DOI:
10.1039/C6RA28634H
(Paper)
RSC Adv., 2017,
7, 21476-21483
Facile fabrication of a magnetic self-healing poly(vinyl alcohol) composite hydrogel
Received
23rd December 2016
, Accepted 10th April 2017
First published on 18th April 2017
Abstract
This study proposes a simple method to fabricate a magnetic self-healing poly(vinyl alcohol) (ms-PVA) composite hydrogel. The obtained ms-PVA hydrogel was characterized by vibrating sample magnetometery and transmission electron microscopy. The self-healing property was investigated using a rheometer and tensile strength tests. Results showed that the ms-PVA hydrogel exhibited an excellent self-healing property and controllable magnetic intensity by adjusting the Fe3O4 particle content. The added Fe3O4 particles did not participate in hydrogen bond interactions but did cause the magnetic properties of the hydrogel. The hydrogel showed the best self-healing performance when the amount of Fe3O4 particles was 5 wt% (based on ms-PVA). The ms-PVA composite hydrogel presents a new application of the PVA hydrogel given its facile fabrication process and excellent performance.
1. Introduction
Hydrogels are 3D crosslinked polymeric networks that immobilize large quantities of water (20% to up to a thousand times their dry weight) and remain insoluble because of the presence of crosslinking,1,2 and have been widely applied in a variety of fields, such as biomaterials in wound dressings,3 implants and soft contact lenses,4 and microspheres for controlled drug release.5 However, with the development of functional materials, common hydrogels still cannot meet some specific needs. As a result, functional hydrogels are currently a hot research topic, and a large number of functional hydrogels have been developed,6–9 such as ternary interpenetrating microvascular networks,10 metallo-hydrogels,11 and peptide based hydrogels.12
Magnetic hydrogels are a type of promising functional hydrogel with magnetic properties and can be prepared by two main methods:13,14 one is to blend pre-synthesized magnetic iron oxide nanoparticles (MIONs) with hydrogels in solution and to crosslink the MIONs with the polymer, the other is in situ generation of MIONs in the preparation of hydrogels. Magnetic hydrogels have been widely used in biological fields such as magnetic resonance imaging,15 magnetically targeted hyperthermia,16 drug delivery,17,18 and immobilization of proteins,19 which have intrinsic magnetic property and favorable biocompatibility. However, magnetic hydrogel is easily damaged during its application, which may affect the normal use of related products and lead to a short service life.20 Therefore, developing a new types of magnetic hydrogels that automatically heal is needed.
The self-healing concept is derived from the self-healing ability of biology. The human skin could self-heal and regenerate after injury. A material that possesses the self-healing properties can significantly prolong service life and save repair cost.21 However, traditional hydrogel does not possess self-healing properties. Many researchers have reported the preparation of self-healing hydrogels,22,23 which have widely attracted the interests of scientists in designing the internal structure of hydrogel with reversible covalent bonding systems (Diels Alder chemistry24 and acylhydrazone bonds25) and non-covalent bonds (π–π stacking,26 hydrogen bond,27,28 electrostatic interactions,29,30 and hydrophobic interactions31,32). Zhang et al.33 determined that poly(vinyl alcohol) (PVA) hydrogel prepared using the freezing thawing method could self-repair at room temperature without a stimulus or healing agent. The hydrogel exhibited the highest fracture stress reported so far (approximately 200 kPa). Zhang first reported a straightforward method to prepare a novel magnetic hydrogel with healing ability by facilely mixing carboxy-modified Fe3O4 nanoparticles in a dynamic hydrogel composed of chitosan and telechelic difunctional poly(ethylene glycol).20 The results indicated that introducing the self-healing function into magnetic hydrogel was meaningful.
Thus, this study proposes a facile method to prepare magnetic hydrogel with self-healing ability by facilely mixing Fe3O4 particles in the self-healing PVA hydrogel. The effects of Fe3O4 particle content on the self-healing ability, mechanical and swelling, and magnetic properties of the PVA hydrogels are investigated.
