DOI:
10.1039/C7RA00361G
(Paper)
RSC Adv., 2017,
7, 15455-15462
Three-dimensional electro-Fenton oxidation of N-heterocyclic compounds with a novel catalytic particle electrode: high activity, wide pH range and catalytic mechanism†
Received
10th January 2017
, Accepted 28th February 2017
First published on 8th March 2017
Abstract
A novel three-dimensional (3D) heterogeneous electro-Fenton (EF) system with sludge deserved activated carbon from sewage and iron sludge (SAC-Fe) as catalytic particle electrodes (CPEs) was constructed in this study. Its application in degrading nitrogen-heterocyclic compounds (NHCs) exhibited high catalytic efficiency over a wide applicable pH range from 3.0 to 9.0. SAC-Fe worked as both CPEs and a heterogeneous catalyst in this 3D EF system, enhancing oxidation activity. Degradation pathways of the NHCs (indole, quinoline and pyridine) and reasonable reaction mechanisms involved in this 3D EF were proposed. At pH 3.0, hydroxyl radicals were the dominant participant oxidants, following a Haber–Weiss mechanism. The FeII sites catalyzed the decomposition of electro-generated H2O2 to yield ˙OH. At pH 9.0, the oxidants generated from the decomposition of H2O2 were mainly ˙O2−/HO2˙ and to lesser extent were ˙OH. The formation and decomposition of H2O2 complex with catalytic sites (FeII and FeIII) as well as the catalytic decomposition of H2O2 were involved in the catalytic reactions to generate ˙O2−/HO2˙ and ˙OH. A quantitative structure–activity relationship analysis (QSAR) model was developed to describe the relationship between degradation properties of NHCs and their structures by involving quantum descriptors.
1. Introduction
Nitrogen-heterocyclic compounds (NHCs) are a group of environmentally significant contaminants, which mainly come from coal gasification, oil processing, coal-tar waste and creosote wood preservation.1 NHCs are generally water-soluble and NHCs are the main refractory organic pollutants residual in coal gasification wastewater.2 Many NHCs are highly toxic and some of them are even mutagenic and carcinogenic.3 Therefore, it is environmentally important to develop options to remove them economically and effectively.
Recently, advanced oxidation processes (AOPs) have been considered as potentially powerful methods and have been the subject of growing interest in wastewater treatment for removing the recalcitrant organics. As one of AOPs, electro-Fenton (EF) has drawn amazing attention as a promissory alternative technology by overcoming some intrinsic drawbacks of traditional Fenton process.4,5 EF employs electrochemical reactions to generate in situ Fenton reagents from eqn (1).6 Therefore, it is of great advantages since no H2O2 is needed. Since ˙OH production does not involve the use of harmful chemicals, this process is environmentally friendly.7–9 Solid catalyst containing iron species is introduced in the traditional EF to avoid pH adjustment, transforming the homogeneous process into a heterogeneous one.10,11 In this case, soluble Fe2+ is replaced by Fe-containing solids.12 Moreover, FeII could be regenerated rapidly from the reduction of FeIII at the cathode thanks to the enhancement of electron transfer, which was the limiting step in traditional Fenton process due to low rate constant of Fe3+ reduction reaction. However, there is a drawback of low efficiency in EF due to the limited cathode surface area. Three-dimensional electrode was proposed as a means of enlarging the electrode surface area by involving particle electrodes since the rate of electrochemical reactions are dependent on electron transfer rate, which is directly proportional to the specific surface area of the electrode.13 Recently, many studies focuses on the preparation of particle electrodes with high activity and stability to facilitate the application of 3D electrochemical system.14 Particle electrodes frequently used in 3D electrochemical reactions are dominantly carbonaceous material and metallic material. However, the complicated preparation process and expensive or unavailable raw materials restrict the development of such kind of particle electrodes.15 EF coupled with three-dimensional electrode could overcome the problem of low current efficiency, as well as give full play to the advantages of both technologies. Catalytic particle electrodes (CPEs) integrated catalyst and particle electrode, and CPEs served both catalyst and particle electrode in 3D EF system. Therefore, it is of great importance to prepare efficient and stable CPEs with available and cost-effective raw materials, which is beneficial to full scale application.16 The integration of EF and three-dimensional electrode would be bound to induce the acceleration of electrochemical reactions and the improvement of treatment efficiency.
|
O2(g) + 2H+ + 2e− → H2O2
| (1) |
In the current study, SAC-Fe was developed from sewage sludge and iron sludge and served as CPEs to form three-dimensional electrochemical system. SAC-Fe also worked as heterogeneous catalyst in EF to catalyze the decomposition of electro-generated H2O2. The corresponding reaction mechanisms at acid and basic conditions were investigated through the role of participant oxidants. Three NHCs were degraded in this 3D EF and the degradation pathways were proposed. Additionally, the relationship between the activity and the structure was explored by the analysis of quantitative structure–activity relationship (QSAR).
