DOI:
10.1039/C7RA01444A
(Paper)
RSC Adv., 2017,
7, 34705-34713
Ag3PO4@holmium phosphate core@shell composites with enhanced photocatalytic activity
Received
4th February 2017
, Accepted 9th June 2017
First published on 11th July 2017
Abstract
In this study, Ag3PO4@holmium phosphate (HP) composite photocatalysts were first prepared by silver-ammonia-solution-assisted solution co-deposition. During co-deposition, Ag3PO4 particles were encapsulated by a flocculent amorphous substance layer of HP, affording a Ag3PO4@HP core–shell heterojunction structure with a Ho/Ag molar ratio of 0.05/2.95. The core–shell structure significantly improved the photocatalytic activity of Ag3PO4. In addition, the photocatalytic mechanism of Ag3PO4@HP was discussed.
1. Introduction
Semiconductor photocatalysts have attracted considerable attention as they demonstrate the potential of addressing energy and environmental issues,1–4 including photochemical water splitting,5 organic pollutant degradation,6 and CO2 photocatalytic reduction.7 In 2010, Ye reported a novel semiconductor photocatalyst Ag3PO4, which exhibited strong photo-oxidative activities for O2 evolution from water splitting in the presence of a sacrificial reagent AgNO3, and a high degradation rate for organic dyes under visible-light irradiation.8 The photocatalytic activity of Ag3PO4 is superior to those of previously reported visible-light photocatalysts, e.g., BiVO4 (ref. 8) and N-doped TiO2.9,10 Hence, it has been widely investigated.11–16
According to previously reported studies, the heterojunction structure between Ag3PO4 and other semiconductor materials may modulate the band gap, improve dispersion stability, and enhance the separation efficiency of photoelectron–hole pairs, leading to the enhanced photocatalytic activity of the composite catalyst. Several Ag3PO4-based composite photocatalysts have been developed, e.g., AgX/Ag3PO4 (X = Cl, Br, and I),17–19 Ag3PO4/TiO2,20,21 Ag3PO4/CeO2,22,23 Ag3PO4/WS2,24 Bi2O3/Ag3PO4,25 Ag3PO4/BiPO4,26–28 Ag3PO4/BiOBr,29 and Ag3PO4/g-C3N4.30–32
In this study, Ag3PO4 and Ag3PO4@HP photocatalysts were prepared by silver-ammonia- solution-assisted solution co-deposition. The core–shell structure and photocatalytic properties of Ag3PO4@HP were investigated in detail.
2. Experimental
2.1 Preparation
2.1.1 Preparation of Ag3PO4. First, AgNO3 and KH2PO4 were separately dissolved in distilled water. Second, a NH3·H2O solution was slowly added to the AgNO3 solution under constant magnetic stirring, affording a silver ammonia solution, until the brown precipitate disappeared. Then, a KH2PO4 solution with a Ag(NH3)2NO3
:
KH2PO4 molar ratio of 1
:
3 was added into the silver ammonia solution under intense stirring for 5 min before standing for 10 min, and the precipitates were collected by centrifugation. The obtained precipitate was washed three times with absolute ethanol and distilled water, followed by drying at 80 °C for 30 min, affording Ag3PO4.
2.1.2 Preparation of Ag3PO4@HP. First, Ho2O3 was dissolved in nitric acid, affording a Ho(NO3)3 solution. Second, the Ho(NO3)3 solution was mixed with the silver ammonia solution in a molar ratio of Ho/Ag/PO43− at x/3–x/1 (x = 0.01, 0.05, 0.1, and 0.5). The next steps were the same as those for the preparation of Ag3PO4. The prepared Ag3PO4@HP samples were denoted as 1-Ag3PO4@HP, 2-Ag3PO4@HP, 3-Ag3PO4@HP, and 4-Ag3PO4@HP, corresponding to x values of 0.01, 0.05, 0.1, and 0.5, respectively.
2.2 Photocatalytic activity experiments
Photocatalytic activity experiments were carried out in a photoreactor at room temperature. BL-GHX-V Xe lamp with a 500 W electric power was used as the light source to simulate sunlight. The reaction solution included 30 mL of a rhodamine B, dahlia B, or methylthionine chloride solution, with an initial concentration of 5 mg L−1, along with 0.05 g of the photocatalyst, respectively. First, the suspensions were stirred in the dark for 30 min to attain the adsorption–desorption equilibrium. After irradiation was started, the reaction solution was removed and immediately filtered after every 15 min. The reaction solution concentration was measured by UV-Vis spectroscopy to evaluate the residual efficiency of the reaction solution. The residual efficiency can be calculated by the following equation, η = C/C0, where C0 (mg L−1) is the initial concentration of the reaction solution before illumination, C is the concentration of the reaction solution (mg L−1) after a certain illumination time (min), which is proportional to the absorbance.
