEM images of hMSCs cells after 6 days of cell seeding on (a) ZrO2, (b) 10TNZ, (c) 20TTNZ and (d) 30TTNZ. Cell body, filopodia, and cell–cell interaction are labeled with arrows. | |
On the other hand, well-elongated and well spread cells with filopodia and cell–cell interactions are observed on anodized Ti–ZrO2 samples compared to roughened ZrO2 samples as shown in Fig. 6b–d. Popat et al. observed similar behavior in their investigation regarding cell bioactivity on a flat Ti surface compared to an anodized Ti surface.64 A significant cell spreading and attachment was observed on the anodized surface compared to roughened ZrO2. This is due to the larger surface area and nanoscale features of anodized samples providing biomechanical cues to the cells, thereby allowing strong adhesion compared to on the flat Ti.60 Moreover, such nano-topographical features on the anodized surface causes higher biological cell plasticity triggering stem cell differentiation processes.68 Comparing the cell orientation and attachment morphology between 10, 20 and 30 min anodized samples, no significant difference is observed. This may likely be due to the similar surface composition of anodized surface with nanotube walls composed of TiO2 and Ti(OH)4. Additionally, non-significant difference in cell attachment between the nanotubes with 15–30 nm diameter is also seen by Park et al.70 They reported that nanotubes with diameters of 15–30 nm show higher amount of fibronectin and vitronectin adsorption on the surface resulting in an integrin mediated cell binding.70
Fig. 7 shows the fluorescence imaging of the cells after 3 and 24 h of seeding on the samples. The cell cytoskeleton was probed by staining the actin filaments using ActinRed 555 Readyprobe and the nucleus was probed using NucBlue stain that binds to the DNA of the cells. After 3 h of cell seeding, ZrO2 samples (Fig. 7a) show well-adhered cells though with rounded morphology that is likely due to the cells anchoring on all directions, which is also corroborated by the FESEM data (Fig. 6). However, on 10, 20 and 30 min anodized Ti–ZrO2 samples (Fig. 7c, e and g), cells show elongated morphology likely due to the hydrophilic nanotubular features of the anodized surface. Huang et al. also observed superior cell spreading on the anodized Ti–6Al–4V samples due to larger filopodia extension.71 They report that nanotubular features on the anodized surface interact with nano-scale cell membrane proteins (i.e., integrin) promoting integrin clustering on the cell membrane, which allows cells to make focal adhesion with the substrates.71 Cellular elongation is necessary for cell differentiation as mentioned above, through which the nucleus confirmation changes, which then transduces signals inside the nucleus to unfold the DNA and thereby triggers stem cell differentiation.68 After 24 h of cell culturing, significant increase in cell number as well as spreading is observed on all the substrates as shown in Fig. 7b, d, f, and h. From fluorescence microscopy, it is observed that the anodized surface allows cells to spread and elongate at the early period (after 3 h) of incubation, whereas roughened ZrO2 samples show elongated cell morphology only after 24 h of incubation. Such behavior of cells with well spreading and attachment observed on anodized surface may indicate its ability to provide bone-implant primary stability within 3 h as compared to 24 h. Fig. 8 shows the quantitative cell viability data of all the surfaces after 1 and 6 days of incubation. A significant (p < 0.05) increase in cells was observed on anodized Ti–ZrO2 samples compared to roughened ZrO2 after 6 days of incubation. No difference in cells was observed between 10TNZ and 20TTNZ samples either after 1 or 6 days of incubation. Likewise, no difference in cells was observed between 20TTNZ and 30TTNZ after 1 or 6 days of incubation. An increase in cells were observed from 1 to 6 days of incubation on all anodized Ti–ZrO2 samples compared with no difference on roughened ZrO2 sample from day 1 to day 6, which indicates that anodized Ti is biologically more active for enhancing cell response compared to the ZrO2 surface. In vivo studies are required in future, where Ti–ZrO2 samples with TiO2 nanotubes are implanted inside rat femur, to investigate the rate of osseointegration.
