DOI:
10.1039/C7RA03208K
(Paper)
RSC Adv., 2017,
7, 27162-27169
Overcoming the blood–brain barrier for glioma-targeted therapy based on an interleukin-6 receptor-mediated micelle system†
Received
18th March 2017
, Accepted 13th May 2017
First published on 23rd May 2017
Abstract
Treatments of cancer in the central nervous system (CNS), such as glioma, require nano-drugs to efficiently cross the blood–brain barrier (BBB) and highly accumulate within tumor regions. In this work, a new interleukin-6 receptor-mediated micelle system, where a short peptide I6P8 was conjugated to biodegradable poly(ethylene glycol)–poly(lactic-co-glycolic acid) (PEG–PLGA), was prepared for cascade-targeting drug delivery to glioma. In vitro results showed that the I6P8-conjugated doxorubicin (DOX)-loaded micelle (I6P8-D-M) could significantly transport across the BBB and subsequently target the glioma cells, which was superior to results of scrambled peptide-modified counterparts. While in vivo results confirmed that this multifunctional I6P8-D-M could introduce the highest glioma apoptosis and longest survival of glioma-bearing mice as compared to other groups. These findings suggest that the I6P8-D-M micelle system is a promising cascade-targeting therapeutic agent, which can overcome the BBB for glioma-targeted therapy.
1. Introduction
Effective delivery of therapeutic agents to the target sites is a promising strategy for treatments of different diseases, especially refractory cancers. Targeting technologies, including enhanced permeation and retention (EPR) effect-based passive targeting and ligand-mediated active targeting, are used to guide the therapeutic cargos to specific regions for the targeting therapy.1,2 However, for the treatments of central nervous system (CNS) diseases, such as malignant brain tumors (glioma), the simple passive or active tumor-targeted strategies are inadequate in delivering therapeutic agents to the desired locations. On one hand, for glioma, even in the late stage, passive accumulation of nanoparticles is not as characteristic as that in other solid tumors.3,4 Drug delivery has been reported to be less efficient in cranial tumors than that in subcutaneous ones.3,4 Thus drug delivery systems with small sizes such as micelles have more potential for glioma therapy.5,6 On the other hand, the presence of blood–brain barrier (BBB), which is a unique physiological interface between the CNS and the systemic blood circulation, can strictly restrict the transmembrane transport of substances into the brain.7,8 To overcome the BBB, elaborate strategies, including penetration via intracranial injection, BBB disruption via chemical or physical approaches, and transportation via biological regulations, have been employed for brain drug delivery.7,9–12 Among them, traversing BBB mediated by biological molecules (proteins, peptides, etc.) has been recognized as the most prospective technology due to its high bioactivity, low invasion and good biosafety.7,13,14 Therefore, combination of biological molecule-mediated BBB traversing and ligand-mediated active targeting would favor the successful brain drug delivery. Nevertheless, the current prevalent strategies for this aim mainly focus on the mediation via dual agents (one biological molecule and one ligand), which consequently increase the complexity, decrease the stability and compromise the targeting ability.15–17 Single biological agent-mediated cascade-targeting strategy across the BBB and then accumulating within glioma may alleviate those drawbacks to dual mediation, which is thereupon subjected to the multiplicity of the biological agents. The main concern for this purpose is the common target in both BBB and glioma cells.
Recently, interleukin 6 receptor (IL-6R) has been reported to be overexpressed on glioma cells and also on the BBB, while almost not on healthy astrocytes.18 The natural ligand of IL-6R, IL-6, was firstly considered as the cascade-targeting ligand. Unfortunately, the interaction between IL-6R and IL-6 facilitates the glioma growth, as introduced by autocrine growth promotion and angiogenesis induction via activation of vascular epithelial growth factor A (VEGF-A).18 Thus, IL-6 can not be used as the targeting ligand for glioma drug delivery. Furthermore, blocking IL-6R could inhibit the IL-6R/IL-6 interaction and further inhibit the glioma growth,18,19 suggesting that IL-6R represents a potential target for both therapy and glioma-targeted delivery across the BBB.
By phage display, a short peptide ligand, LSLITRL, was reported to possess similar targeting function for selectively binding with IL-6R to mediate nanoparticles into glioma cells,19 inhibiting the tumor growth.18 More importantly, this peptide could also inhibit tumor growth by the competitive binding with IL-6R as an IL-6 antagonist.18–20 Therefore, an N-terminally cysteinized LSLITRL, I6P8 peptide, would be considered to be a significant cascade-targeting ligand for glioma-targeted drug delivery across the BBB, and at the same time a therapeutic agent against glioma growth.