2. Materials and methods
2.1. Chemicals
The NHCs were purchased from Yongda Chemical Reagent Company Ltd (Tianjin, China). HPLC grade methanol and acetic acid were obtained from Fisher Scientific Company (Fair Lawn, USA). All chemicals were analytical grade reagents and were used directly without further purification. All the reaction mediums were deionized water.
2.2. Preparation and characterization of CPEs
SAC-Fe was composed of sewage sludge and iron sludge, prepared via a one-step method combining the carbonization and Fe-loading process. The facile synthesis process was as follows: first, the dewatered sewage sludge and iron sludge were dried at 105 °C for 24 h, and then ground and sieved into a uniform size of less than 200 mesh. 20 g mixture of sewage sludge and iron sludge samples according to a certain proportion was added into a 150 mL of 3 mol L−1 ZnCl2 solution as activation agent (sludge
:
ZnCl2 = 1
:
3, by mass). The suspension was stirred for 24 h slowly at ambient temperature, evaporated in a rotary evaporator at 60 °C and dried at 105 °C. Subsequently, the dried solid was pyrolyzed under flowing nitrogen (100 mL min−1) in a horizontal quartz furnace at 700 °C for 2 h at a rate of 20 °C min−1. The pyrolyzed samples were washed with 3.0 mol L−1 HCl to rinse the inorganic impurities followed by thoroughly washed with deionized water several times until pH of rinsed water became constant to remove the loosely bonded metal irons. Sewage sludge derived activated carbon (SAC) was prepared without adding iron sludge. Finally the samples were dried at 105 °C and stored in desiccators prior to use.
The main physicochemical properties of CPEs are listed in Table S1.† SAC-Fe and SAC exhibited a high specific surface area more than 350 m2 g−1 and showed mesoporous character (Fig. S1†). The main catalytic component of SAC-Fe was Fe3O4 according to the XRD and XPS analysis (Fig. S2 & S3†), providing catalytic sites in EF reaction. The FTIR spectra (Fig. S4, detailed information in ESI†) indicated that chemical bonds between the inorganic compound (SiO2) in the sewage sludge and the loaded Fe compound were formed during the carbonization process. The iron oxide was impregnated in the carbon matrix, which was beneficial from the pre-mixture of sewage sludge and iron sludge (iron source). The formation of chemical bands between Fe and carbon matrix, carbonaceous texture, high Fe content and large specific surface area of the as-synthesized SAC-Fe sample guarantee the potential to act as a stable and efficient CPEs and heterogeneous catalyst for the 3D EF system. (More information on the preparation and characterization of SAC-Fe could be obtained in our previous study.16)
2.3. Experimental procedures
Degradation experiments were conducted in a one-compartment electrochemical cell (1.0 L). The anode was a Pt sheet (4 cm × 5 cm × 0.3 cm) and the cathode was a piece of activated carbon fiber (ACF) with the same dimension. The gap between the electrodes was 4 cm. In a typical run, the as-synthesized CPEs (SAC-Fe and SAC) were filled into NHCs solutions. Then the vessels were shaken for 48 h to ensure the adsorption equilibrium. After equilibrium, the adsorption saturated CPEs were transferred to electrolysis cell. The degradation reaction was initialed by switching on the DC current. Current density and was optimized and maintained at 10 mA cm−2, and CPEs dosage was set to be 5.0 g L−1 (ESI†). Air was bubbled from the bottom of the reactor (4.0 L min−1) to provide oxygen and generate stirring in the solution. The reaction temperature was kept at 25 °C by a thermostatic bath. NHCs were degraded in an aqueous medium containing 0.05 M Na2SO4 as supporting electrolyte. The initial concentrations of the three NHCs were 20 mg L−1, according to the water quality analysis of coal chemical industry wastewater. Samples were withdrawn at pre-selected time intervals followed by filtered with 0.45 μm filter paper. Then the samples were stored at 4 °C for further analysis.
