DOI:
10.1039/C7RA02286G
(Paper)
RSC Adv., 2017,
7, 26532-26536
Sequestration of orange G and methylene blue from aqueous solutions using a Co(II) coordination polymer†
Received
23rd February 2017
, Accepted 9th May 2017
First published on 17th May 2017
Abstract
A Co(II) coordination polymer known for its crystalline sponge properties (CoSP) is used to sequester Orange G (OG) and methylene blue (MB) in aqueous solutions. Its ability to encapsulate the cationic MB is much greater than for OG. The CoSP material is notable for its structural transformations under different conditions (i.e. removal from solvent or immersion in alternative solvent systems) giving rise to both 3D and 2D networks. Herein, we study the activated semi-amorphous Co sponge (a-CoSP) as a host in aqueous solutions and find that guest uptake results in increased crystallinity as evidenced by powder X-ray diffraction (PXRD). Reactivation releases some dye due to decomposition of the coordination polymer however, the solubility of the building blocks in solution enables the reconstruction of the sponge.
Introduction
Organic dyes are a class of pollutants that are commonly found in industrial wastewater due to their wide application as colorants for textiles, papers, food, and as imaging agents.1 There are more than 100
000 commercially available organic dyes of various sizes, colors, and ionicity that are being produced annually.2 Aside from their toxicities and carcinogenicities, these colored effluents may interfere with the absorption and reflection of light in water, thereby affecting biological processes in natural systems.3 Many dyes are stable and non-biodegradable in water. As a consequence, strict environmental regulations are currently imposed by national and international agencies for the manufacture and use of these organic dyes.4
The most common methods for the removal of organic dyes in wastewater is through physical, chemical, and/or biological methods.5,6 Each method has its own advantages and disadvantages; however, physical removal via adsorption is generally considered as the most rapid way to reduce dye concentration in water.4 Nanomaterials have been extensively studied, not only as adsorbents for the removal of organic dyes in water, but also as photo-catalysts for degradation.7–11 These materials are very efficient in organic dye removal and degradation but suffer from agglomeration and post-separation difficulties.2
Over the past two decades metal–organic frameworks (MOFs) have attracted attention for their application in the adsorption and photo-catalytic degradation of organic dyes.2,12 One of the practical challenges in the use of MOFs as adsorbents for organic dyes is their water instability.13 Several studies have demonstrated that frameworks with N-atom donors are more water stable than those with O-atom donors, such as carboxylates.14,15
With this in mind, we hypothesized that the [(Co(NCS)2)3(TPT)4]·25(o-C6H4Cl2)·5(MeOH) (TPT = 2,4,6-tris(4-pyridyl)-1,3,5-triazine) “cobalt sponge” coordination polymer (CoSP) previously reported by Fujita and co-workers16 may serve as a stable host for the absorption of organic dyes in water. The host/guest chemistry of this material has been demonstrated using a variety of guests including tetrathiafulvalene,16 fullerenes,16 titanocene,16 aldehydes,17 and cyclohexanone.18 This ability of MOFs to accommodate guests in crystalline form was known as the crystalline sponge method. The structure of this Co sponge (Fig. 1a) was initially solved in the cubic space group Fm
m with octahedral Co(II) centres with two trans NCS ligands and four pyridyl donors originating from four unique TPT ligands. Murugesu and co-workers recently reported microcrystals of CoSP belonging to the trigonal space group R3m with the same overall structure as the framework reported by Fujita and co-workers, with different solvents of crystallization.19 It was observed that the orange crystals (i-CoSP; inactive form) transformed to a green semi-amorphous material (a-CoSP; activated form) upon removal from the mother liquor, attributed to the removal of solvent and loss of crystallinity of the framework. In this activated form, guest molecules can occupy the void spaces that once housed solvents of crystallization. A second transformation to a 2D network was also observed.19