In this work, an canonical polymeric drug carrier, biodegradable poly(ethylene glycol)–poly(lactic-co-glycolic acid) (PEG–PLGA) micelle, which has been proved to be an robust vehicle for long-term delivery of BBB impermeable therapeutic agents to tumor, was modified by I6P8 peptide, and then deliver hydrophobic anticancer drug doxorubicin (DOX) to overcome the BBB for glioma-targeted therapy. The constructed brain drug delivery system, systematically demonstrated in vitro and in vivo, was illustrated in Scheme 1.
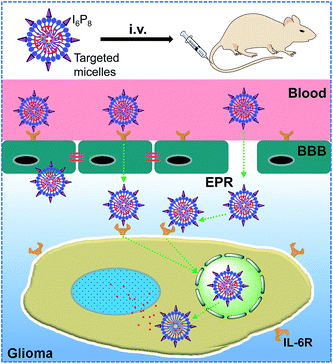 |
| Scheme 1 Transportation of targeted micelles after intravenously injected to glioma-bearing mice. | |
2. Experimental section
2.1. Materials
Mal–PEG5.5k-b-PLGA (75
:
25)2.2k and mPEG4k-b-PLGA (75
:
25)2.2k were purchased from Advanced Polymer Materials Inc. (Montreal, Canada). Targeting I6P8 peptide and scrambled I6P8 peptide (I6P8scr) were synthesized by Ziyu Biotech (Shanghai, China). DOX was bought from Huafeng United Tech (Beijing, China). TdT-mediated dUTP nick end labeling (TUNEL) apoptosis detection kit (FITC-labeled) was purchased from Keygen Biotech (Nanjing, China). Temozolomide (TMZ) was purchased from Meilun Biotech (Dalian, Chain). Trimethylamine (TEA), sodium fluorescein (NaF), indocyanine green (ICG), and other reagents, if not specified, were obtained from Sigma-Aldrich (MO, U.S.A.). All the chemicals were used without further purification.
Brain endothelial bEnd.3 cells were bought from ATCC (MD, U.S.A.). Human glioma U251 cells were purchased from Shanghai Cell Bank, Chinese Academy of Medical Sciences. U251 cells stably expressing green fluorescent proteins (GFP-U251 cells) were obtained from Genomeditech (Shanghai, China). Rat astrocytes (RA) cells were obtained from ScienCell Research Laboratories.
2.2. Synthesis and characterization of different polymers
I6P8 and its scrambled peptide were conjugated to Mal–PEG–PLGA based on the specific reaction of thiol group (–SH) in cysteine of peptides and maleimide group (–Mal) in the polymer. Peptides (1 mg) and Mal–PEG–PLGA (9.5 mg) were dissolved in PBS (pH 7.0) at the molar ration of 1.2
:
1 and stirred at room temperature overnight. Then the products were purified via dialysis against distilled water (MWCO = 10
000). The resulting peptide-conjugated polymers (I6P8–PEG–PLGA and I6P8scr–PEG–PLGA) were freeze-dried and stored for further usage.
To characterize the synthesis, different polymers were dissolved in deuterium chloroform and characterized using a 400 MHz nuclear magnetic resonance (NMR) spectrometer (Varian, USA).
2.3. Preparation of different micelles
Different DOX-loaded micelles were prepared using thin film rehydration method mainly as described previously.21 Briefly, 2 mg DOX was dispersed in 6 ml acetonitrile and excessive TEA (30 μl) was added to discard hydrochloric acid. And, different amount of I6P8–PEG–PLGA and mPEG–PLGA (the initial amount was 4 mg) with the molar percent of I6P8–PEG–PLGA ranged from 0% to 40% were mixed and dissolved in 4 ml acetonitrile. Then DOX was added at a molar ratio of 6
:
1 to total PEG–PLGA. After that the mixture was rotarily evaporated at 60 °C and then the obtained thin film was hydrated with saline. Unencapsulated DOX was removed via ultrafiltration and detected using a fluorescence spectrophotometer (Cary Eclipse, Agilent, U.S.A.).
2.4. Characterizations of micelles
Drug encapsulation efficiency (EE) and loading content (LC) were calculated as follows.
The sizes of different micelles were measured by a dynamic light scattering using a Nano-ZS instrument (Malvern, UK). Corresponding polydispersity was also recorded.