2.3 Characterization
X-ray diffraction (XRD) patterns were recorded on a XRD instrument (BDX3200) with CuKα radiation. The morphology images and energy-dispersive X-ray (EDX) spectra of the samples were obtained by field-emission scanning electron microscopy (FE-SEM, S-4800). Transmission electron microscopy (TEM) and high-resolution transmission electron microscopy (HRTEM) images were recorded on a TEM (FEI F30) instrument. Binding energy (BE) was analyzed on a Perkin-Elmer PHI-1600 X-ray photoelectron spectroscopy (XPS) system, which was calibrated using adventitious carbon (C 1s) as the reference peak. Photoluminescence (PL) and afterglow curves were recorded on a Hitachi F-7000 fluorescence spectrophotometer (Japan) equipped with a 450 W Xe lamp as the excitation light source. UV-Vis diffuse reflectance spectra (UV-Vis DRS) were obtained on a UV-Vis spectrometer (Puxi, UV1901), with an integral sphere, using BaSO4 as the reference in the measurement range from 220 nm to 800 nm.
3. Results and discussion
3.1 Structural characterization
Fig. 1 shows the XRD patterns of Ag3PO4@HP and Ag3PO4. All of the diffraction peaks for as-prepared Ag3PO4 were indexed to the cubic phase of Ag3PO4 (JCPDS card 06-0505, Fig. 1). The XRD pattern of PH did not exhibit strong diffraction peaks, indicative of poor crystallinity. At low Ho3+ concentrations (x ≤ 0.1), nearly no impurity peaks were observed for Ag3PO4@HP, and no obvious shift in the diffraction peaks was observed compared to those observed for pure Ag3PO4. The values of the lattice parameter a were 6.0190, 6.0154, 6.0194, and 6.0190 for the samples 1-Ag3PO4@HP to 4-Ag3PO4@HP, respectively, indicating that low Ho3+ concentrations almost do not affect the crystal lattice parameters of Ag3PO4. However, with the increase in the Ho/Ag molar ratio to 0.5/2.5, the strength of diffraction peaks significantly decreased, suggesting that Ho3+ does not enter the crystal lattice of Ag3PO4, but forms a new compound phase HP.
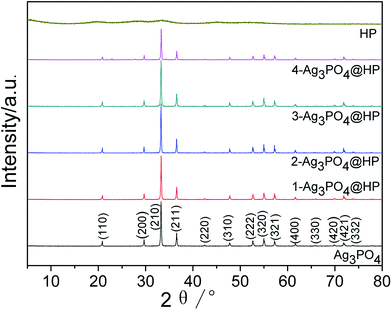 |
| Fig. 1 XRD patterns of Ag3PO4, HP and Ag3PO4@HP with the mole ratio of Ho/Ag at 0.01 : 2.99, 0.05 : 2.95, 0.1 : 2.9, 0.5 : 2.5. | |
Fig. 2 shows the FE-SEM images of Ag3PO4 and Ag3PO4@HP. Without the introduction of Ho3+, globular Ag3PO4 particles were primarily observed with sizes ranging from 200 to 300 nm (Fig. 2a). However, at a Ho/Ag molar ratio of 0.05/2.95, regular cubes were observed for Ag3PO4 particles, with sizes increasing to 300–400 nm (Fig. 2b). Ag3PO4 particles were coated by a thin layer of a flocculent amorphous substance HP, which was clearly observed at the junction of two neighboring particles; this observation was further confirmed from the TEM and HRTEM results (Fig. 4c and d). The crystalline-phase structure of the Ag3PO4 particles was distinctly improved, growing under the action of a thin layer of HP. As shown in Fig. 2c, with the increase in the Ho/Ag molar ratio to 0.1/2.9, the amount of flocculent amorphous substance HP rapidly increased. In Fig. 2c, the Ag3PO4 particle surface was surrounded by HP, making the shape of the Ag3PO4 particles irregular. Moreover, with the increase in the Ho/Ag molar ratio to 0.5/2.5, Ag3PO4 particles were fully encapsulated in the massive HP, and the size of the Ag3PO4 particles decreased to 100–200 nm.
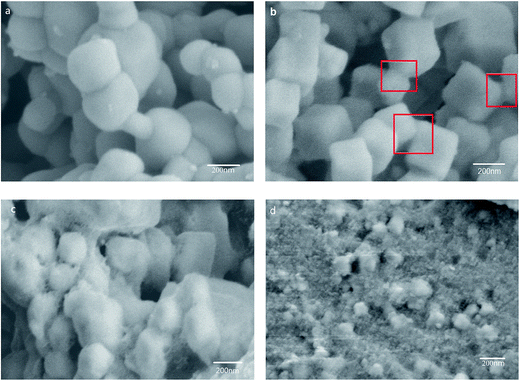 |
| Fig. 2 FE-SEM images of Ag3PO4 (a) and Ag3PO4@HP with the mole ratio of Ho/Ag at 0.05 : 2.95 (b), 0.1 : 2.9 (c), 0.5 : 2.5 (d). | |
EDX elemental mapping images were also recorded for further confirming the 2-Ag3PO4@HP composite structure. The elemental mapping images of silver, phosphorus, and oxygen exhibited similar shape as well as location (Fig. 3), confirming the existence of Ag3PO4 in the composite. The mapping of Ho revealed that Ho is well distributed in the sample, indicative of the presence of holmium. Furthermore, the mapping density of Ag on the surface was clearly greater than that of the other elements, which is possible for providing more photoelectrons for photocatalysis.