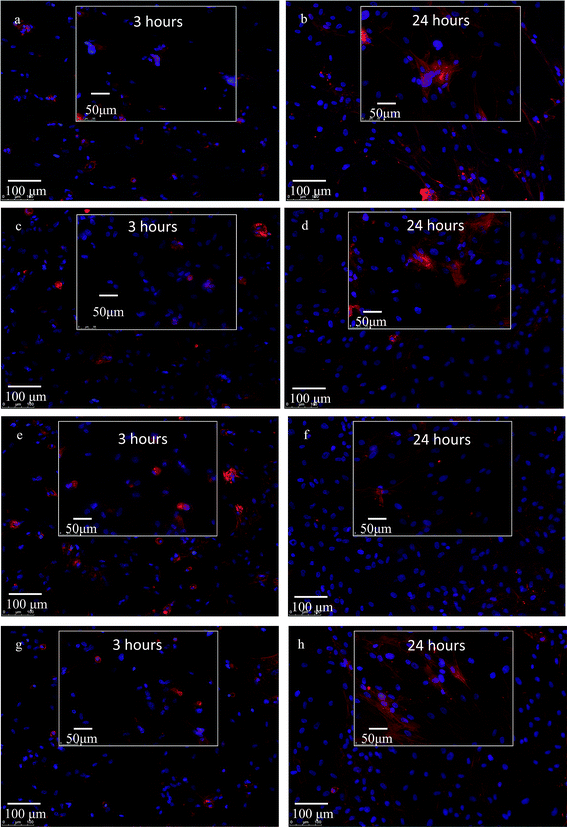 |
| Fig. 7 Fluorescence microscopy of hMSCs cells on ZrO2 (a and b), 10TNZ (c and d), 20TTNZ (e and f), and 30TTNZ (g and h) after 3 and 24 hours, respectively. Blue = nucleus; red = cytoskeleton. | |
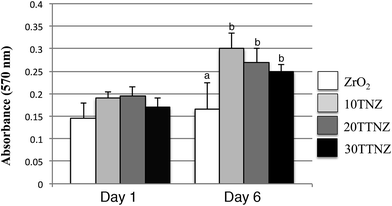 |
| Fig. 8 Cell viability test on ZrO2, 10TNZ, 20TTNZ and 30TTNZ (anodization conditions are indicated in Fig. 1) after 1 and 6 days of incubation in 5% CO2 and 37 °C. (a) p < 0.05 compared to group (b). | |
Conclusion
ZrO2 has been considered a potential alternative material for dental implants due to its tooth colored appearance. However, its bioactivity compared to titanium implants is still uncertain. Therefore, in this study the bioactivity of ZrO2 vs. different topographies of titanium deposited ZrO2 has been investigated. It was concluded that depositing titanium on ZrO2 substrate improves the bioactivity of the ZrO2 substrate in terms of enhanced cell viability, cell attachment and cell elongation. However, due to the gray colored appearance of Ti, the white colored appearance of ZrO2 is compromised. Therefore, the formation of titania nanotubes for 20 and 30 min in 2 vol% H2O, 0.25 wt% NH4F in EG at 60 V on the ZrO2 substrates was conducted. The achieved surface (TTNZ) improves cellular viability, cell adhesion, and cell elongation, and also maintains the white colored appearance of the ZrO2 substrate. Such cellular behavior is known to occur due to the favorable surface characteristics and roughness of TNT surfaces which is composed of TiO2 and Ti(OH)4 and is characterized by a significantly high surface area. The aforementioned factors not only provide high surface area for cellular attachment but also higher surface hydrophilicity can allow fibronectin and vitronectin attachment with the nanotubular surface, which in turn leads to integrin mediated elongated cell attachment. Additionally, it is important to note that significant improvement on the cell spreading was observed on the anodized surface without having to anneal the surface, which provides the added benefit of avoiding the annealing step. Future direction of this work will be to investigate the hMSCs differentiation on anodized Ti–ZrO2 substrates, to understand the mechanical stability of titania nanotubes on the ZrO2 substrate, and to perform the in vivo investigation in order to study the osseointegration rate.