2.4. Analytical methods
NHCs were measured by HPLC equipped with Agilent AQ-C18 column (4.6 mm × 150 mm × 5 μm) and selected photodiode detector. The mobile phase was a mixture of methanol and acetic acid with a flow rate of 1.0 mL min−1. Total organic carbon (TOC) was measured with a TOC Analyzer (TOC-CPN, Shimadzu, Japan). For intermediates identification, samples were analyzed by GC-MS followed by extraction with CH2Cl2 and concentrated processes. The organics were determined with an Agilent 6890N gas chromatograph interfaced with a 5973C mass selective detector (MSD) and equipped with an Agilent 7683B auto sampler and a DB5-MS capillary column. Helium was used as the carrier gas with a flow rate of 1.0 mL min−1. Hydrogen peroxide generated in the solution was measured with iodide method.17 The generation of hydroxyl radicals (˙OH) was monitored by means of terephthalic acid (TA) fluorescent probe method on RF-6500 fluorescence spectrometer.18
3. Results and discussion
3.1. Catalytic performance of 3D EF over wide pH ranges
Fig. 1a illustrates the degradation of NHCs (indole, quinoline and pyridine) in 3D EF with SAC-Fe as CPEs at optimal pH 3.0. NHCs were rapidly degraded in this 3D EF system. The removal efficiencies of indole, quinoline and pyridine achieved 99.1%, 96.7% and 64.2% within 120 min, with the final concentrations of 0.18, 0.66 and 7.16 mg L−1 respectively. The oxidation behavior in 3D EF with the addition of SAC-Fe benefited from not only the properties of iron oxide embedded in SAC-Fe but also by the porous texture of the material. The high degradation efficiencies of NHCs were mostly due to the excellent characteristics of adsorption, conductivity and catalysis of SAC-Fe with extremely high surface area of 351.6 m2 g−1, which can efficiently generate H2O2 during electrochemical process and catalytically decompose H2O2 to powerful radicals. Microporosity strongly favored the pollutants adsorption capacity and tended to reduce the metal leaching, while mesoporosity strongly increased the metal dispersion.12,19 That is why SAC-Fe exhibited efficient catalytic activity in 3D EF. In addition, the kinetic analysis was conducted for NHCs degradation in the 3D EF system. Since the establishment of Fenton system proceeded in the initial period of EF, the degradation process after 20 min was separated for kinetic analysis. The degradations of NHCs were observed to approximately follow a pseudo-first-order kinetic model. The apparent rate constants were determined to be 0.0447, 0.0317 and 0.0097 min−1 for indole, quinoline and pyridine degradations.
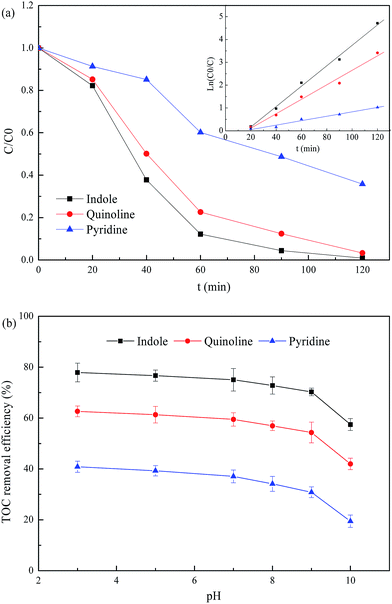 |
| Fig. 1 Catalytic degradation of NHCs and effect of pH on degradation performance in 3D EF. | |
TOC was measured to indicate the organic mineralization. TOC removals of NHCs degradation in the 3D EF at a wide pH range of 3.0–10.0 were illustrated in Fig. 1b. TOC removal efficiencies of NHCs degradation decreased slightly (∼8%) when increasing pH from 3.0 to 9.0. While a quick decline of TOC removals (∼12%) were observed when further raising pH to 10.0. The reasons are mostly due to the fast decomposition of H2O2 into H2O and O2 at strong basic conditions as well as the decreasing of the adsorption of H2O2 on the catalyst covered with FeIII complexes.20 In addition, the oxidation power of radicals weakened as increasing alkalinity. It was demonstrated that the 3D EF with SAC-Fe as CPEs was efficient within wide pH ranges from 3.0 to 9.0 without sacrificing efficiency.
3.2. Reaction mechanism
In order to understand the promotion effect of the prepared CPEs in 3D EF, amounts of electro-generated H2O2 were measured. It was determined that the corresponding amount of H2O2 was 442.7 μmol L−1 (60 min) with SAC as CPEs, much higher than that (259.9 μmol L−1) in 2D system (without CPEs), which demonstrated that the high efficient pollutants removals in 3D EF were mainly related to the more amount of H2O2 generation. Every pair of CPEs formed a micro-electrolysis cell with the addition of CPEs, transforming it into 3D system. The electrode area and active sites to produce H2O2 increased multiply, inducing the rise of H2O2 production accordingly.