2.5. Cell culture
Human glioma U251 cells were cultured in special Dulbecco's modified Eagle medium (DMEM) supplemented with 10% fetal bovine serum (FBS), 1% L-glutamine, 1% penicillin and 1% streptomycin, at 37 °C and in 5% CO2 atmosphere. The GFP-U251 cells were cultured at the similar condition with U251 cells, except the addition of 15% FBS.
Brain capillary endothelial cells (bEnd.3) were expanded and routinely cultured in DMEM with the addition of 20% heat-inactivated FBS, 100 μg ml−1 epidermal cell growth factor (ECGF), 2 mM L-glutamine, 40 U ml−1 heparin, 100 U ml−1 penicillin, 100 μg ml−1 streptomycin, and maintained at 37 °C under a humidified atmosphere containing 5% CO2. RA cells were cultured under the same condition as bEnd.3.
2.6. Cellular uptake
To optimize the molar percent of I6P8–PEG–PLGA, confocal microscopy and intracellular DOX level were performed to qualify and quantify the cellular uptake levels, respectively. U251 cells were seeded in 96-well microplates at a density of 1 × 104 cells per well for 24 h. DOX-loaded micelles (calculated DOX concentration was 100 μg ml−1) with the molar percent of I6P8–PEG–PLGA ranged from 0% to 40% were incubated with U251 cells for 1 h. Then the cells were rinsed with PBS and fixed with 4% paraformaldehyde for 15 min, after which the medium was changed to PBS. Confocal microscopy was performed using a TCs SP5 microscope (Leica, Germany). For quantitative evaluation, U251 cells were seeded in 24-well plates at a density of 5 × 104 cells per well for 24 h, then incubated with DOX-loaded micelles (calculated DOX concentration was 100 μg ml−1) with the molar percent of I6P8–PEG–PLGA ranged from 0% to 40% for 1 h. After that, cells were rinsed with PBS three times, lysed with 1% triton (200 μl per well), and the protein level was determined using the Bio-Rad Protein Assay Kit.22 The intracellular DOX level was measured by a fluorescence spectrophotometer. And the calculated DOX concentrations to cell proteins were used for evaluation.
2.7. Transportation studies in BBB monolayers
In vitro BBB monolayers were constructed in a modified method according to our previous work.13 Briefly, bEnd.3 cells were seeded in 24-well transwell filters (Falcon Cell Culture Insert, Becton Dickinson Labware, NJ, U.S.A.) at a density of 6 × 104 cells per cm2. One week later, confluency was verified under a microscope, and the transendothelial electrical resistance (TEER) was measured to monitor the BBB monolayer integrity via an epithelial voltohmmeter. Only BBB monolayers with TEER larger than 200 Ω cm2 were used for transportation studies. Furthermore, NaF with final concentration of 10 μg ml−1 was used as an indicator to monitor the BBB monolayer integrity during the entire experiment.
Different DOX-loaded micelles (100 μl) were added in the donor chamber of the transwells with the addition of NaF. The accepter chamber contained 900 μl Hank's solution. The wells were incubated at 37 °C under 50 rpm shaking condition. An aliquote of 300 μl sample was removed from the acceptor chamber and the same volume of fresh medium was added back immediately at 5, 10, 15, 30, 45 and 60 min. The transported amount of DOX was determined using a Tecan Infinite M1000 Pro microplate reader (Switzerland). The apparent permeability (Papp) was calculated as follows:13
where ∂
Q/∂
t represents the permeability rate (nmol s
−1),
C0 is the initial concentration (nmol ml
−1) in the donor chamber, and
A is the surface area (cm
2) of the filter membrane. The data of transport ratio of micelles were also presented.
2.8. Targeting evaluation in vitro
In vitro glioma-targeted efficiency of different micelles was evaluated using confocal microscopy. GFP-U251 cells were seeded in 24-well microplates at a density of 3 × 104 cells per well for 24 h. Then the medium was removed and healthy RA cells were added at a density of 1 × 104 cells per well. These two kinds of cells were co-cultured for another 24 h. Different DOX-loaded micelles were incubated with the co-cultured cells for 1 h. Then the cells were rinsed with PBS and fixed with 4% paraformaldehyde for 15 min, after which the medium was changed to PBS. Finally, the cells were examined under a confocal microscope.