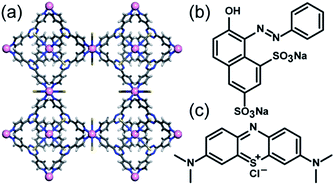 |
| Fig. 1 (a) Structure of CoSP (C = gray, N = blue, Co = pink, S = yellow, CCDC no. 766604,† ref. 17); organic dye pollutants orange G (b) and methylene blue (c). | |
Results and discussion
In this work, the crystalline sponge method for the absorption of an anionic dye, orange G (OG, Fig. 1b), and a cationic dye, methylene blue (MB, Fig. 1c) was investigated. CoSP crystals were synthesized in 1
:
1 (v/v) o-C6H4Cl2
:
MeOH as published in the literature.16 Crystals still immersed in the mother liquor were combined with solutions containing OG or MB. The combined solutions were slowly evaporated to concentrate the dyes.20 The encapsulation of OG and MB into i-CoSP were observed within a few minutes, as shown in videos S1 and S2 (ESI†). The encapsulation process is more distinct with MB, wherein the orange crystals become light green (Fig. 2a and b). Although single crystals were obtained that readily reveal the metal–organic backbone, the location of encapsulated dyes could not be determined due to disorder. The tendency of guests to display static disorder was also observed by Fujita and co-workers.20
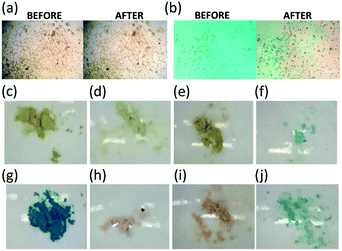 |
| Fig. 2 Images of CoSP crystals before and after absorption of (a) OG and (b) MB; (c) i-CoSP powder, (d) after adsorption of water, (e) OG, and (f) MB; and (g) a-CoSP powder, (h) after adsorption of water, (i) OG, and (j) MB. | |
We were interested in determining if the loss of crystallinity of CoSP had an effect on the competency of the material for host/guest chemistry, particularly for organic dyes. The crystalline sponge method is predicated on maintaining single crystal quality, thus the host/guest chemistry of the semi-amorphous state has not been explored. For these experiments, microcrystalline CoSP was prepared at a 100 mg scale.17
Upon synthesis, orange microcrystals were obtained. Filtration of these microcrystals delivers a fine orange powder (i-CoSP, Fig. 2c). Air-drying or activation at 120 °C under vacuum effects a change to a green powder (activated, Fig. 2g).
PXRD patterns of i-CoSP (Fig. 3a) and a-CoSP (Fig. 3d) are very similar, and also match the pattern of the green semi-amorphous material reported by Murugesu and co-workers. SEM provides further evidence that a-CoSP represents a conversion to the semi-amorphous state. The facets observed of the crystallites of a-CoSP are softened but still observable in the activated material (Fig. S1†). Our results are consistent with the observations of Murugesu, wherein the transformation into a green powder occurs upon the loss of solvent molecules that occupy voids in the coordination polymer.
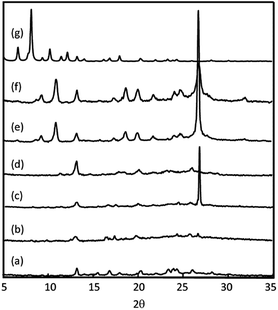 |
| Fig. 3 PXRD patterns of (a) i-CoSP; (b) i-CoSP + H2O; (c) i-CoSP + aqueous OG; (d) a-CoSP; (e) a-CoSP + H2O; (f) a-CoSP + aqueous OG; and (g) simulated. | |
Thermogravimetric analysis
TGA curves (Fig. 4) showed three significant mass-loss steps for these materials. The first step at 150 °C is consistent with the removal of H2O, MeOH, and o-C6H4Cl2. The mass-loss at 350 °C has previously been attributed to the decomposition of the TPT ligands.21
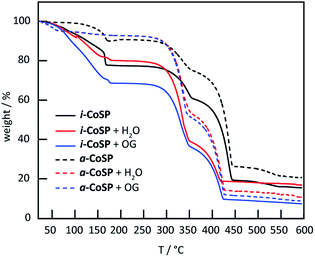 |
| Fig. 4 TGA curves of inactive and activated Co sponge powder before and after uptake of water and OG. | |
The final step at 450 °C corresponds to the decomposition of the SCN− ligands.19 Both i-CoSP and a-CoSP showed mass loss as the samples were heated to 150 °C; however, the inactivated sample underwent a larger percent change, consistent with the loss of a greater amount of solvent molecules relative to the activated cage, in which many solvents had already been removed. As the temperature exceeded ∼160 °C, a steep drop in % weight was observed attributed to a loss of o-C6H4Cl2.