Since ˙OH is crucial for understanding the efficiency of EF, amount of ˙OH in this efficient 3D EF system was detected with TA at different pH values. As can be seen from Fig. 2, amount of ˙OH declined notably with extending pH from 3.0 to 9.0. More ˙OH were produced in acid solution than basic condition. However, insignificant decline of TOC removal was observed when increasing pH from 3.0 to 9.0. This observation indicated that the oxidation mechanism of 3D EF with SAC-Fe addition in acid and basic solution would be different. Therefore, participant oxidants generated during the decomposition of H2O2 by SAC-Fe in acid and basic solution were deeply studied as follows.
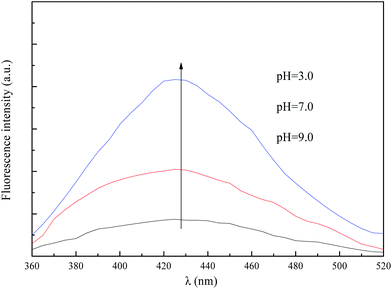 |
| Fig. 2 Fluorescence test for hydroxyl radicals measurement. | |
Moreover, ˙OH and/or superoxide anion (˙O2−) were the main oxidants generated from the decomposition of H2O2. The superoxide anion was the conjugate base of hydroperoxyl radicals (HO2˙) (HO2˙ ↔ H+ + ˙O2−). Herein, radical scavengers were employed to investigate the possible role of ˙OH and ˙O2−. Isopropanol (i-PrOH) has been described as the best ˙OH quencher due to its high rate constant reaction with ˙OH (1.9 × 109 M−1 s−1),17,21 while benzoquinone (BQ) is known for possessing high ability to trap superoxide anions by a simple electron transfer mechanism.22,23 As shown in Fig. 3a, higher inhibition rate was observed in TOC removal at pH 3.0 when i-PrOH was added than BQ. e.g. TOC removal efficiency declined 53.62% and 4.37% for indole degradation when radical scavengers of i-PrOH and BQ were added to acid solution at pH 3.0. The significant inhibitory effect of isopropanol suggested that ˙OH presented as main participation under acid conditions. Fig. 3b depicted the influences of radical scavengers on NCHs degradation at pH 9.0. 25.08% and 37.04% inhibition were observed in TOC removal for indole degradation as the addition of i-PrOH and BQ. The results categorically evidenced that different radicals were the main participant oxidants under acid and basic condition. Hydroxyl radical was dominant participant oxidant in acid solution and oxidants generated in 3D EF under basic condition were mainly ˙O2−/HO2˙ and to lesser extent were ˙OH.
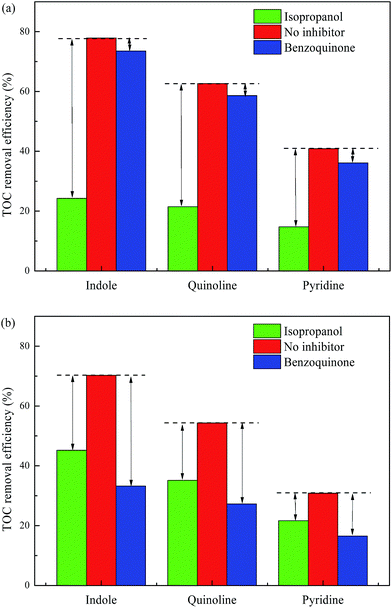 |
| Fig. 3 Effects of scavengers on NHCs degradations ((a) pH = 3.0, (b) pH = 9.0). | |
Since the catalytic site of Fenton reaction was immobilized on the solid CPEs, decomposition of H2O2 was almost completely surface-catalyzed process (stability and reusability tests of SAC-Fe were shown in the ESI†). Based on the above study, the possible reaction mechanism involved in 3D EF with the prepared CPEs was proposed and as depicted in Fig. 4. The reaction was possibility initialed by the carbon adsorption. Firstly an abundance of active O2 and pollutants were rapidly diffused toward the CPEs surface and enriched in diverse size pores of CPEs and kept a high local concentration due to the efficient adsorption performance. Then, active O2 was reduced to H2O2 in situ via two-electron reduction on the interface between CPEs and the bulk phase. It was reported that heterogeneous Fenton reaction began with the adsorption of H2O2 molecules on the hydrated catalyst.24
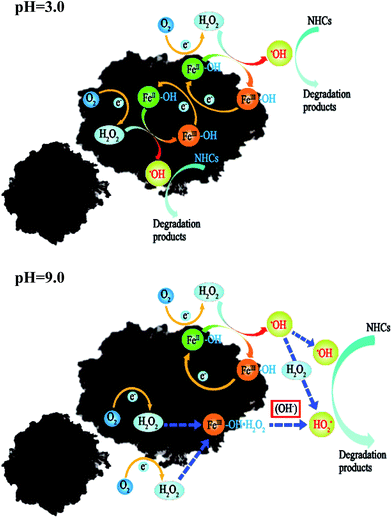 |
| Fig. 4 Schematic illustration of 3D EF reaction mechanisms with SAC-Fe in acidic and basic condition. | |
In acidic solution, once H2O2 molecules were generated on the CPEs, it would be catalytically decomposed to strong oxidizer ˙OH radicals by embedded iron oxide on the surface and internal of the CPEs promptly, presumably through a Haber–Weiss mechanism. Finally, the NHCs were degraded even mineralized by the generated ˙OH.