2.9. Orthotopic animal models
Orthotopic glioma-bearing models were constructed using nude mice with the body weight between 20–24 g. Nude mice were purchased from Shanghai Laboratory Animal Center and maintained under standard conditions. Briefly, U251 cells (5 × 105) were slowly implanted into the right striatum (1.8 mm lateral to the bregma and 3 mm of depth) using a stereotactic fixation device with a mouse adaptor. After surgery, mice were further maintained under standard housing conditions. Animals were maintained in accordance with the guidelines of National Laboratory Animal Welfare and Ethics, China, and approved by the ethics committee of Fudan University.
2.10. Targeting evaluation in vivo
Tissue distribution was carried out to evaluate the targeting efficiency of micelles in vivo. The near infrared dye, ICG, was loaded to micelles to avoid the disturbance of autofluorescence. ICG-loaded micelles (250 μg calculated DOX per mouse) modified with normal or scrambled I6P8 peptide were intravenously administrated to the glioma-bearing mice. After 4 h, the mice were sacrificed and major organs (brain, heart, liver, spleen, lung and kidney) were excised. Fluorescent imaging analysis was performed using an In Vivo IVIS Spectrum Imaging System (PerkinElmer, U.S.A.).
2.11. Anti-glioma effects
TUNEL apoptosis detection and survival analysis were applied to evaluate the anti-glioma effects. At the 10th, 12th and 14th day after surgery described above, glioma-bearing mice were intravenously injected with saline (the negative control), free DOX (the positive control, 250 μg per mouse), I6P8scr-D-M and I6P8-D-M at the dose of 250 μg calculated DOX per mouse. Another positive control was TMZ-treated group, in which mice received TMZ via intragastric administration with a dose of 50 mg kg−1 at the 10th, 11th, 12th, 13th and 14th day after surgery. Two days later, two mice from each group were sacrificed and used for preparing frozen sections (10 μm). Slides were stained with DAPI and then examined using TUNEL apoptosis detection kit (FITC-labeled) according to the manufacturer's instruction. Finally, all the treated slides were observed under a fluorescent microscope. Ten mice from each group were continually monitored for survival.
2.12. Data analysis
Data were expressed as mean ± S.D. Statistical analysis was performed by two-tailed student's t-test using GraphPad InStat 3. Statistical significance was defined as p < 0.05. The survival data were analyzed by GraphPad Prism 5 using the Kaplan-Meier method and the log-rank test.
3. Results and discussion
3.1. Synthesis and characterization of polymers
The successful conjugation of the multifunctional I6P8 or the scrambled peptide (I6P8scr) to the classical PEG–PLGA polymer was based on the specific reaction between –SH group on peptides and –Mal group on PEG, which was consequently evidenced by the NMR results. As shown in Fig. S1,† the characteristic peak of –Mal around 6.7 ppm in the original Mal–PEG–PLGA micelles disappeared after conjugation with peptides, demonstrating the covalent bonding of I6P8 or I6P8scr with PEG–PLGA. Other peaks, indexed as the repeat units of PEG at 3.6 ppm and the typical methyl/methylene chemical shifts in lactide units (1.6 ppm & 5.2 ppm) and glycolide units (4.8 ppm) of PLGA, all reserved, which proved the no other special bonding and the stability of the multifunctional micelle polymers.
3.2. Characterizations of prepared micelles
According to the above procedure, different amounts of peptides were conjugated to PEG–PLGA to form I6P8–PEG–PLGA micelle. As shown in Table 1, all these micelles were around 24 nm with excellent polydispersity. Anti-cancer drug, DOX, could be encapsulated in these micelles. The encapsulation and loading efficiency slightly went up when increasing the amount of peptide ligands. This might be attributed to the increased hydrophilic segment in polymer, which can stabilize the micelles with even more hydrophobic segment (DOX) aggregated together via surface hydration layer, in good agreement with the results in Luo's lab.23 However, this effect reached a platform, indicating that proper amount of the peptide ligand might achieve suitable encapsulation and loading efficiency. Then, three different micelle formulations were characterized. The results showed that no apparent difference was observed in size, encapsulation and loading efficiency (Table 2). Furthermore, the cumulative DOX release from these three micelles was also similar (Fig. S2†), suggesting the parallel preparations of micelles.