Fourier transform infrared spectroscopy
Fourier transform infrared spectroscopy (FT-IR) analysis further supported the hypothesis of solvent removal upon activation without a total loss of the framework. The FT-IR spectra of both the inactive and activated sponges (Fig. 5) showed characteristic CN stretching bands at <2100 cm−1 that correspond to the SCN− ligands.22,23
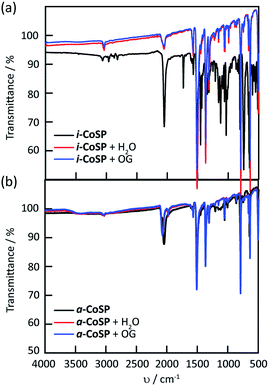 |
| Fig. 5 FT-IR spectra of (a) inactive and (b) activated Co sponge powder before and after uptake of water and OG. | |
The broad band at ∼3350 cm−1 typically observed for OH stretching is absent in both materials. However, stretching (3030 and 1600 cm−1) and bending (690–900 cm−1) bands for the aromatic rings and stretching (800–550 cm−1) bands for C–Cl are observed in i-CoSP. These bands are attenuated in a-CoSP, as expected if the o-C6H4Cl2 are removed during activation.
Dye update studies
Both inactive and activated powders were tested for the uptake of OG and MB. ∼10 mg of material were added to 40 μM (18 mg L−1) OG and 10 μM (4 mg L−1) MB aqueous solutions. These mixtures were stirred for 30 min at room temperature. The solutions were analysed over the course of 2 days. The electronic absorption spectra showed an 8% decrease in OG concentration for i-CoSP and a 55% decrease for a-CoSP (Fig. 6a and b). i-CoSP removes a significant amount of MB (70% decrease in MB concentration). The use of a-CoSP drops the dye concentration even further, by 93% (Fig. 6c and d). Fig. 7 summarizes the absorption capacities (qe) of 10 mg inactive and activated powders for OG and MB. In all cases, dye uptake is completed within 24 h, resulting in capacities of 0.4 mg g−1 for i-CoSP + OG; 4.6 mg g−1 for a-CoSP + OG; 1.4 mg g−1 for i-CoSP + MB; and 1.8 mg g−1 for a-CoSP + MB. These values indicate that activation is necessary to maximize the uptake capacity of the Co sponge for both anionic and cationic dyes.
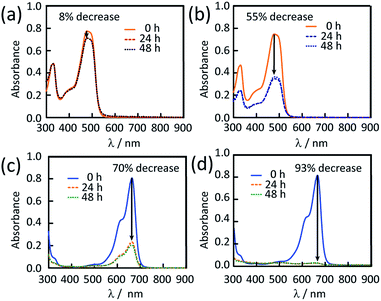 |
| Fig. 6 UV-vis spectra for the dye absorption of i-CoSP (left) and a-CoSP (right) with OG (top) and MB (bottom). | |
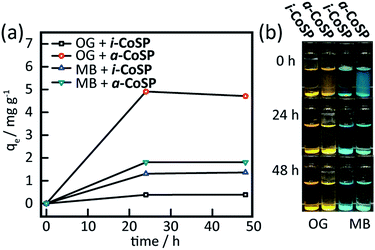 |
| Fig. 7 (a) Absorption capacity (qe in mg dye per g Co sponge) of inactive and activated Co sponge powder for OG and MB and (b) images of OG and MB solutions after 0, 24, and 48 h of adding inactive and activated Co sponge powder. | |
Absorption kinetics
The kinetics of the absorption processes were investigated using a series of absorption experiments (Fig. S2a and b†).14 The absorption capacities of MB into both i-CoSP and a-CoSP were plotted against time and the data were fit with first-order kinetics, providing rate constants (k1) of 0.09 and 0.20 s−1, respectively (Table S1†), indicating that the activated material more rapidly removes the dye from solution. In both cases, the maximum absorption capacity is achieved within 40 minutes. Due to the settling time required for scatter-free spectra, OG uptake was measured every 20 minutes and all spectral changes were complete in the blind-time following initial mixing. Since all uptake occurred within the first 20 minutes, it is clear that OG is sequestered faster than MB.