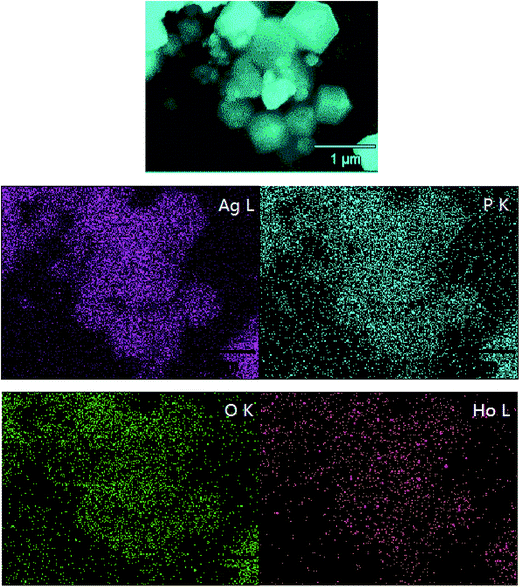 |
| Fig. 3 EDX elemental mapping images of 2-Ag3PO4@HP with the mole ratio of Ho/Ag at 0.05 : 2.95. | |
Fig. 4 shows the TEM and HRTEM images of the Ag3PO4 and 2-Ag3PO4@HP samples. The size of the predominantly globular Ag3PO4 particles was ∼200–300 nm, and Ag3PO4 particles exhibited a smooth surface with few edges and corners, but there was serious aggregation (Fig. 4a). As shown in Fig. 4b, Ag3PO4 particles presented a cubic shape with a mean size of ∼350 nm. The sharp edges and corners of the Ag3PO4 particles significantly increased. It is because that a small amount of the HP flocculent amorphous substance was generated on the surface or in the vicinity of the Ag3PO4 particles, improving the dispersion of Ag3PO4 particles and permitting the unrestricted growth of Ag3PO4 particles into cubes. This result is consistent with FE-SEM results. The specific surface areas of Ag3PO4 and Ag3PO4@HP were estimated as 3 m2 g−1 and 11 m2 g−1, respectively. The higher specific surface area of Ag3PO4@HP is related to the amorphous structure of HP and the improved dispersion of Ag3PO4.
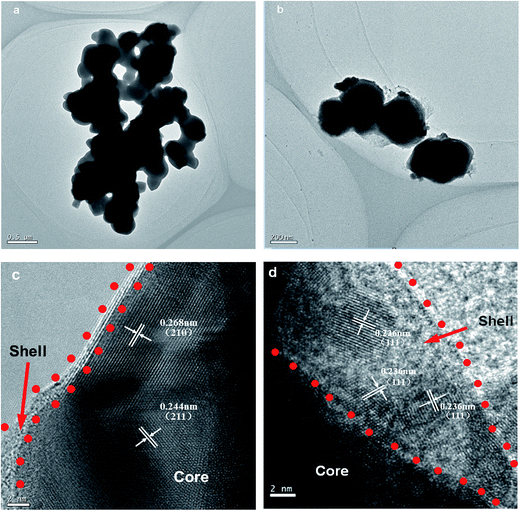 |
| Fig. 4 TEM images of Ag3PO4 (a) and 2-Ag3PO4@HP (b) and HRTEM of 2-Ag3PO4@HP (c, d) with the mole ratio of Ho/Ag at 0.05 : 2.95. | |
In the HRTEM image shown in Fig. 4a, c and d crystalline lattice structure for 2-Ag3PO4@HP was clearly observed. As shown in Fig. 4c and d, Ag3PO4 particles were further confirmed to be encapsulated by an HP layer, affording the Ag3PO4@HP core–shell composite. The shell layer of the HP amorphous material was distinctly different from the Ag3PO4 core, and the thickness of the shell layer was approximately 2–10 nm. Lattice spacings of 0.27 and 0.24 nm were observed, corresponding to the (210) and (211) planes of Ag3PO4, respectively. Interestingly, as shown in Fig. 4d, some small particles with sizes of 4–5 nm were crystallized in the thicker HP shell. The lattice spacing of these small particles was 0.236 nm, corresponding to the (111) planes of Ag0. Ag0 is possibly produced by the reduction of Ag+, owing to daylight illumination during preparation.21 The reaction can be proposed as shown in eqn (1):
|