Table 1 Characteristics of I6P8-D-M with different molar percent of I6P8–PEG–PLGA (mean ± S.D., n = 4)
Molar percent of I6P8–PEG–PLGA |
Micellar size (nm) |
Polydispersity |
EE (%) |
LC (%) |
2.5% |
23.08 ± 1.01 |
0.101 ± 0.02 |
89.2 ± 1.7 |
28.0 ± 0.8 |
5% |
24.11 ± 1.36 |
0.121 ± 0.02 |
90.1 ± 1.3 |
30.1 ± 0.6 |
10% |
23.55 ± 1.47 |
0.075 ± 0.01 |
91.0 ± 2.3 |
30.3 ± 0.9 |
20% |
23.89 ± 1.08 |
0.162 ± 0.09 |
91.7 ± 1.9 |
31.0 ± 0.7 |
40% |
25.21 ± 1.91 |
0.176 ± 0.11 |
90.1 ± 1.6 |
30.2 ± 0.9 |
Table 2 Characteristics of different DOX-loaded micelles (5% molar percent of peptide in modified micelles) (mean ± S.D., n = 4)
Polymeric micelles |
Micellar size (nm) |
Polydispersity |
EE (%) |
LC (%) |
D-M |
23.16 ± 0.95 |
0.090 ± 0.01 |
88.9 ± 1.6 |
28.8 ± 0.6 |
I6P8scr-D-M |
24.53 ± 1.52 |
0.108 ± 0.02 |
90.7 ± 2.7 |
30.6 ± 0.9 |
I6P8-D-M |
24.11 ± 1.36 |
0.121 ± 0.02 |
90.1 ± 1.3 |
30.1 ± 0.6 |
3.3. Cellular uptake
The optimal amount of I6P8 peptide conjugated to PEG–PLGA was screened via cellular uptake qualified by confocal microscopy and quantified by intracellular DOX level. As shown in Fig. 1A–L, the cellular uptake of I6P8-D-M increased with the molar percent of I6P8–PEG–PLGA and then reached a platform. When the molar percent was 5%, the cells showed strongest DOX signal. This was verified by the quantified result where the 5% molar percent of I6P8 mediated the highest cellular uptake of I6P8-D-M compared to other groups ranged from 0–40% (Fig. 1M). It has been reported that optimal modification extent of targeting ligands might introduce maximum cellular uptake.21 In this work, the optimal molar percent of I6P8 in the micelles was 5%, which was therefore fixed for the preparation of I6P8-D-M in the following experiments.
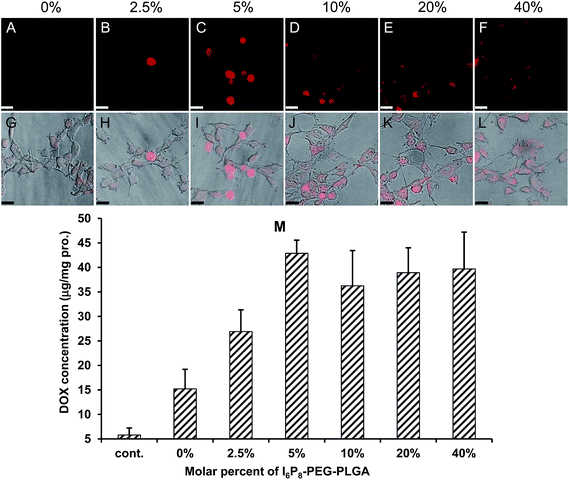 |
| Fig. 1 Confocal microscopy images (A–L) and intracellular DOX level (M) of I6P8-D-M with different molar percent of I6P8–PEG–PLGA. The micelles were incubated with U251 glioma cells for 1 h. Red: DOX. Bar = 25 μm. Data were represented as mean ± S.D. (n = 4). | |
3.4. Transportation across the BBB
The BBB-crossing ability of different micelles was evaluated in in vitro BBB monolayers. With the modification of I6P8 peptide, the significantly enhanced permeability and transport ratio of micelles were observed from the comparison results (Fig. 2). The apparent permeability of I6P8-D-M was 13 × 10−6 cm s−1 after 1 h incubation, while those of I6P8scr-D-M and D-M were only 4.26 × 10−6 cm s−1 and 3.82 × 10−6 cm s−1, respectively. No apparent difference in BBB transportation was observed between the two control groups. These results verified the IL-6 receptor-mediated transportation, and also I6P8 peptide was an important segment in mediating micelles across the BBB.