The ability to remove guests from such systems is attractive insomuch that the material may then be reused to sequester additional molecules.24 The equilibrium constant associated with the host/guest complexation plays a significant role in the ease with which guests may be removed. The kinetics of the release of OG and MB were investigated (Fig. S2c, d and Table S1†). Using a first-order kinetic model, k−1 for dye release was found to be 0.04 s−1 for OG. For MB, small spectral changes were observed when the material was reintroduced to solution; however, these changes are consistent with a small amount of network dissolving and thus releasing the guest, rather than release from the intact CoSP. The lack of any significant absorption from free MB obviates a determination of k−1 and indicates that MB binds much more strongly to the material.25
The water stabilities of i-CoSP and a-CoSP were determined using UV-vis spectroscopy. CoSP has a characteristic band at 500 nm that is used to monitor stability, though the material is very insoluble (A ∼ 0.02) over the course of 48 hours this band is largely unchanged, with evidence for a slight amount of further dissolution (Fig. S3a and b†). The shoulder at 350 nm is due to absorption by the encapsulated o-C6H4Cl2 in the inactive material.26
X-ray methods
We noted an additional crystal transformation behaviour over the course of our studies. When the semi-amorphous activated powder is exposed to either water or solutions of dyes in water, the PXRD patterns indicate that crystallinity is increased relative to the initial material (Fig. 3). The increase in crystallinity occurs with restoration of solvent molecules back into the coordination network as evidenced by the growth of characteristic bands in the FT-IR spectra (Fig. 5). The presence of encapsulated water is observed as a broad band at 3350 cm−1 in the FT-IR spectra (Fig. 5b). Images of the powders, shown in Fig. 2, illustrate that the activated material (Fig. 2g) undergoes a significant colour change when suspended in water (Fig. 2h), or when exposed to the dyes (Fig. 2i and j). Similar changes occur for the inactivated material, however given the orange nature of initial state (Fig. 2c), the changes are subtler. Upon reactivation (r-CoSP), i.e. activated material is exposed to solvent or dye, then reheated at 120 °C for 10 h, solvent removal is very minimal based on the TGA curves (Fig. S4†) and the PXRD patterns do not show significant differences to those of the singly-activated materials (Fig. S5f and h†). This is consistent with a slow dye release rate wherein dyes largely remain and prevent reuptake. However, r-CoSP is still water stable as demonstrated by the minimal decrease in absorption at 500 nm compared to the activated sponge (Fig. S3c†).
X-ray photoelectron spectroscopy (XPS) has been used to provide information about the composition of extended networks and the oxidation states any metal ions present in a sample.27 XPS was conducted on a-CoSP, a-CoSP + H2O, a-CoSP + OG, and a-CoSP + MB. In all four samples, survey spectra showed the presence of Co(II), N, C, S, and O. The first four components are directly part of the CoSP network, whereas the oxygen originates from H2O or from the dyes, when present. As shown in Fig. S7–S10,† high-resolution peaks corresponding to C 1s indicate a significant amount of non-oxygenated carbon (∼284.8 eV) with some C–N contributions at higher energy (∼286.9 eV).28 In all of the samples, the Co 2p binding energies show 2p1/2 and 2p3/2 peaks at ∼797.2 eV and ∼781.2 eV, respectively. These peaks have satellite bands shifted to higher energy by ∼6 eV, diagnostic of Co(II).29,30 Although the bands corresponding to all five elements (Co, N, C, S, O) are present in all samples, the intensities and high resolution fits differ due to the compositional changes associated with activation and guest uptake.
Experimental
Materials and methods
All reagents and solvents were reagent grade and used as received without further purification. 2,4,6-Tris(4-pyridyl)-1,3,5-triazine (TPT), Orange G (OG), and o-dichlorobenzene (o-C6H4Cl2) were purchased from TCI Chemicals. Methylene blue (MB) was purchased from Acros Organics. Cobalt(II) thiocyanate (Co(NCS)2) was purchased from Alfa Aesar. Methanol (MeOH) was purchased from Fisher Chemical. Deionized water was used in all absorption, release, and stability experiments. All UV-vis spectra were acquired from an Agilent Cary 8454 UV-vis Diode Array system with a 10 mm rectangular quartz cuvette. Blank spectra with deionized water were acquired in between runs. All powder X-ray diffraction (PXRD) patterns were collected from a Rigaku Ultima IV system with inert atmosphere attachment and heated sample chamber equipped with Cu source (0.15418 nm Kα) and operated at 1.76 kW power (40 kV, 44 mA). Diffraction patterns were measured within 2θ range of 5 to 35° at a rate of 1° min−1. Thermogravimetric analysis (TGA) curves were measured from a TA Instruments DSC SDT Q600 instrument at a heating rate of 5° min−1 from 25–600 °C under constant flow of nitrogen (100 mL min−1). Fourier transform-infrared (FT-IR) spectra were acquired from a Perkin Elmer 1760 FT-IR spectrometer equipped with horizontal attenuated total reflectance (HATR) from 4000 to 500 cm−1 wavenumbers. Field Emission Scanning Microscope (FESEM) images were captured using a Hitachi SU70 FESEM with Oxford Energy-dispersive X-ray Spectrometer (EDS). All X-ray photoelectron spectroscopy (XPS) spectra were measured using a Physical Electronics Phi Versaprobe 5000 with Al Kα source instrument.