2AgNO3 = 2Ag + 2NO2↑ + O2↑
| (1) |
Fig. 5a shows the full-scan XPS spectra of Ag3PO4 and 2-Ag3PO4@HP: all elements (Ag(b), O(c), P(d), and Ho(e)) were observed in 2-Ag3PO4@HP, among which Ho was only observed in Ag3PO4@HP. Fig. 5b shows the Ag 3d spectra of Ag3PO4 and Ag3PO4@HP samples: Two characteristic BE peaks were observed at 367.7/373.9 eV and 367.6/373.8 eV, attributed to Ag 3d5/2 and Ag 3d3/2 bands, respectively. After the peak fitting of O 1s, two sets of binding energy at 530.6/532.7 eV and 530.4/532.5 eV, corresponding to Ag3PO4 and Ag3PO4@HP samples, respectively (Fig. 5c). Two peaks corresponded to the crystal lattice oxygen and surface hydroxyl groups, respectively. The P 2p peaks of 2-Ag3PO4@HP and Ag3PO4 were observed at 133.2 and 132.8 eV, respectively (Fig. 5d); both peaks were assigned to the sample lattice P5+. Compared with that corresponding to Ag3PO4, the P 2p peak of 2-Ag3PO4@HP slightly shifted to higher BE, indicating that P 2p on the Ag3PO4 surface undergoes a different chemical reaction from the inside, which is the formation of the HP shell. A BE peak was observed at 163.1 eV in the XPS spectrum for Ho (Fig. 5e), corresponding to Ho 4d5/2. This result further indicated the presence of Ho as Ho3+ in the composite.
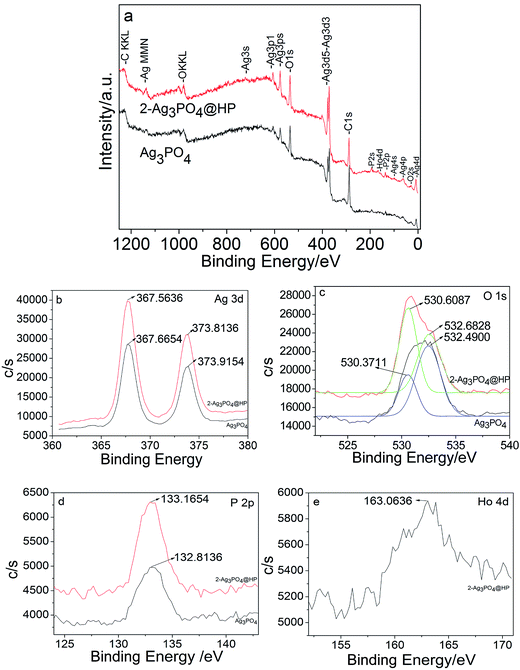 |
| Fig. 5 The XPS results images of Ag3PO4 and 2-Ag3PO4@HP with the mole ratio of Ho/Ag at 0.05 : 2.95. | |
Fig. 6 shows the UV-Vis DRS spectra of Ag3PO4 and 2-Ag3PO4@HP. Absorption edges of Ag3PO4 and Ag3PO4@HP were observed in the visible region (Fig. 6a), and considerable absorption was observed in the visible spectrum in the range of ∼200–530 nm. HoPO4 exhibited strong absorption in the UV region (<350 nm). In addition, some sharp absorption peaks were observed in the visible range of HP, related to the characteristic absorption of Ho3+. Absorption peaks were observed at 421 nm, 447 nm, 457 nm, 477 nm, 540 nm, and 642 nm, corresponding to the energy-level transitions of Ho3+, 5G5 → 5I8, 5G0(5F1) → 5I8, 5G6 → 5I8, 5F3 → 5I8, 5S2(5F4) → 5I8, and 5F5 → 5I8, respectively. The direct band gaps of Ag3PO4 and HP were obtained from the plot of (αhν)2 versus hν, which were 2.42 eV and 3.50 eV, respectively (Fig. 6b).