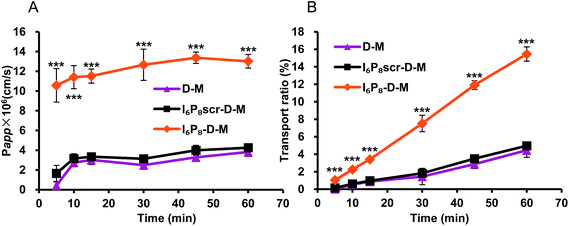 |
| Fig. 2 The apparent permeability and transport ratio of different micelles across the in vitro BBB monolayers. Data were represented as mean ± S.D. (n = 4). Significance: ***, p < 0.001. | |
3.5. Glioma-targeted efficiency
Glioma-targeted efficiency of different drug-loaded micelles was determined in the co-culture model of GFP-U251 glioma cells and RA normal brain cells. In I6P8-D-M-treated wells, much stronger DOX signal was observed in U251 cells compared to that in RA cells (Fig. 3K–O). In contrast, in D-M and I6P8scr-D-M-treated wells, all the DOX signals were weak and had no apparent difference between U251 and RA cells (Fig. 3A–J). This demonstrated the efficient targeting ability of I6P8-D-M to glioma cells. In view of the no distinguished difference between D-M and I6P8scr-D-M in BBB-crossing and glioma-targeted efficiency, only I6P8scr-D-M was applied as the control in the following studies.
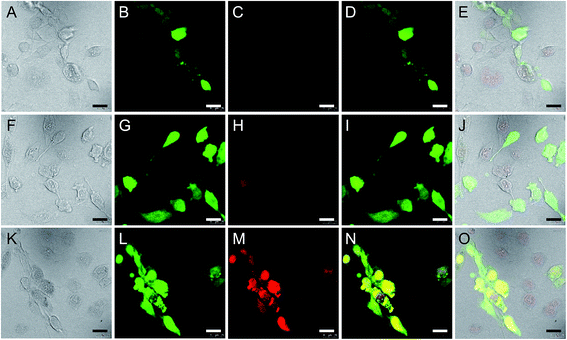 |
| Fig. 3 In vitro glioma-targeted efficiency of D-M (A–E), I6P8scr-D-M (F–J) and I6P8-D-M (K–O). The micelles were incubated with the co-culture model of GFP-U251 glioma cells and RA normal cells for 1 h. Red: DOX. Green: GFP-labled U251 cells. Bar = 25 μm. | |
3.6. In vivo targeting evaluation
The in vivo targeting ability of micelles was studied by the fluorescence imaging of mice intravenously injected with ICG-loaded micelles, which could achieve strong and accurate signals without disturbance of animal autofluorescence. As shown in Fig. 4A, the I6P8-modified micelle mediated much more intensive accumulation within glioma area, compared to that of I6P8scr-D-M. Interestingly, apparent accumulation of I6P8-D-M was observed around the central glioma area, which might be the glioma stem cells. It has been reported that IL-6 signal pathway is important not only in glioma cells but also in glioma stem cells.20,24 Thus this work further indicated the desirable accumulation of I6P8-D-M within both glioma cells and glioma stem cells, which can therefore be exploited as brain drug delivery systems for recurrent glioma therapy. Moreover, I6P8-D-M treated mouse exhibited less liver fluorescence than the I6P8scr-D-M treated one (Fig. 4B), revealing the relatively low nonspecific retention of I6P8-D-M in vivo. Interestingly, large amount of accumulation within liver and kidney was observed for both micelles, indicating the possible excretion by these two organs (Fig. 4B). However, this exists inconsistency compared to a previous study where more micelles were found in the liver and spleen,21 the reason of which should be further explored in the future study.
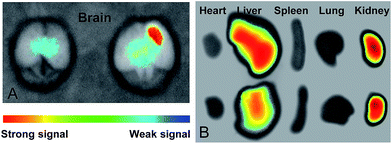 |
| Fig. 4 Fluorescent images of main organs of glioma-bearing nude mice treated with ICG-loaded I6P8scr-modified micelle (left in A and up in B) and I6P8-modified micelle (right in A and down in B). The organs were excised 4 h after treatments. The fluorescence signal of near-infrared dye ICG was recorded. | |
3.7. Glioma apoptosis
In vivo anti-glioma effect of different formulations was firstly evaluated using TUNEL apoptosis detection kit, with free DOX and commercial TMZ as positive controls, and saline as the negative control. As shown in Fig. 5, owing to the only EPR effect of DOX-loaded formulations, the glioma apoptosis of I6P8scr-D-M treated mouse was just a little more than that of free DOX group. The I6P8-D-M treated mouse exhibited highest glioma apoptosis among all the groups, even higher than the commercial TMZ treated group. This evidenced the strongly targeting lethal effect of I6P8-D-M for glioma therapy.