In basic solution, the interaction of H2O2 with the catalytic sites (FeIII and FeII) on the SAC-Fe surface was expected.22 Based on the above analysis, a surface-based mechanism different from in acidic condition was proposed. The electro-generated H2O2 interacted with surface iron species (FeIII–OH and FeII–OH) of the hydrated surface. For FeII–OH, it reacted with H2O2 to FeIII–OH and ˙OH (eqn (2) and (3)). Meanwhile, most of generated ˙OH in first stage would be scavenged by considerable H2O2 at the immediate vicinity (eqn (4)). For FeIII–OH, it interacted with H2O2 to form a precursor surface complex of H2O2 (eqn (5)). The surface H2O2 complex may further undergo a reversible ground-state electron transfer from ligand to metal (eqn (6)), and then the successor complex deactivated via eqn (7) to release HO2˙ oxidants.22,25 It was noted that reaction (6) would be more active in basic solution since hydroxyl ions can react with the formed protons from reaction (5) promptly, promoting the forward reaction. The FeII–OH and FeIII–OH recycled via reaction (3), (6) and (7). That is why participant oxidants generated in 3D EF at pH 9.0 were mainly HO2˙ and to lesser extent were ˙OH.
|
FeII–OH + H2O2 → FeII–OH˙H2O2(ads)
| (2) |
|
FeII–OH˙H2O2(ads) → FeIII–OH + ˙OH + OH−
| (3) |
|
 | (4) |
|
FeIII–OH + H2O2 → FeIII–OH˙H2O2(ads)
| (5) |
|
 | (6) |
|
 | (7) |
|
 | (8) |
The formed radicals then reacted with the contaminants and degraded those compounds into intermediates or complete mineralization (eqn (8)). Since the contaminants were enriched on the CPEs resulting from adsorption effect and maintained at high concentration, these pollutants in situ captured radicals and improved radicals utilization.
Abundant iron species embedded in porous CPEs with high exposure endowed more catalytic active sites. Fe species were impregnated in the carbon matrix obtained from the pyrolysis process, preventing the iron from aggregating into clusters and exhibiting better decentrality both on the surface and internally in the CPEs, which resulted in the improvement in the number and properties of the reactive surface sites to promote radicals production. Thus, the efficient degradation of NHCs was realized.
3.3. Degradation pathway
The changes of UV-Vis spectra in the degradations of NHCs were illustrated in Fig. 5. A continuous decrease at 256 nm was observed for pyridine degradation (Fig. 5a), indicating the decomposition of pyridine. For quinoline degradation, the adsorption peak at 313 nm declined rapidly in 60 min, demonstrating the fast degradation of quinoline (Fig. 5b). The peak appeared at around 256 nm suggested that intermediates analogous to the structure of pyridine were formed in the degradation of quinoline. A quick decline at 270 nm was found in indole degradation (Fig. 5c). Other peaks emerged at around 245–260 nm and 304 nm evidenced the generation of considerable intermediates. The main intermediates in the degradations of NHCs were listed in Table 1. Pyridine is one kind of NHCs lack of π electron. In the structure of pyridine, the pair of electrons at the nitrogen atom does not conjugate with the ring, and the nitrogen atom attracts electrons and deactivates the ring, since the electron cloud density of the ring is low resulted from the attraction of nitrogen atom.26 The cloud density of the meta carbon atom is higher than others, and the meta carbon atom is prone to electrophile substitution reaction. In the structure of quinoline, the isolated pair of electrons at the nitrogen atom does not conjugate with the heterocyclic ring, inducing the higher cloud density on the benzene ring. This structure reduces the reactivity of the heterocyclic ring to electrophile reagent and enhances the reactivity of the benzene ring instead. The electrophile attack occurs readily on the benzene ring. The pyrrole ring of indole is super π electron structure, causing the high reactivity of the pyrrole ring due to its high cloud density. Based on the structure analysis of NHCs and the intermediates detections, the degradation pathways of NHCs by the 3D EF oxidation were proposed in Fig. 6.