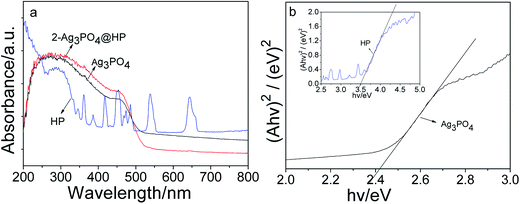 |
| Fig. 6 UV-Vis diffuse reflection spectrum of Ag3PO4, HP and Ag3PO4@HP. | |
3.2 Photocatalytic activity
Fig. 7(a–c) show the photocatalytic degradation of rhodamine B, dahlia B, and methylthionine chloride using Ag3PO4, HP, and Ag3PO4@HP under sunlight irradiation, respectively. Ag3PO4 and Ag3PO4@HP samples almost degraded the three dyes within 45 min with different degradation rates, but HP marginally affected the degradation of all dyes. From the comparison of the photocatalytic experimental results, at a Ho
:
Ag molar ratio of less than 0.1
:
2.9, the photocatalytic activity of Ag3PO4@HP was typically greater than that of pure Ag3PO4. After irradiation for 30 min, 2-Ag3PO4@HP exhibited the best degradation rates of 96.70%, 93.05%, and 93.98% for rhodamine B, dahlia B, and methylthionine, respectively, while the corresponding degradation rates for Ag3PO4 were 74.66%, 69.11%, and 77.58%, calculated from the data shown in Fig. 7(a–c). Generally, the photocatalytic activity of Ag3PO4 fits the first-order kinetics. According to the first-order kinetics (ln(C0/C) = kt), the reaction rate constant k can be calculated (Fig. 7d). The k values for rhodamine B, dahlia B, and methylthionine chloride using 2-Ag3PO4@HP were 0.1251 min−1, 0.1088 min−1, and 0.10317 min−1. These values were 3.1, 2.8, and 2.4 times greater than that of pure Ag3PO4, respectively.
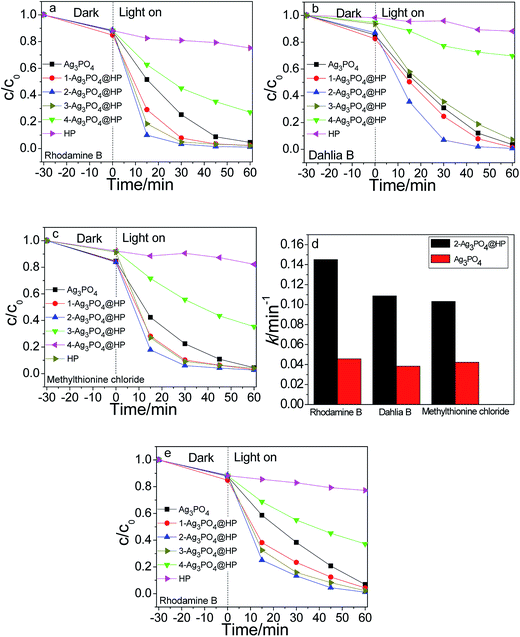 |
| Fig. 7 The photocatalytic degradation results of Ag3PO4, HP and Ag3PO4@HP. The degradation results of rhodamine B (a), dahlia B (b), and methylthionine chloride (c); the values of the reaction rate constant k (d) of the photocatalytic degradation; the visible-light photocatalytic activities (e) in the degradation of rhodamine B, using a Xe-lamp with a 420 nm cutoff filter. | |
Fig. 7e shows the visible-light photocatalytic activities of Ag3PO4 and Ag3PO4@HP for the degradation of rhodamine B using a Xe lamp with a 420 nm cutoff filter. After irradiation for 30 min, the degradation rates of rhodamine B for Ag3PO4, 1-Ag3PO4@HP, ∼4-Ag3PO4@HP, and HP were 56.30%, 72.50%, 84.97%, 81.99%, 37.62%, and 6.07%, respectively. 2-Ag3PO4@HP still exhibited better visible-light photocatalytic activity compared to the other tested photocatalysts.
In addition, the cycling stability of photocatalysts is an important property for practical applications. Hence, the stability of 2-Ag3PO4@HP was examined in terms of the recycling degradation of a rhodamine B solution under sunlight irradiation. As shown in Fig. 8a, after five recycling runs, 2-Ag3PO4@HP completely degraded the reaction solution with a concentration of 5 mg L−1 in 60 min, suggesting a high recycling stability for 2-Ag3PO4@HP. To evaluate the structural stability, the crystalline structures of Ag3PO4 and Ag3PO4@HP before and after recycling experiments were examined (Fig. 8b). No diffraction peaks corresponding to metallic Ag were observed in the unused photocatalyst samples. However, metallic Ag was observed in the HRTEM images of unused 2-Ag3PO4@HP (Fig. 4d), possibly because of the low amount of the metallic Ag, resulting in the absence of the XRD diffraction peaks of Ag. After five recycling runs, diffraction peaks corresponding to metallic Ag were clearly observed in the XRD pattern of pure Ag3PO4 at 2θ = 38.115° and 44.299°, indicating that Ag3PO4 is partially reduced into metallic Ag particles during photocatalytic degradation. Compared to the XRD pattern of pure Ag3PO4, smaller diffraction peaks corresponding to metallic Ag were observed in the XRD pattern of 2-Ag3PO4@HP after five recycling runs, indicating that the HP shell enhances the stability of Ag3PO4.