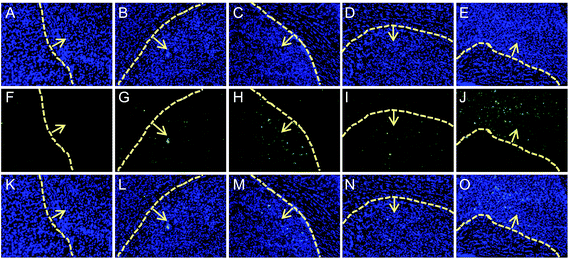 |
| Fig. 5 Apoptosis of glioma-bearing mice treated with saline (A, F and K), free DOX (B, G and L), TMZ (C, H and M), I6P8scr-D-M (D, I and N) and I6P8-D-M (E, J and O). Slides were subjected to TUNEL apoptosis detection kit. Yellow dotted lines marked the borders of glioma and arrows pointed to the inner sides of glioma. The third panel images were merged images of corresponding first and second panels. Blue: DAPI; green: FITC-indicated apoptosis. | |
3.8. Survival analysis
In vivo anti-glioma effect was secondly verified via the survival profile. As shown in Fig. 6, an apparently prolonged survival time was observed in I6P8-D-M treated mice (49.5 days) as compared to those treated with saline (31 days), free DOX (33.5 days), TMZ (44 days) and I6P8scr-D-M (36.5 days), respectively. Besides the chemotherapeutic effect, this might be attributed to (1) I6P8 peptide mediated the significantly enhanced BBB-crossing efficiency (Fig. 2) and glioma-targeted accumulation (Fig. 3 and 4), and (2) I6P8 peptide mediated the tumor growth inhibition by blocking the inimical interaction between IL-6 and IL-6R.18,19 All the results demonstrated that multifunctional I6P8 peptide-linked classical PEG–PLGA micelle is a highly efficient glioma-targeted therapeutic platform.
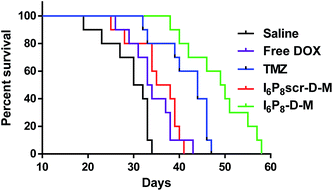 |
| Fig. 6 The survival profile of glioma-bearing mice with different treatments. | |
4. Conclusions
In summary, a multifunctional I6P8 peptide-conjugated classical PEG–PLGA micelle, was prepared and developed as a drug vehicle for targeting delivery of hydrophobic DOX to glioma. The I6P8 peptide-linked micelle could significantly enhance the BBB-transporting efficiency and glioma-targeted accumulation. When encapsulating DOX, I6P8-D-M introduced highest glioma tissue apoptosis and longest survival of glioma-bearing mice, compared to other groups. Overcoming the BBB for glioma-targeted therapy accomplished by the IL-6R-mediated micelle, together with the potential tumor growth inhibition effect of I6P8 peptide, is therefore expected to be a promising alternation for brain cancer treatment.
Acknowledgements
This study was supported by the grants from National Natural Science Foundation of China (21675032) and Nantong Municipal Science and Technology People's Livelihood Demonstration Project (MS32015023).
References
- N. Schleich, C. Po, D. Jacobs, B. Ucakar, B. Gallez, F. Danhier and V. Preat, J. Controlled Release, 2014, 194, 82–91 CrossRef CAS PubMed.
- E. J. Chung, Y. Cheng, R. Morshed, K. Nord, Y. Han, M. L. Wegscheid, B. Auffinger, D. A. Wainwright, M. S. Lesniak and M. V. Tirrell, Biomaterials, 2014, 35, 1249–1256 CrossRef CAS PubMed.
- Y. Liu and W. Lu, Expert Opin. Drug Delivery, 2012, 9, 671–686 CrossRef CAS PubMed.
- A. Gutkin, Z. R. Cohen and D. Peer, Expert Opin. Drug Delivery, 2016, 13, 1573–1582 CrossRef CAS PubMed.
- Y. Huang, W. Liu, F. Gao, X. Fang and Y. Chen, Int. J. Nanomed., 2016, 11, 1629–1641 CAS.
- X. Chen, L. Tai, J. Gao, J. Qian, M. Zhang, B. Li, C. Xie, L. Lu, W. Lu and W. Lu, J. Controlled Release, 2015, 218, 29–35 CrossRef CAS PubMed.
- B. Oller-Salvia, M. Sanchez-Navarro, E. Giralt and M. Teixido, Chem. Soc. Rev., 2016, 45, 4690–4707 RSC.