2. Experimental
2.1 Materials and methods
Poly(vinyl alcohol) (PVA, Mw = 240 kDa), iron(III) chloride hexahydrate (FeCl3·6H2O) powder, iron(II) ferrous sulfate (FeSO4·7H2O) and ammonia solution (25 wt%) were purchased from Aladdin Chemistry Co. All other reagents were analytical grade and used as received without further purification. Deionized water was used throughout the experiments. The crystal structures of Fe3O4 were characterized using an X-ray diffractometer (XRD, PANalytical. B.V.) at a scanning range 2θ of 10° to 90° and rad of 0.04°. The tensile strength was tested on a universal testing machine (DCS-5000, Shimadzu) according to ISO 527-2:1993 at a constant crosshead speed of 100 mm min−1. The magnetic moment was recorded at 300 K on an MPMS XL-7 vibrating-sample magnetometer (VSM). The thermal decomposition behavior of the polymers was examined by thermogravimetry with a heating rate of 10 K min−1 in nitrogen atmosphere on a model TA Q500 (USA). Rheological date were obtained from an AR1500EX strain-controlled rheometer using a 25 mm parallel plate geometry at 25 °C and analyzed with the rheology advantage data analysis software. Transmission electron microscope (TEM, JEM-2100F from JEOL) with an accelerating voltage of 200 kV was used. The samples were thin-sectioned after drying at room temperature for 3 d.
The swelling ratio (S) of ms-PVA hydrogels was calculated as follows:
where
W0 and
Wt are the mass of the dried ms-PVA composite hydrogels before and after immersion in water, respectively.
2.2 Synthesis of magnetic Fe3O4 particles
The Fe3O4 nanoparticles are prepared as follows: first, 12.5 g (0.04 mol) of FeSO4·7H2O and 21.7 g (0.08 mol) of FeCl3·6H2O were dissolved in 50 mL distilled water. Then, ammonia solution (50 mL) was added to the solution with a stirring speed of 1000 rpm under the protection of dry nitrogen at 40 °C to form a homogeneous solution. The color of the solution changed from light brown to black after mixing the solution, which indicated the formation of Fe3O4 nanoparticles. This process was maintained for 30 min, and the temperature was increased to 80 °C to crystallize completely for another 60 min. The precipitate Fe3O4 particles were washed by repeating cycles of centrifugation and redispersion in distilled water. Washing was performed five times in distilled water. The final products were dried in a vacuum oven at 60 °C for 24 h, and the Fe3O4 nanoparticles were finally obtained.
2.3 Synthesis of magnetic self-healing poly(vinyl alcohol) (ms-PVA) composite hydrogels
A series of ms-PVA samples was prepared by adjusting the feed amounts of Fe3O4 nanoparticles to investigate the effect of Fe3O4 nanoparticle content on the properties of ms-PVA composite gels (see Table 1). In a typical procedure, 30 g PVA was dissolved in 100 mL distilled water at 95 °C with a stirring speed of 25 rpm for 30 min. A certain amount of Fe3O4 particles was added, and the solution was stirred for 1 h. Then, the homogeneous solution was into a mold of the desired dimension and cooled at −25 °C for 1 h, followed by thawing at room temperature for 1 h. Finally, the ms-PVA composite hydrogels were obtained. Unless otherwise stated, the hydrogel used in this work was prepared with a PVA concentration of 30 wt% and subjected to one freezing thawing cycle.
Table 1 Preparation of magnetic self-heal PVA composite hydrogels
Sample |
PVA (g) |
Fe3O4 (g) |
H2O (g) |
ms-PVA-1 |
30 |
0.75 |
100 |
ms-PVA-2 |
30 |
1.50 |
100 |
ms-PVA-3 |
30 |
2.25 |
100 |
ms-PVA-4 |
30 |
3.00 |
100 |
3. Results and discussion
3.1 Preparation of Fe3O4 particles
The optical microscope image of Fe3O4 particles dispersed in water is presented in Fig. 1. Fig. 1 shows that magnetic Fe3O4 particles were dispersed uniformly with an average particle size of 1 μm to 5 μm, and a few particles were connected. Fig. 2 shows the XRD pattern of the sample. Six characteristic peaks for Fe3O4 (2θ = 30.1, 35.5, 43.3, 53.4, 57.2 and 62.5) were observed, which indicates the successful preparation of the magnetic Fe3O4 particles.34
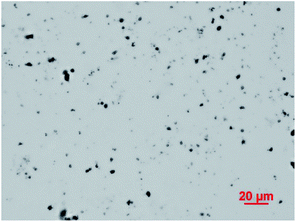 |
| Fig. 1 The optical microscope image of Fe3O4 particles. | |
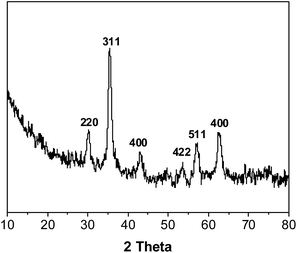 |
| Fig. 2 XRD curve of Fe3O4 particles. | |
3.2 Preparation of ms-PVA composite hydrogels
The synthetic route of the preparation of ms-PVA composite hydrogels illustrated in Scheme 1. First, PVA solution and an appropriate amount of Fe3O4 particles were mixed to form a homogeneous solution. Then, the ms-PVA composite hydrogels were fabricated by freezing and thawing the solution. The synthetic protocol was designed based on the following considerations. On one hand, the PVA molecular chain would crosslink each other to form a network structure by intermolecular or intramolecular hydrogen bonding through freezing and thawing; the structure consists of crystalline regions and amorphous region.35 On the other hand, the iron oxide particles could endow PVA with excellent magnetic characteristics and serve as a crosslinker to gelate PVA molecules.36 Moreover, the hydrogels exhibited excellent self-healing property through the reversible hydrogen bond between the hydroxyl groups because of the existence of a large number of hydroxyl groups in the PVA chain (Fig. 3). Fig. 4 shows the TEM images of ms-PVA hydrogels and ones after prolong freezing time. As shown in Fig. 4, PVA hydrogels all display like dried river beds, for neat PVA, a large number of cracks are observed, which provide relatively good swelling properties of the gel. However, with increasing the feeding amounts of Fe3O4 nanoparticles, few cracks are produced, this led to the poor water swelling properties of the hydrogel. In addition, the longer freezing time, the more cracks are formed.