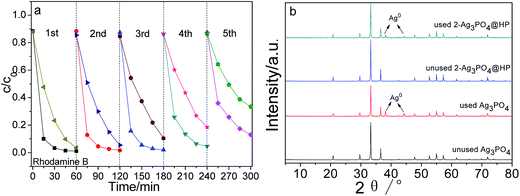 |
| Fig. 8 Recycling runs of Ag3PO4 and 2-Ag3PO4@HoPO4 samples in the degradation of rhodamine B. | |
3.3 Possible photocatalytic mechanism
To examine the reactive species, scavenging experiments were carried out to elucidate the possible pathway or mechanism for photocatalytic degradation. To probe different reactive species during the photocatalysis of Ag3PO4@HP systems, t-butanol, benzoquinone (BQ), EDTA-2Na, and potassium dichromate (K2Cr2O7) were separately added to the RhB solutions as the scavengers ([scavenger] = 0.3 mmol L−1) for hydroxyl radicals (·OH−), superoxide radicals (˙O2), holes (h+), and electrons (e−), respectively (Fig. 9). K2Cr2O7 negligibly affected the photodegradation rates of RhB. The results indicated that e− do not appear to be the main reactive species during photocatalysis. The addition of EDTA–2Na and BQ caused the rapid termination of photocatalysis, indicating that the photogenerated h+ and ˙O2 possibly play a crucial role during the photocatalytic degradation of RhB. The contribution of the reactive species for photocatalytic degradation follows the order of h+ > ˙O2 > ˙OH− > e−.
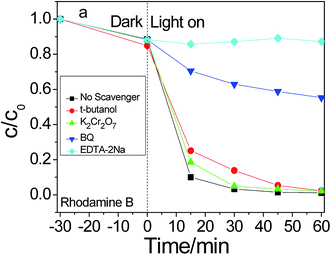 |
| Fig. 9 Photodegradation efficiency of rhodamine B with 2-Ag3PO4@HP as photocatalyst under different scavengers. | |
Considering the energy-band structure theory, a previous study,33 and structural and performance characteristics, a possible energy-band structure model was proposed for improving the photocatalytic activity and stability of the Ag3PO4@HP heterostructures, as shown in Fig. 10. The valence-band (VB) and conduction-band (CB) potentials of Ag3PO4 can be calculated by the following empirical formula (as shown in eqn (2) and (3), respectively), where X is the Mulliken electronegativity (XHP ≅ 6.3205 eV), Ee is the energy of free electrons on the hydrogen scale (Ee ≅ 4.5 eV), and Eg is the bandgap (Eg = 3.5 eV). Therefore, the VB and CB potentials of HP were calculated as 3.44 eV and −0.06 eV, respectively.
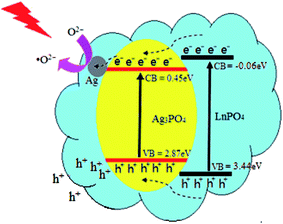 |
| Fig. 10 The photocatalytic mechanism model of Ag3PO4@HP. | |
Under Xe lamp irradiation, both Ag3PO4 and HP were excited, and the photogenerated electrons and holes were simultaneously created. The CB potential (0.45 eV) of Ag3PO4 was lower than that of HP (−0.06 eV), and the VB potential (2.89 eV) was greater than that of HP (3.44 eV). Thus, the photogenerated electrons and holes spontaneously shift from HP to Ag3PO4. Simultaneously, the photogenerated electrons rapidly migrate from the CB of Ag3PO4 to the Ag nanoparticles via the Schottky barrier. Ag nanoparticles on the Ag3PO4 surface acted as a sink for electrons, these electrons reacted with the adsorbed O2 to generate the active ion species (˙O2−). This process promoted the separation of the photogenerated electron–hole pairs. However, the holes (h+) in the VB of Ag3PO4 were directly transferred to the organic dye surface for further reaction. Thus, this photocatalytic system, consisting of LnPO4, Ag nanoparticles, and Ag3PO4, may be more effective for the degradation of organic pollutants.
In addition, the higher specific surface area of Ag3PO4@HP was crucial for enhancing the photocatalytic activity of Ag3PO4, related to the more efficient adsorption of the dye molecules. Therefore, the high photocatalytic activity of Ag3PO4@HP is believed to be related to the combination of two effects.
4. Conclusions
In this study, Ag3PO4@Ag3PO4@HP composite photocatalysts were prepared by silver-ammonia-solution-assisted solution co-deposition. The core–shell structure of the Ag3PO4@HP composite significantly improved the photocatalytic activity of Ag3PO4.
Acknowledgements
The project was supported by the National Natural Science Fund of China (Grant No. 51572069).
References
- A. Fujishima and K. Honda, Electrochemical photocatalysis of water at semiconductor electrode, Nature, 1972, 238, 53–58 CrossRef.
- R. Asahi, T. Morikawa, T. Ohwaki, K. Aoki and Y. Taga, Visible-light photocatalysis in nitrogen-doped titanium oxides, Science, 2001, 293, 269–271 CrossRef CAS PubMed.