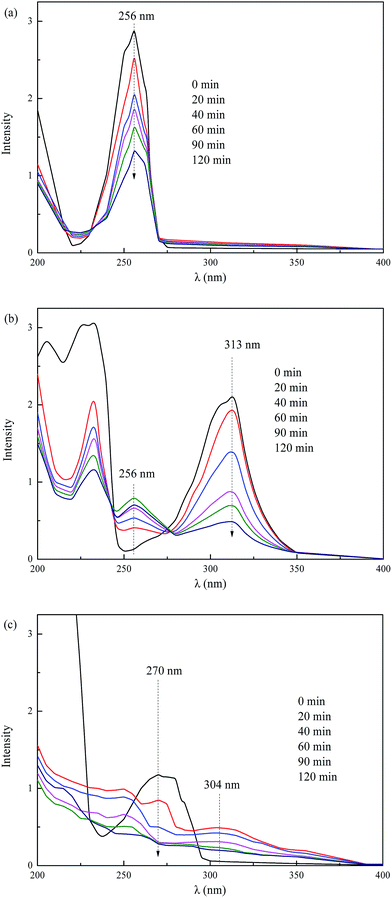 |
| Fig. 5 Variations of UV-Via spectra in the degradation of NHCs. ((a) Pyridine, (b) quinoline, (c) indole.) | |
Table 1 Observed organic intermediates in NHCs degradations
NHCs |
Observed intermediates |
Pyridine |
3-Hydroxypyridine, 2,3-dihydroxypyridine, 2(2H)-pyridinone-one, 2N hexadiene dicarboxaldehyde, succinate |
Quinoline |
5-Hydroxyquinoline, 8-hydroxyquinoline, 5,8-dihydroxyquinoline, pyridine-2,3-diglyoxylate acid, pyridine-2,3-dicarboxaldehyde, pyridine-2,3-dicarboxylic acid, pyridine-2-carboxyl-3-carboxaldehyde, pyridine-3-carboxyl-2-carboxaldehyde, 3-carboxypyridine, 2-carboxypyridine, succinate, formic acid |
Indole |
Isatin, o-aminobenzoic acid, oxalic acid, propionic acid, acetic acid |
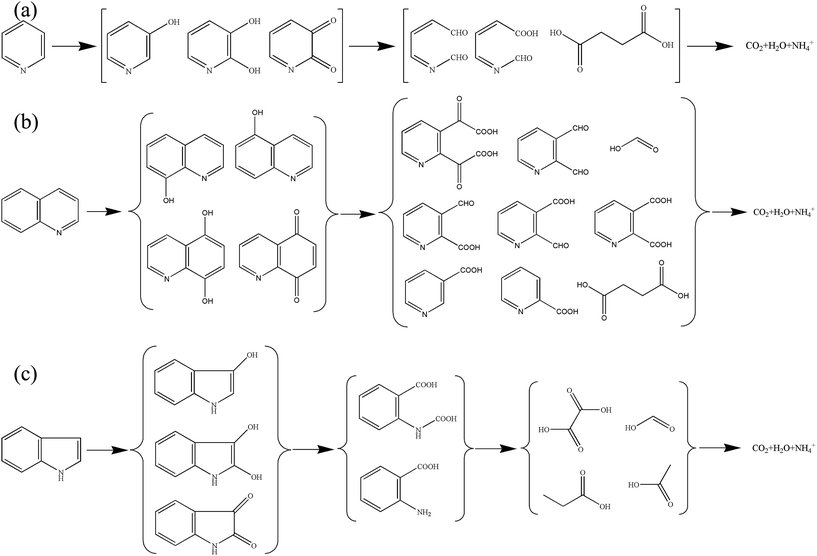 |
| Fig. 6 Possible degradation pathways of the three NHCs. (a) Pyridine, (b) quinoline, (c) indole. | |
3.4. QSAR
Significant differences were observed in the degradations of the above three NHCs. Compound structure determines the degradation properties, so it was meaningful to explore the relationship between the degradation rates and the pollutant structure. The pseudo-first-order rate constants of the NHCs were calculated in Section 3.1 (Fig. 1). The degradation rates of the three NHCs by 3D EF in decline order were indole > quinoline > pyridine. In order to explore the relationship between the structure and activity, a QSAR analysis was performed. The quantum descriptors used were EHOMO, ELUMO and ELUMO − EHOMO (Table 2). These descriptors reflect the character of oxidation/reduction of the compounds.27,28 The QSAR model was obtained from the stepwise variance regression of ln
ka and the descriptors (eqn (9)). The polynomial regression model produced high squared correlation coefficient (R2 = 0.9947), corroborating the high precision and reliability of the developed QSAR model to describe the relationship between the degradation properties and the compound structures. An increase of ELUMO − EHOMO leaded to a decrease of degradation rate, and the low EHOMO had adverse effect on the degradation. The compound with a high value of ELUMO − EHOMO implies that electrophile attack is restricted.29,30 The attack of ˙OH is electrophile reaction. For example, ELUMO − EHOMO of pyridine is highest among these NHCs, so the electrophile attack takes place difficultly. The developed QSAR model was validated by the degradation of isoquinoline. The apparent rate constants of isoquinoline degraded by 3D EF and calculated from the model were 0.0372 and 0.0383 min−1 respectively, further evidencing the accuracy of the model. The results provided an insight to the relationship between the degradation of the NHCs and their structures. |