Acknowledgements
The authors would like to thank Maria Runa for the surface roughness measurements performed in Dr Wimmers’ Tribology lab at Rush University Medical Center. This work made use of instrument in the Electron Microscopy Service (Research Resource Center, UIC). Financial support was provided by the Mechanical Engineering Department at MTU. We are grateful to National Science Foundation, DMR Grant # 1564950 and CBET Grant # 1067424, for making some of our characterizations possible.
References
- H. M. Grandin, S. Berner and M. Dard, A Review of Titanium Zirconium (TiZr) Alloys for Use in Endosseous Dental Implants, Materials, 2012, 5(12), 1348–1360 CrossRef CAS.
- Facts and Figures on Dental Implants, Dental Implants Facts and Figures 2014, cited 2014 August 28, http://www.aaid.com/about/press_room/dental_implants_faq.html.
- M. Özcan and C. Hämmerle, Titanium as a Reconstruction and Implant Material in Dentistry: Advantages and Pitfalls, Materials, 2012, 5(12), 1528–1545 CrossRef.
- D. Hagi, Biomechanical and Clinical Attributes of Zirconia Dental Implants – Two Case Reports, Oral Health Group, 2013, cited 2014 6th October, http://www.oralhealthgroup.com/news/biomechanical-and-clinical-attributes-of-zirconia-dental-implants-two-case-reports/1002516574/?%26er=NA Search PubMed.
- D. Little, Zirconia: Conservative Options in Metal-Free Dentistry, Dentsply Prosthetics, 2006, 5 Search PubMed.
- A.-E. Borgonovo, A. Fabbri, V. Vavassori, R. Censi and C. Maiorana, Multiple teeth replacement with endosseous one-piece yttrium-stabilized zirconia dental implants, Med. Oral Patol. Oral Cir. Bucal., 2012, 17(6), 7 Search PubMed.
- S. M. Zahnmed and P. A. Assal, The Osseointegration of Zirconia Dental Implants, Research and Science, 2013, 123, 12 Search PubMed.
- M. Goutam, G. Chandu, S. K. Mishra, M. Singh and B. Singh Tomar, Factors affecting Osseointegration: A Literature Review, J. Orofacial Research, 2013, 3(3), 5 Search PubMed.
- C. Yao, E. B. Slamovich and T. J. Webster, Enhanced osteoblast functions on anodized titanium with nanotube-like structures, J. Biomed. Mater. Res., Part A, 2008, 85A(1), 157–166 CrossRef CAS PubMed.
- M. Andreiotelli and R. J. Kohal, Fracture strength of zirconia implants after artificial aging, Clin. Implant Dent. Relat. Res., 2009, 11(2), 158–166 CrossRef PubMed.
- R. J. Kohal, D. Weng, M. Bächle and J. R. Strub, Loaded custom-made zirconia and titanium implants show similar osseointegration: an animal experiment, J. Periodontol., 2004, 75(9), 1262–1268 CrossRef PubMed.
- R. Depprich, H. Zipprich, M. Ommerborn, C. Naujoks, H. P. Wiesmann, S. Kiattavorncharoen, H. C. Lauer, U. Meyer, N. R. Kübler and J. Handschel, Osseointegration of zirconia implants compared with titanium: an in vivo study, Head Face Med., 2008, 4(1), 1–8 Search PubMed.
- M. Gahlert, S. Röhling, M. Wieland, C. M. Sprecher, H. Kniha and S. Milz, Osseointegration of zirconia and titanium dental implants: a histological and histomorphometrical study in the maxilla of pigs, Clin. Oral Implants Res., 2009, 20(11), 1247–1253 CrossRef CAS PubMed.
- M. Gahlert, T. Gudehus, S. Eichhorn, E. Steinhauser, H. Kniha and W. Erhardt, Biomechanical and histomorphometric comparison between zirconia implants with varying surface textures and a titanium implant in the maxilla of miniature pigs, Clin. Oral Implants Res., 2007, 18(5), 662–668 CrossRef CAS PubMed.
- S. Oh, C. Daraio, L. H. Chen, T. R. Pisanic, R. R. Fiñones and S. Jin, Significantly accelerated osteoblast cell growth on aligned TiO2 nanotubes, J. Biomed. Mater. Res., Part A, 2006, 78A(1), 97–103 CrossRef CAS PubMed.