- M. Z. Ge, C. Y. Cao, J. Y. Huang, S. H. Li, Z. Chen, K. Q. Zhang, S. S. Aldeyab and Y. K. Lai, A review of one-dimensional TiO2 nanostructured materials for environmental and energy applications, J. Mater. Chem. A, 2016, 4, 6772–6801 CAS.
- S. Obregón and G. Colón, On the origin of the photocatalytic activity improvement of BiVO4 through rare earth tridoping, Appl. Catal., A, 2015, 501, 56–62 CrossRef.
- X. F. Zhang, B. Y. Zhang, K. Cao, J. Brillet, J. Y. Chen, M. K. Wang and Y. Shen, Perovskite Solar Cell-TiO2@BiVO4 Photoelectrochemical System for Direct Solar Water Splitting, J. Mater. Chem. A, 2015, 3, 21630–21636 CAS.
- T. A. Kandiel, A. Y. Ahmed and D. Bahnemann, TiO2(B)/anatase heterostructure nanofibers decorated with anatase nanoparticles as efficient photocatalysts for methanol oxidation, J. Mol. Catal. A: Chem., 2016, 425, 55–60 CrossRef CAS.
- H. K. Jin, G. Magesh, H. J. Kang, M. Banu, H. K. Ju, J. Lee and J. S. Lee, Carbonate-coordinated cobalt co-catalyzed BiVO4/WO3 composite photoanode tailored for CO2 reduction to fuels, Nano Energy, 2015, 15, 153–163 CrossRef.
- Z. G. Yi, J. H. Ye, N. Kikugawa, T. Kako, S. Ouyang, H. Stuartwilliams, H. Yang, J. Cao, W. Luo and Z. Li, An orthophosphate semiconductor with photooxidation properties under visible-light irradiation, Nat. Mater., 2010, 9, 559–564 CrossRef CAS PubMed.
- Y. Bi, H. Hu, Z. Jiao, H. Yu, G. Lu and J. Ye, Two-dimensional dendritic Ag3PO4 nanostructures and their photocatalytic properties, PCCP, 2012, 14, 14486–14488 RSC.
- Y. Bi, H. Hu, S. Ouyang, G. Lu, J. Cao and J. Ye, Photocatalytic and photoelectric properties of cubic Ag3PO4 sub-microcrystals with sharp corners and edges, Cheminform, 2012, 43, 3748–3750 Search PubMed.
- W. Y. Lin, S. J. Zhang, D. Y. Wang, C. X. Zhang and D. L. Sun, Ultrasound-assisted synthesis of high-efficiency Ag3PO4/CeO2 heterojunction photocatalyst, Ceram. Int., 2015, 41, 8956–8963 CrossRef CAS.
- C. Wang, J. X. Zhu, X. Y. Wu, H. Xu, Y. H. Song, J. Yan, Y. X. Song, H. Y. Ji, K. Wang and H. M. Li, Photocatalytic degradation of bisphenol A and dye by graphene-oxide/Ag3PO4 composite under visible light irradiation, Ceram. Int., 2014, 40, 8061–8070 CrossRef CAS.
- U. Sulaeman, F. Febiyanto, S. Yin and T. Sato, The highly active saddle-like Ag3PO4 photocatalyst under visible light irradiation, Catal. Commun., 2016, 85, 22–25 CrossRef CAS.
- Q. Wu, P. F. Wang, F. T. Niu, C. P. Huang, Y. Li and W. F. Yao, A novel molecular sieve supporting material for enhancing activity and stability of Ag3PO4 photocatalyst, Appl. Surf. Sci., 2016, 378, 552–563 CrossRef CAS.
- S. S. Patil, D. R. Patil, S. K. Apte, M. V. Kulkarni, J. D. Ambekar, C. J. Park, S. W. Gosavi, S. S. Kolekar and B. B. Kale, Confinement of Ag3PO4 nanoparticles supported by surface plasmon resonance of Ag in glass: efficient nanoscale photocatalyst
for solar H2 production from waste H2S, Appl. Catal., B, 2016, 190, 75–84 CrossRef CAS.
- Q. H. Xiang, L. Di, T. T. Shen and L. Fan, Graphene-modified nanosized Ag3PO4 photocatalysts for enhanced visible-light photocatalytic activity and stability, Appl. Catal., B, 2015, 162, 196–203 CrossRef CAS.
- P. Amornpitoksuk and S. Suwanboon, Photocatalytic decolorization of methylene blue dye by Ag3PO4–AgX(X = Cl−, Br−and I−) under visible light, Adv. Powder Technol., 2014, 25, 1026–1030 CrossRef CAS.
- J. Cao, B. Luo, H. Lin, B. Xu and S. Chen, Visible light photocatalytic activity enhancement and mechanism of AgBr/Ag3PO4 hybrids for degradation of methyl orange, J. Hazard. Mater., 2012, 217–218, 107–115 CrossRef CAS PubMed.