ln ka = −0.64102 × (ELUMO − EHOMO) − 0.38264 × ELUMO + 2.42808
| (9) |
Table 2 Theoretical descriptors of NHCs (eV)
NHCs |
EHOMO |
ELUMO |
ELUMO − EHOMO |
Pyridine |
−10.036 |
0.609 |
10.645 |
Quinoline |
−9.003 |
0.145 |
9.148 |
Indole |
−8.355 |
0.173 |
8.528 |
Isoquinoline |
−8.825 |
0.032 |
8.857 |
4. Conclusions
This study reported a three-dimensional heterogeneous electro-Fenton system with SAC-Fe as CPEs. This 3D EF system worked very well over a wide pH ranges from 3.0 to 9.0 without sacrificing efficiency. SAC-Fe worked not only as CPEs for efficiently in situ electro-generating H2O2, but also as heterogeneous catalyst for catalytically decomposing H2O2 to produce radicals. The corresponding reaction mechanisms of the 3D EF on the CPEs at different pH ranges were proposed by employing radicals scavengers. In acidic solution, ˙OH was the dominant participant oxidant, following a Haber–Weiss mechanism. FeII sites on SAC-Fe catalyzed the decomposition of H2O2 to generate ˙OH. While in basic solution, the oxidants generated from the decomposition of H2O2 were mainly ˙O2−/HO2˙ and to lesser extent were ˙OH. The formation and decomposition of H2O2 complex with catalytic sites (FeII and FeIII) as well as the catalytic decomposition of H2O2 were involved in the catalytic reactions to generate ˙O2−/HO2˙ and ˙OH. Possible degradation pathways of NHCs were proposed as well. A QSAR model was developed to describe the relationship between degradation properties of NHCs and their structures. This promising 3D EF has great development potential for practical wastewater treatment since its high efficiency and friendly working condition especially at neutral pH. And the understanding of the catalytic oxidation mechanism may lead to the development of more efficient CPEs and optimization of the process parameters.
Acknowledgements
This work was supported by the National Natural Science Foundation of China (No. 41472328 and No. 41672350) and the scientific research project of Hunan Provincial Education Department (No. 15C0556).
References
- F. Sun, Q. Yu, J. Zhu, L. Lei, Z. Li and X. Zhang, Chemosphere, 2015, 134, 402–407 CrossRef CAS PubMed.
- P. Xu, H. Han, H. Zhuang, B. Hou, S. Jia, D. Wang, K. Li and Q. Zhao, J. Environ. Sci., 2015, 31, 221–225 CrossRef PubMed.
- P. Liao, S. Yuan, W. Xie, W. Zhang, M. Tong and K. Wang, J. Colloid Interface Sci., 2013, 390, 189–195 CrossRef CAS PubMed.
- B. Hou, W. Ma, H. Han, P. Xu, H. Zhuang and K. Li, Desalin. Water Treat., 2016, 57, 8174–8182 CrossRef CAS.
- H. Y. Zhao, L. Qian, X. H. Guan, D. L. Wu and G. H. Zhao, Environ. Sci. Technol., 2016, 50, 5225–5233 CrossRef CAS PubMed.