- A. R. Elmore, Final report on the safety assessment of aluminum silicate, calcium silicate, magnesium aluminum silicate, magnesium silicate, magnesium trisilicate, sodium magnesium silicate, zirconium silicate, attapulgite, bentonite, Fuller's earth, hectorite, kaolin, lithium magnesium silicate, lithium magnesium sodium silicate, montmorillonite, pyrophyllite, and zeolite, Int. J. Toxicol., 2003, 22(1), 37–102 CrossRef PubMed.
- C. J. Frandsen, K. Noh, K. S. Brammer, G. Johnston and S. Jin, Hybrid micro/nano-topography of a TiO2 nanotube-coated commercial zirconia femoral knee implant promotes bone cell adhesion in vitro, Mater. Sci. Eng., C, 2013, 33(5), 2752–2756 CrossRef CAS PubMed.
- J. C. Grotberg, Modifying Ti6Al4V Implant Surfaces: Cell Responses and Corrosion Resistance of Annealed Titania Nanotubes, in Bioengineering. 2014, University of
Illinois at Chicago, p. 115 Search PubMed.
- S. B. Patel, A. Hamlekhan, D. Royhman, A. Butt, J. Yuan, T. Shokuhfar, C. Sukotjo, M. T. Mathew, G. Jursich and C. G. Takoudis, Enhancing surface characteristics of Ti–6Al–4V for bio-implants using integrated anodization and thermal oxidation, J. Mater. Chem. B, 2014, 2(23), 3597–3608 RSC.
- A. Hamlekhan, A. Butt, S. Patel, D. Royhman, C. Takoudis, C. Sukotjo, J. Yuan, G. Jursich, M. T. Mathew, W. Hendrickson, A. Virdi and T. Shokuhfar, Fabrication of Anti-Aging TiO2 Nanotubes on Biomedical Ti Alloys, PLoS One, 2014, 9(5), e96213 Search PubMed.
- D. H. Shin, T. Shokuhfar, C. Kyoung Choi, S.-H. Lee and C. Friedrich, Wettability changes of TiO2 nanotube surfaces, Nanotechnology, 2011, 22(31), 315704 CrossRef PubMed.
- D. M. Rivera-Chacon, M. Alvarado-Velez, C. Y. Acevedo-Morantes, S. P. Singh, E. Gultepe, D. Nagesha, S. Sridhar and J. E. Ramirez-Vick, Fibronectin and vitronectin promote human fetal osteoblast cell attachment and proliferation on nanoporous titanium surfaces, J. Biomed. Nanotechnol., 2013, 9(6), 1092 CrossRef CAS PubMed.
- X. Zhu, J. Chen, L. Scheideler, R. Reichl and J. Geis-Gerstorfer, Effects of topography and composition of titanium surface oxides on osteoblast responses, Biomaterials, 2004, 25(18), 4087–4103 CrossRef CAS PubMed.
- C. Strobel, N. Bormann, A. Kadow-Romacker, G. Schmidmaier and B. Wildemann, Sequential release kinetics of two (gentamicin and BMP-2) or three (gentamicin, IGF-I and BMP-2) substances from a one-component polymeric coating on implants, J. Controlled Release, 2011, 156(1), 37–45 CrossRef CAS PubMed.
- B. G. X. Zhang, D. E. Myers, G. G. Wallace, M. Brandt and P. F. Choong, Bioactive Coatings for Orthopaedic Implants—Recent Trends in Development of Implant Coatings, Int. J. Mol. Sci., 2014, 15(7), 11878–11921 CrossRef PubMed.
- J. Park, S. Bauer, A. Pittrof, M. S. Killian, P. Schmuki and K. von der Mark, Synergistic Control of Mesenchymal Stem Cell Differentiation by Nanoscale Surface Geometry and Immobilized Growth Factors on TiO2 Nanotubes, Small, 2012, 8(1), 98–107 CrossRef CAS PubMed.