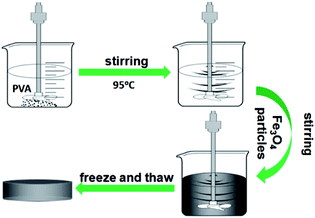 |
| Scheme 1 Synthetic route to the ms-PVA composite hydrogels. | |
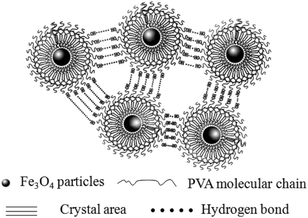 |
| Fig. 3 Internal structure of ms-PVA hydrogels. | |
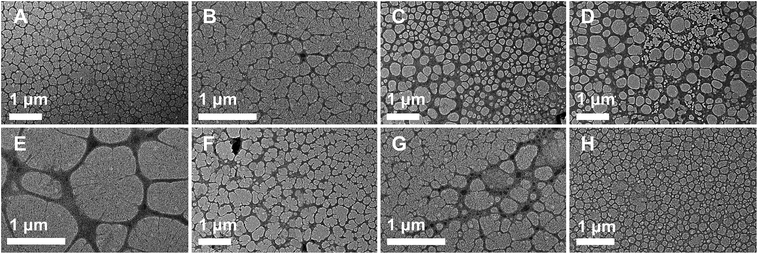 |
| Fig. 4 TEM images of ms-PVA hydrogels and ones after prolong freezing time ((A) neat PVA, (B) ms-PVA-1, (C) ms-PVA-2, (D) ms-PVA-3, (E) ms-PVA-4, (F) ms-PVA-2 freezing time 2 h, (G) ms-PVA-2 freezing time 3 h, (H) ms-PVA-2 freezing time 4 h). | |
3.3 Thermogravimetric analysis
Thermogravimetric analysis was conducted to investigate the stability of the ms-PVA composite hydrogels further as shown in Fig. 5. Neat PVA hydrogel was almost completely decomposed as the temperature approached 500 °C, and its residue weight was 5.4%. However, the composite gels had lower initial decomposition temperatures and higher final decomposition temperatures and residues compared to neat PVA due to the presence of Fe3O4 particles, which resulting in the decrease of the crystallinity of the soft crosslinked gel. The relative differences in the residual weight of composite gels above 500 °C were attributed to the inherently different compositions of Fe3O4 particles.
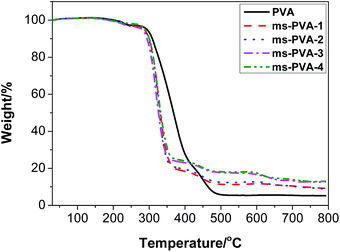 |
| Fig. 5 TGA curve of the hydrogels. | |
3.4 Magnetic properties
The magnetic properties of Fe3O4 particles (Fig. 6a) and the ms-PVA hydrogels (Fig. 6b) were measured by VSM at 300 K. The magnetization curves showed that Fe3O4 particles and ms-PVA hydrogels were superparamagnetic with no coercivity at room temperature. The actual saturation magnetization values for Fe3O4 particles, ms-PVA-1, ms-PVA-2, ms-PVA-3 and ms-PVA-4 were 64.7, 1.3, 2.7, 4.3 and 5.5 emu g−1, respectively, which were close to the theoretical value (see Table 2). Therefore, the magnetic intensity of the ms-PVA hydrogels can be readily tuned by altering the weight ratio of MIONs. The favorable property of ms-PVA hydrogel promises the remote control property as shown in the inset of Fig. 6b, the circle is the magnet as the external magnetic field, the black is the hydrogel sample, the hydrogel can interact with the magnet under the magnetic effect, so the remote control of the hydrogel can be realized by controlling the shift of the magnet.