- E. Bocos, M. Pazos and M. A. Sanroman, RSC Adv., 2016, 6, 1958–1965 RSC.
- A. Ozcan, Y. Sahin and M. A. Oturan, Water Res., 2013, 47, 1470–1479 CrossRef CAS PubMed.
- W. R. P. Barros, P. C. Franco, J. R. Steter, R. S. Rocha and M. R. V. Lanza, J. Electroanal. Chem., 2014, 722, 46–53 CrossRef.
- E. Alfaya, O. Iglesias, M. Pazos and M. A. Sanroman, RSC Adv., 2015, 5, 14416–14424 RSC.
- E. G. Garrido-Ramirez, M. L. Mora, J. F. Marco and M. S. Ureta-Zanartu, Appl. Clay Sci., 2013, 86, 153–161 CrossRef CAS.
- Y. B. Wang, H. Y. Zhao and G. H. Zhao, Appl. Catal., B, 2015, 164, 396–406 CrossRef CAS.
- L. Bounab, O. Iglesias, E. Gonzalez-Romero, M. Pazos and M. A. Sanroman, RSC Adv., 2015, 5, 31049–31056 RSC.
- C. H. Zhang, H. Lin, J. Chen and W. W. Zhang, Environ. Technol., 2013, 34, 2371–2376 CrossRef CAS PubMed.
- B. Hou, H. Han, H. Zhuang, P. Xu, S. Jia and K. Li, Bioresour. Technol., 2015, 196, 721–725 CrossRef CAS PubMed.
- Z. Y. Wang, J. Y. Qi, Y. Feng, K. Li and X. Li, J. Ind. Eng. Chem., 2014, 20, 3672–3677 CrossRef CAS.
- B. Hou, H. Han, S. Jia, H. Zhuang, P. Xu and K. Li, J. Taiwan Inst. Chem. Eng., 2016, 60, 352–360 CrossRef CAS.
- S. Qiu, D. He, J. X. Ma, T. X. Liu and T. D. Waite, Electrochim. Acta, 2015, 176, 51–58 CrossRef CAS.
- X. W. Cheng, Q. F. Cheng, X. Y. Deng, P. Wang and H. L. Liu, Electrochim. Acta, 2015, 184, 264–275 CrossRef CAS.
- H. Y. Zhao, Y. Chen, Q. S. Peng, Q. N. Wang and G. H. Zhao, Appl. Catal., B, 2017, 203, 127–137 CrossRef CAS.
- Y. Wang, Y. K. Sun, W. G. Li, W. D. Tian and A. Irini, Chem. Eng. J., 2015, 267, 1–8 CrossRef CAS.
- L. Lyu, L. Zhang and C. Hu, Chem. Eng. J., 2015, 274, 298–306 CrossRef.
- W. G. Li, Y. Wang and A. Irini, Chem. Eng. J., 2014, 244, 1–8 CrossRef CAS.
- O. Scialdone, A. Galia, C. Gattuso, S. Sabatino and B. Schiavo, Electrochim. Acta, 2015, 182, 775–780 CrossRef CAS.
- L. Gu, N. W. Zhu, H. Q. Guo, S. Q. Huang, Z. Y. Lou and H. P. Yuan, J. Hazard. Mater., 2013, 246, 145–153 CrossRef PubMed.
- L. Gu, N. W. Zhu and P. Zhou, Bioresour. Technol., 2012, 118, 638–642 CrossRef CAS PubMed.
- H. Zhao, S. H. Xu, J. B. Zhong and X. H. Bao, Catal. Today, 2004, 93–5, 857–861 CrossRef.
- H. Kusic, B. Rasulev, D. Leszczynska, J. Leszczynski and N. Koprivanac, Chemosphere, 2009, 75, 1128–1134 CrossRef CAS PubMed.
- H. C. Zhu, Z. M. Shen, Q. L. Tang, W. C. Ji and L. J. Jia, Chem. Eng. J., 2014, 255, 431–436 CrossRef CAS.
- L. J. Jia, Z. M. Shen, W. M. Guo, Y. A. Zhang, H. C. Zhu, W. C. Ji and M. H. Fan, J. Taiwan Inst. Chem. Eng., 2015, 46, 140–147 CrossRef CAS.
- H. C. Zhu, W. M. Guo, Z. M. Shen, Q. L. Tang, W. C. Ji and L. J. Jia, Chemosphere, 2015, 119, 65–71 CrossRef CAS PubMed.
Footnote |
† Electronic supplementary information (ESI) available. See DOI: 10.1039/c7ra00361g |
|
This journal is © The Royal Society of Chemistry 2017 |