- K. C. Popat, M. Eltgroth, T. J. LaTempa, C. A. Grimes and T. A. Desai, Titania Nanotubes: A Novel Platform for Drug-Eluting Coatings for Medical Implants?, Small, 2007, 3(11), 1878–1881 CrossRef CAS PubMed.
- T. Shokuhfar, S. Sinha-Ray, C. Sukotjo and A. L. Yarin, Intercalation of anti-inflammatory drug molecules within TiO2 nanotubes, RSC Adv., 2013, 3(38), 17380–17386 RSC.
- M. S. Aw, K. Khalid, K. Gulati, G. J. Atkins, P. Pivonka, D. M. Findlay and D. Losic, Characterization of drug-release kinetics in trabecular bone from titania nanotube implants, Int. J. Nanomed., 2012, 7, 10 Search PubMed.
- D. Regonini, C. R. Bowen, A. Jaroenworaluck and R. Stevens, A review of growth mechanism, structure and crystallinity of anodized TiO2 nanotubes, Mater. Sci. Eng., R, 2013, 74(12), 377–406 CrossRef.
- C. A. Grimes and G. K. Mor, TiO2 nanotube array, Springer Science+Business Media, New York, 2009, p. 380 Search PubMed.
- H. Yin, H. Liu and W. Z. Shen, The large diameter and fast growth of self-organized TiO2 nanotube arrays achieved via electrochemical anodization, Nanotechnology, 2010, 21, 8 Search PubMed.
- S. Berger, P. Roy and P. Schmuki, TiO2 Nanotubes: Synthesis and Applications, Angew. Chem., Int. Ed., 2011, 50, 36 Search PubMed.
- B. Chen, J. Hou and K. Lu, Formation Mechanism of TiO2 Nanotubes and Their Applications in Photoelectrochemical Water Splitting and Supercapacitors, Langmuir, 2013, 29(19), 5911–5919 CrossRef CAS PubMed.
- K. R. Hebert, S. P. Albu, I. Paramasivam and P. Schmuki, Morphological instability leading to formation of porous anodic oxide films, Nat. Mater., 2011, 11(2), 162–166 CrossRef PubMed.
- S. J. Garcia-Vergara, P. Skeldon, G. E. Thompson and H. Habazaki, A flow model of porous anodic film growth on aluminium, Electrochim. Acta, 2006, 52(2), 681–687 CrossRef CAS.
- P. Skeldon, G. E. Thompson, S. J. Garcia-Vergara, L. Iglesias-Rubianes and C. E. Blanco-Pinzon, A Tracer Study of Porous Anodic Alumina, Electrochem. Solid-State Lett., 2006, 9(11), 5 CrossRef.
- A. Z. Sadek, H. Zheng, K. Latham, W. Wlodarski and K. Kalantar-Zadeh, Anodization of Ti Thin Film Deposited on ITO, Langmuir, 2009, 25(1), 509–514 CrossRef CAS PubMed.
- T. Yu-Xin, T. Jie, Z. Yan-Yan, W. Tao, T. Hai-Jun and Z. Ya-Rong, Preparation of TiO2 nanotube on glass by anodization of Ti films at room temperature, Trans. Nonferrous Met. Soc. China, 2009, 19, 7 Search PubMed.
- J. Tupala, M. Kemell, E. Härkönen, M. Ritala and M. Leskelä, Preparation of regularly structured nanotubular TiO2 thin films on ITO and their modification with thin ALD-grown layers, Nanotechnology, 2012, 23(12), 125707 CrossRef PubMed.
- D. W. Liu, C. H. Perry and R. P. Ingel, Infrared spectra in nonstoichiometric yttria-stabilized zirconia mixed crystals at elevated temperatures, J. Appl. Phys., 1988, 64(3), 1413–1417 CrossRef CAS.
- H. L. Ningyuan Duan, L. Li, J. Hu, L. Bi, H. Lu, X. Weng, J. Xie and L. Deng, ZrO2-TiO2 thin films: a new material system for mid-infrared integrated photonics, Opt. Mater. Express, 2013, 3(9), 9 Search PubMed.
- J. Judes and V. Kamaraj, Preparation and characterization of yttria stabilized zirconia minispheres by the sol–gel drop generation method, Mater. Sci., 2009, 27(2), 9 Search PubMed.