- H. Katsumata, T. Hayashi, M. Taniguchi, T. Suzuki and S. Kaneco, AgI/Ag3PO4 hybrids with highly efficient visible-light driven photocatalytic activity, Mater. Res. Bull., 2015, 63, 116–122 CrossRef CAS.
- W. F. Yao, B. Zhang, C. P. Huang, C. Ma, X. L. Song and Q. J. Xu, Synthesis and characterization of high efficiency and stable Ag3PO4/TiO2 visible light photocatalyst for the degradation of methylene blue and rhodamine B solutions, J. Mater. Chem., 2012, 22, 4050–4055 RSC.
- C. N. Tang, E. Z. Liu, J. Fan, X. Y. Hu, L. M. Kang and J. Wan, Heterostructured Ag3PO4/TiO2 nano-sheet film with high efficiency for photodegradation of methylene blue, Ceram. Int., 2014, 40, 15447–15453 CrossRef CAS.
- Z. M. Yang, G. F. Huang, W. Q. Huang, J. M. Wei, X. G. Yan, Y. Y. Liu, C. Jiao, Z. Wan and A. Pan, Novel Ag3PO4/CeO2 composite with high efficiency and stability for photocatalytic applications, J. Mater. Chem. A, 2014, 2, 1750–1756 CAS.
- W. Y. Lin, S. J. Zhang, D. Y. Wang, C. X. Zhang and D. L. Sun, Ultrasound-assisted synthesis of high-efficiency Ag3PO4/CeO2 heterojunction photocatalyst, Ceram. Int., 2015, 41, 8956–8963 CrossRef CAS.
- H. J. Yu, Y. Yu, J. H. Liu, P. Y. Ma, Y. C. Wang, F. Zhang and Z. Y. Fu, Space-confined growth of Ag3PO4 nanoparticles within WS2 sheets: Ag3PO4/WS2 composites as visible-light-driven photocatalysts for decomposing dyes, J. Mater. Chem. A, 2015, 3, 19439–19444 CAS.
- F. Ding, S. Z. Zhang, X. G. Luo and X. Y. Lin, Fabrication of Ag3PO4/α-Bi2O3 composites with enhanced photocatalytic properties under visible light, RSC Adv., 2015, 5, 96685–96694 RSC.
- N. Mohaghegh, M. Tasviri, E. Rahimi and M. Gholami, A novel p–n junction Ag3PO4/BiPO4-based stabilized Pickering emulsion for highly efficient photocatalysis, RSC Adv., 2015, 12944–12955 RSC in press.
- Y. G. Lv, K. Huang, W. Zhang, B. Yang, F. L. Chi, S. L. Ran and X. G. Liu, One step synthesis of Ag/Ag3PO4/BiPO4 double-heterostructured nanocomposites with enhanced visible-light photocatalytic activity and stability, Ceram. Int., 2014, 40, 8087–8092 CrossRef CAS.
- S. Y. Wu, H. Zheng, Y. Y. Wu, W. Lin, T. Z. Xu and M. Guan, Hydrothermal synthesis and visible light photocatalytic activity enhancement of BiPO4/Ag3PO4 composites for degradation of typical dyes, Ceram. Int., 2014, 40, 14613–14620 CrossRef CAS.
- O. Mehraj, N. A. Mir, B. M. Pirzada and S. Sabir, Fabrication of novel Ag3PO4/BiOBr heterojunction with high stability and enhanced visible-light-driven photocatalytic activity, Appl. Surf. Sci., 2015, 332, 419–429 CrossRef CAS.
- S. Kumar, T. Surendar, A. Baruah and V. Shanker, Synthesis of a novel and stable
g-C3N4–Ag3PO4 hybrid nanocomposite photocatalyst and study of the photocatalytic activity under visible light irradiation, J. Mater. Chem. A, 2013, 1, 5333–5340 CAS.
- J. F. Zhang, J. L. Lv, K. Dai, Q. Liu, C. H. Liang and G. P. Zhu, Facile and green synthesis of novel porous g-C3N4/Ag3PO4 composite with enhanced visible light photocatalysis, Ceram. Int., 2016, 43, 1522–1529 CrossRef.
- L. Li, Y. H. Qi, J. R. Lu, S. L. Lin, W. J. An, Y. H. Liang and W. Q. Cui, A stable Ag3PO4@g-C3N4 hybrid core@shell composite with enhanced visible light photocatalytic degradation, Appl. Catal., B, 2015, 183, 133–141 CrossRef.
- T. Y. Huang, Y. J. Chen and C. Y. Lai, et al. Synthesis, characterization, enhanced sunlight photocatalytic properties, and stability of Ag/Ag3PO4 nanostructure- sensitized BiPO4[J], RSC Adv., 2015, 5, 43854–43862 RSC.
|
This journal is © The Royal Society of Chemistry 2017 |