Syntheses
Synthesis of crystalline Co sponge (CoSP). Co sponge crystals, {[(Co(NCS)2)3(TPT)4]·25(o-C6H4Cl2)·5(MeOH)}n, were grown following a published procedure.16 A solution of TPT (6.3 mg, 20 μmol) in 5 mL of 1
:
4 (v/v) MeOH
:
o-C6H4Cl2 was placed in a dram vial. Carefully layered on top of this is a 1 mL MeOH solution of Co(NCS)2 (7.0 mg, 40 μmol). This solution was left to stand for 7 days or until orange crystals are formed on the glass surface.
Synthesis of inactive Co sponge powder (i-CoSP). {[(Co(NCS)2)3(κ3-TPT)4]·a(H2O)·b(MeOH)}n (i-CoSP),19 was synthesized following a published procedure.17 A solution of TPT (315 mg, 1.0 mmol) in 250 mL of 1
:
4 (v/v) MeOH
:
o-C6H4Cl2 was placed in a 500 mL round bottom flask and stirred at 600 rpm. A 50 mL MeOH solution of Co(NCS)2 (350 mg, 2.0 mmol) was added to the TPT solution. The mixture was stirred for 15 min and left to stand for 2 h. i-CoSP was collected by filtration and washed with o-C6H4Cl2.
Activated Co sponge powder (a-CoSP). {[(Co(NCS)2)3(κ0–3-TPT)4]·c(H2O)}n19 (a-CoSP) was prepared by pre-treating the synthesized powder before use.31 This involves heating the synthesized i-CoSP at 120 °C under vacuum for at least 10 h.
Conclusions
In summary, we have established that CoSP MOF first reported by Fujita and co-workers is capable of serving as a host for two organic dyes, OG and MB. The absorption capability of the sponge is greatly enhanced by removal of trapped solvents by activating with heat at 120 °C for 10 h. The activation accelerates the transformation to a semi-amorphous material previously discovered by Murugesu and co-workers. We have shown that this activation altered the crystallinity of the MOF but does not hinder host/guest chemistry. Removal of trapped solvents frees up void spaces for dye encapsulation. CoSP does not degrade in aqueous solution and remains capable of dye adsorption even in a semi-amorphous state. The crystallinity of the material was greatly affected by this host/guest chemistry. The semi-amorphous activated form regained crystallinity when immersed in water or solutions of dyes. Although the kinetics of uptake were faster for OG, the cationic MB bound much more tightly to the framework, with minimal reversibility.
Acknowledgements
TRC thanks the University at Buffalo and State University of New York Research Foundation for support. The authors thank Dr Joshua Wallace for assistance with XPS measurements.
Notes and references
- G. Booth, H. Zollinger, K. McLaren, W. G. Sharples and A. Westwell, in Ullmann's Encyclopedia of Industrial Chemistry, Wiley-VCH Verlag GmbH & Co. KGaA, 2000, DOI:10.1002/14356007.a09_073.
- C.-C. Wang, J.-R. Li, X.-L. Lv, Y.-Q. Zhang and G. Guo, Energy Environ. Sci., 2014, 7, 2831–2867 CAS.
- W.-T. Tsai, H.-C. Hsu, T.-Y. Su, K.-Y. Lin, C.-M. Lin and T.-H. Dai, J. Hazard. Mater., 2007, 147, 1056–1062 CrossRef CAS PubMed.
- Environmental Chemistry of Dyes and Pigments, ed. A. F. Reife and H. S. Freeman, John Wiley and Sons, New York, 1996 Search PubMed.
- O. J. Hao, H. Kim and P.-C. Chiang, Crit. Rev. Environ. Sci. Technol., 2000, 30, 449–505 CrossRef CAS.
- Y. M. Slokar and A. Majcen Le Marechal, Dyes Pigm., 1998, 37, 335–356 CrossRef CAS.
- S. Wang, H. Sun, H. M. Ang and M. O. Tadé, Chem. Eng. J., 2013, 226, 336–347 CrossRef CAS.
- S. C. N. Tang and I. M. C. Lo, Water Res., 2013, 47, 2613–2632 CrossRef CAS PubMed.