- P. Ganesh babu, C. Kumar, K. Ravichandran and P. Manohar, Synthesis and Characterization of Zirconium Tin Titanate (Zr0.8Sn0.2TiO4), Int. J. ChemTech Res., 2013, 5(5), 8 Search PubMed.
- S. K. Sharma and S. Som, Eu3+/Tb3+-codoped Y2O3 nanophosphors: Rietveld refinement, bandgap and photoluminescence optimization, J. Phys. D: Appl. Phys., 2012, 45(415102), 12 Search PubMed.
- E.-M. Köck, M. Kogler, T. Bielz, B. Klötzer and S. Penner, In Situ FT-IR Spectroscopic Study of CO2 and CO Adsorption on Y2O3, ZrO2, and Yttria-Stabilized ZrO2, J. Phys. Chem. C, 2013, 117(34), 17666–17673 Search PubMed.
- M.-C. Rosu, R.-C. Suciu, M. Mihet and I. Bratu, Physical–chemical characterization of titanium dioxide layers sensitized with the natural dyes carmine and morin, Mater. Sci. Semicond. Process., 2013, 16(6), 1551–1557 CrossRef CAS.
- T. Bezrodna, G. Puchkovska, V. Shymanovska, J. Baran and H. Ratajczak, IR-analysis of H-bonded H2O on the pure TiO2 surface, J. Mol. Struct., 2004, 700(1–3), 175–181 CrossRef CAS.
- D. C. L. Vasconcelos, Infrared Spectroscopy of Titania Sol–Gel Coatings on 316L Stainless Steel, Mater. Sci. Appl., 2011, 02(10), 1375–1382 CAS.
- D. Velten, V. Biehl, F. Aubertin, B. Valeske, W. Possart and J. Breme, Preparation of TiO2 layers on cp-Ti and Ti6Al4V by thermal and anodic oxidation and by sol–gel coating techniques and their characterization, J. Biomed. Mater. Res., 2002, 59(1), 18–28 CrossRef CAS PubMed.
- V. A. Zeitler and C. A. Brown, The Infrared Spectra of Some Ti–O–Si, Ti–O–Ti and Si–O–Si Compounds, J. Phys. Chem., 1957, 61(9), 1174–1177 CrossRef CAS.
- R.-J. Wu, Y.-L. Sun, C.-C. Lin, H.-W. Chen and M. Chavali, Composite of TiO2 nanowires and Nafion as humidity sensor material, Sens. Actuators, B, 2006, 115(1), 198–204 CrossRef CAS.
- K. Hadjiivanov, J. Lamotte and J.-C. Lavalley, FTIR Study of Low-Temperature CO Adsorption on Pure and Ammonia-Precovered TiO2 (Anatase), Langmuir, 1997, 13(13), 3374–3381 CrossRef CAS.
- H. U. Lee, S. C. Lee, S. Choi, B. Son, S. M. Lee, H. J. Kim and J. Lee, Efficient visible-light induced photocatalysis on nanoporous nitrogen-doped titanium dioxide catalysts, Chem. Eng. J., 2013, 228, 756–764 CrossRef CAS.
- G. Zhao, Z. Schwartz, M. Wieland, F. Rupp, J. Geis-Gerstorfer, D. L. Cochran and B. D. Boyan, High surface energy enhances cell response to titanium substrate microstructure, J. Biomed. Mater. Res., Part A, 2005, 74A(1), 49–58 CrossRef CAS PubMed.
- A. B. Novales Jr, S. L. de Souza, R. R. Martins de Barros, K. K. Yamashina Pereira, G. Iezzi and A. Piattelli, In fluence of Implant Surfaces on Osseointegration, Braz. Dent. J., 2010, 21(6), 11 Search PubMed.
- J. H. Park, C. E. Wasilewski, N. Almodovar, R. Olivares-Navarrete, B. D. Boyan, R. Tannenbaum and Z. Schwartz, The responses to surface wettability gradients induced by chitosan nanofilms on microtextured titanium mediated by specific integrin receptors, Biomaterials, 2012, 33(30), 7386–7393 CrossRef CAS PubMed.