- A. Jain, A. Jain, N. K. Garg, R. K. Tyagi, B. Singh, O. P. Katare, T. J. Webster and V. Soni, Acta Biomater., 2015, 24, 140–151 CrossRef CAS PubMed.
- Y. Z. Zhao, L. J. Chen, Q. Lin, J. Cai, W. Z. Yu, Y. P. Zhao, C. Y. Xu, K. L. Mao, F. R. Tian, W. F. Li, H. L. Wong and C. T. Lu, Oncotarget, 2015 DOI:10.18632/oncotarget.5144.
- Y. Z. Zhao, Q. Lin, H. L. Wong, X. T. Shen, W. Yang, H. L. Xu, K. L. Mao, F. R. Tian, J. J. Yang, J. Xu, J. Xiao and C. T. Lu, J. Controlled Release, 2016, 224, 112–125 CrossRef CAS PubMed.
- R. K. Oberoi, K. E. Parrish, T. T. Sio, R. K. Mittapalli, W. F. Elmquist and J. N. Sarkaria, Neuro-Oncology, 2016, 18, 27–36 CrossRef PubMed.
- C. H. Fan, C. Y. Ting, Y. C. Chang, K. C. Wei, H. L. Liu and C. K. Yeh, Acta Biomater., 2015, 15, 89–101 CrossRef CAS PubMed.
- H. Yao, K. Wang, Y. Wang, S. Wang, J. Li, J. Lou, L. Ye, X. Yan, W. Lu and R. Huang, Biomaterials, 2015, 37, 345–352 CrossRef CAS PubMed.
- T. Yin, L. Yang, Y. Liu, X. Zhou, J. Sun and J. Liu, Acta Biomater., 2015, 25, 172–183 CrossRef CAS PubMed.
- P. J. Yue, L. He, S. W. Qiu, Y. Li, Y. J. Liao, X. P. Li, D. Xie and Y. Peng, Mol. Cancer, 2014, 13, 191 CrossRef PubMed.
- X. Wei, J. Gao, C. Zhan, C. Xie, Z. Chai, D. Ran, M. Ying, P. Zheng and W. Lu, J. Controlled Release, 2015, 218, 13–21 CrossRef CAS PubMed.
- T. Zong, L. Mei, H. Gao, K. Shi, J. Chen, Y. Wang, Q. Zhang, Y. Yang and Q. He, J. Pharm. Sci., 2014, 103, 3891–3901 CrossRef CAS PubMed.
- A. Sturzu and S. Heckl, Chem. Biol. Drug Des., 2010, 75, 369–374 CAS.
- J. L. Su, K. P. Lai, C. A. Chen, C. Y. Yang, P. S. Chen, C. C. Chang, C. H. Chou, C. L. Hu, M. L. Kuo, C. Y. Hsieh and L. H. Wei, Cancer Res., 2005, 65, 4827–4835 CrossRef CAS PubMed.
- H. Wang, J. D. Lathia, Q. Wu, J. Wang, Z. Li, J. M. Heddleston, C. E. Eyler, J. Elderbroom, J. Gallagher, J. Schuschu, J. MacSwords, Y. Cao, R. E. McLendon, X. F. Wang, A. B. Hjelmeland and J. N. Rich, Stem Cells, 2009, 27, 2393–2404 CrossRef CAS PubMed.
- Y. Meng, S. Wang, C. Li, M. Qian, Y. Zheng, X. Yan and R. Huang, Int. J. Pharm., 2016, 498, 40–48 CrossRef CAS PubMed.
- R. Q. Huang, Y. H. Qu, W. L. Ke, J. H. Zhu, Y. Y. Pei and C. Jiang, FASEB J., 2007, 21, 1117–1125 CrossRef CAS PubMed.
- F. Cheng, X. Guan, H. Cao, T. Su, J. Cao, Y. Chen, M. Cai, B. He, Z. Gu and X. Luo, Int. J. Pharm., 2015, 492, 152–160 CrossRef CAS PubMed.
- A. Hossain, J. Gumin, F. Gao, J. Figueroa, N. Shinojima, T. Takezaki, W. Priebe, D. Villarreal, S. G. Kang, C. Joyce, E. Sulman, Q. Wang, F. C. Marini, M. Andreeff, H. Colman and F. F. Lang, Stem Cells, 2015, 33, 2400–2415 CrossRef CAS PubMed.
Footnote |
† Electronic supplementary information (ESI) available. See DOI: 10.1039/c7ra03208k |
|
This journal is © The Royal Society of Chemistry 2017 |
Click here to see how this site uses Cookies. View our privacy policy here.