- X. Wang, M. Zhu, H. Liu, J. Ma and F. Li, Sci. Total Environ., 2013, 449, 157–167 CrossRef CAS PubMed.
- X. Zhao, L. Lv, B. Pan, W. Zhang, S. Zhang and Q. Zhang, Chem. Eng. J., 2011, 170, 381–394 CrossRef CAS.
- D. Shuai, D. C. McCalman, J. K. Choe, J. R. Shapley, W. F. Schneider and C. J. Werth, ACS Catal., 2013, 3, 453–463 CrossRef CAS.
- E. M. Dias and C. Petit, J. Mater. Chem. A, 2015, 3, 22484–22506 CAS.
- A. Ayati, M. N. Shahrak, B. Tanhaei and M. Sillanpää, Chemosphere, 2016, 160, 30–44 CrossRef CAS PubMed.
- Z.-P. Qi, J.-M. Yang, Y.-S. Kang, F. Guo and W.-Y. Sun, Dalton Trans., 2016, 45, 8753–8759 RSC.
- J. J. Low, A. I. Benin, P. Jakubczak, J. F. Abrahamian, S. A. Faheem and R. R. Willis, J. Am. Chem. Soc., 2009, 131, 15834–15842 CrossRef CAS PubMed.
- Y. Inokuma, T. Arai and M. Fujita, Nat. Chem., 2010, 2, 780–783 CrossRef CAS PubMed.
- S. Matsuzaki, T. Arai, K. Ikemoto, Y. Inokuma and M. Fujita, J. Am. Chem. Soc., 2014, 136, 17899–17901 CrossRef CAS PubMed.
- Y. Y. Inokuma Shota, A. Junko, A. Tatsuhiko, H. Yuki, T. Kentaro, M. Shigeki, R. Kari and F. Makoto, Nature, 2013, 495, 461–466 CrossRef PubMed.
- G. Brunet, D. A. Safin, I. Korobkov, A. Cognigni and M. Murugesu, Cryst. Growth Des., 2016, 16, 4043–4050 CAS.
- M. Hoshino, A. Khutia, H. Xing, Y. Inokuma and M. Fujita, IUCrJ, 2016, 3, 139–151 CAS.
- M.-X. Li, Z.-X. Miao, M. Shao, S.-W. Liang and S.-R. Zhu, Inorg. Chem., 2008, 47, 4481–4489 CrossRef CAS PubMed.
- K. Nakamoto, in Infrared and Raman Spectra of Inorganic and Coordination Compounds, John Wiley & Sons, Inc., 2008, ch. 2, pp. 149–354, DOI:10.1002/9780470405840.
- B. Schrader, in Infrared and Raman Spectroscopy, Wiley-VCH Verlag GmbH, 2007, pp. 711–764, DOI:10.1002/9783527615438.refs.
- S.-N. Sheng, Y. Han, B. Wang, C. Zhao, F. Yang, M.-J. Zhao, Y.-B. Xie and J.-R. Li, J. Solid State Chem., 2016, 233, 143–149 CrossRef CAS.
- M. Roushani, Z. Saedi and T. Musa beygi, J. Taiwan Inst. Chem. Eng., 2016, 66, 164–171 CrossRef CAS.
- J. G. Mattay and A. G. Griesbeck, in Photochemical Key Steps in Organic Synthesis, Wiley-VCH Verlag GmbH, 2007, pp. 261–283, DOI:10.1002/9783527615797.ch05.
- J. Jiang, L. Huang, X. Liu and L. Ai, ACS Appl. Mater. Interfaces, 2017, 9, 7193–7201 CAS.
- S. Bag, K. Roy, C. S. Gopinath and C. R. Raj, ACS Appl. Mater. Interfaces, 2014, 6, 2692–2699 CAS.
- W. Wei, W. Chen and D. G. Ivey, Chem. Mater., 2008, 20, 1941–1947 CrossRef CAS.
- S. Bennici, H. Yu, E. Obeid and A. Auroux, Int. J. Hydrogen Energy, 2011, 36, 7431–7442 CrossRef CAS.
- F.-R. Dai, D. C. Becht and Z. Wang, Chem. Commun., 2014, 50, 5385–5387 RSC.
Footnote |
† Electronic supplementary information (ESI) available: Experimental details of dye uptake and release; UV-vis spectra; TGA data; PXRD patterns; FT-IR spectra; XPS spectra. See DOI: 10.1039/c7ra02286g |
|
This journal is © The Royal Society of Chemistry 2017 |
Click here to see how this site uses Cookies. View our privacy policy here.