- M. L. González-Martín, L. Labajos-Broncano, B. Jańczuk and J. M. Bruque, Wettability and surface free energy of zirconia ceramics and their constituents, J. Mater. Sci., 1999, 34(23), 5923–5926 CrossRef.
- A. Noro, M. Kaneko, I. Murata and M. Yoshinari, Influence of surface topography and surface physicochemistry on wettability of zirconia (tetragonal zirconia polycrystal), J. Biomed. Mater. Res., Part B, 2013, 101B(2), 355–363 CrossRef CAS PubMed.
- M. Taborelli, M. Jobin, P. François, P. Vaudaux, M. Tonetti, S. Szmukler-Moncler, J. P. Simpson and P. Descouts, Influence of surface treatments developed for oral implants on the physical and biological properties of titanium. (I) Surface characterization, Clin. Oral Implants Res., 1997, 8(3), 208–216 CAS.
- F. Rupp, L. Scheideler, N. Olshanska, M. de Wild, M. Wieland and J. Geis-Gerstorfer, Enhancing surface free energy and hydrophilicity through chemical modification of microstructured titanium implant surfaces, J. Biomed. Mater. Res., Part A, 2006, 76A(2), 323–334 CrossRef CAS PubMed.
- K. J. Kubiak, M. C. T. Wilson, T. G. Mathia and P. H. Carval, Wettability versus roughness of engineering surfaces, Wear, 2011, 271(3–4), 523–528 CrossRef CAS.
- N. B. Dahotre, S. R. Paital, A. N. Samant and C. Daniel, Wetting behaviour of laser synthetic surface microtextures on Ti–6Al–4V for bioapplication, 2010, vol. 368, pp. 1863–1889 Search PubMed.
- K. C. Popat, L. Leoni, C. A. Grimes and T. A. Desai, Influence of engineered titania nanotubular surfaces on bone cells, Biomaterials, 2007, 28(21), 3188–3197 CrossRef CAS PubMed.
- N. Wang, H. Li, W. Lü, J. Li, J. Wang, Z. Zhang and Y. Liu, Effects of TiO2 nanotubes with different diameters on gene expression and osseointegration of implants in minipigs, Biomaterials, 2011, 32(29), 6900–6911 CrossRef CAS PubMed.
- A. F. Mavrogenis, R. Dimitriou, J. Parvizi and G. C. Babis, Biology of Implant Osseointegration, J. Musculoskeletal Neuronal Interact., 2009, 9(2), 11 Search PubMed.
- T. Shokuhfar, A. Hamlekhan, J. Y. Chang, C. K. Choi, C. Sukotjo and C. Friedrich, Biophysical evaluation of cells on nanotubular surfaces: the effects of atomic ordering and chemistry, Int. J. Nanomed., 2014, 9, 3737–3748 Search PubMed.
- S. Oh, K. S. Brammer, Y. S. Julie Li, D. Teng, A. J. Engler, S. Chien and S. Jin, Stem cell fate dictated solely by altered nanotube dimension, Proc. Natl. Acad. Sci. U. S. A., 2009, 106(7), 2130–2135 CrossRef CAS PubMed.
- A. Pae, H. Lee, H. S. Kim, Y. D. Kwon and Y. H. Woo, Attachment and growth behaviour of human gingival fibroblasts on titanium and zirconia ceramic surfaces, Biomed. Mater., 2009, 4(2), 025005 CrossRef PubMed.
- J. Park, S. Bauer, K. von der Mark and P. Schmuki, Nanosize and Vitality:
TiO2 Nanotube Diameter Directs Cell Fate, Nano Lett., 2007, 7(6), 1686–1691 CrossRef CAS PubMed. - H.-H. Huang, C.-P. Wu, Y.-S. Sun, W.-E. Yang and T.-H. Lee, Surface nanotopography of an anodized Ti–6Al–7Nb alloy enhances cell growth, J. Alloys Compd., 2014, 615, S648–S654 CrossRef CAS.
|
This journal is © The Royal Society of Chemistry 2017 |