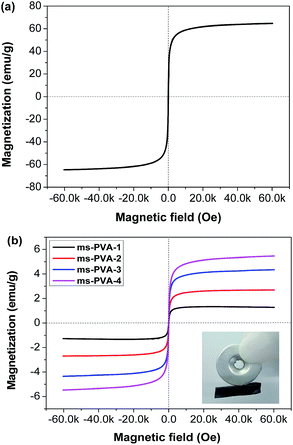 |
| Fig. 6 Magnetic hysteresis loops of Fe3O4 particles (a) and the ms-PVA hydrogels (b) at 300 K. | |
Table 2 Saturation magnetization for Fe3O4 particles and the ms-PVA hydrogels
|
Fe3O4 |
ms-PVA-1 |
ms-PVA-2 |
ms-PVA-3 |
ms-PVA-4 |
Saturation magnetization (emu g−1) |
64.7 |
1.3 |
2.7 |
4.3 |
5.5 |
Fe3O4 content (%, theoretical) |
100 |
2.4 |
4.8 |
7.0 |
9.1 |
Fe3O4 content (%, actual) |
100 |
2.0 |
4.2 |
6.6 |
8.5 |
3.5 Swelling capacity
The effect of Fe3O4 content on the swelling absorption of ms-PVA hydrogels was investigated. Fig. 7 shows that Fe3O4 content is an important factor that influences the water absorbency of the composite hydrogels. The increasing Fe3O4 weight ratio from 0.0% to 0.1% caused a decrease in water absorbency because of two factors. First, the interaction of the PVA chains with the Fe3O4 particles may form some low-mobility regions that can act as additional physical crosslinking points, which reduced the swelling ability of the matrix.36 Second, the addition of Fe3O4 particles reduces the content of PVA in hydrogel, which is the main factor influencing the water-absorbing capacity. Therefore, water absorbency decreased with the increase of in Fe3O4 weight ratio.
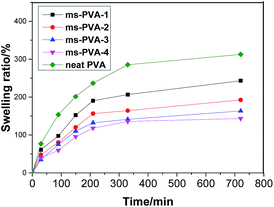 |
| Fig. 7 Swelling ratio of hydrogels. | |
3.6 Rheological analysis
3.6.1 Fe3O4 particle content. The rheological properties of ms-PVA hydrogels were evaluated by dynamic mechanical characterization. A series of hydrogels with different ratios of Fe3O4 particles was prepared, and the storage modulus G′ values versus frequency were tested (Fig. 8).
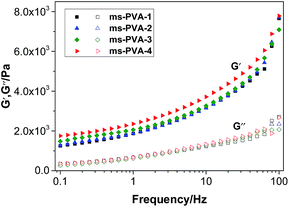 |
| Fig. 8 Frequency scanning of magnetic hydrogels. | |
Fig. 8 shows that the value of storage modulus G′ is higher than that of loss modulus G′′, indicating hydrogel formation.37 Moreover, with the increase in frequency (0.1 Hz to 100 Hz, strain (%) = 5) particles, the storage modulus G′ of the hydrogels increased. The polymer chains could not recover from previous movement in high frequency, indicating the formation of the stable structure of the hydrogels, which was not broken down by the mechanical shear force. Furthermore, a higher G′ value was observed for the samples with a high ratio of Fe3O4 particles, indicating that the addition of Fe3O4 particles had a positive effect on the gelation of PVA aqueous solution.
3.6.2 Freezing time. A series of ms-PVA-2 hydrogels with different freezing times was prepared, and the storage modulus G′ values versus frequency were tested (Fig. 9). The elastic modulus of the three hydrogels, shows that the storage modulus G′ gradually increased with the increase in freezing time, indicating that the internal hydrogel increasingly crosslinked and the hydrogel strength gradually increased, which reduced the PVA molecular mobility.
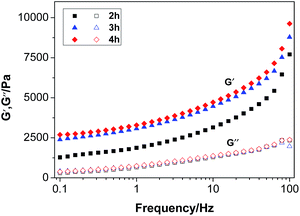 |
| Fig. 9 Frequency scanning of magnetic hydrogels. | |
3.6.3 Number of freezing and thaw cycles. A series of ms-PVA-2 hydrogels with different numbers of freezing and thaw cycles was prepared, and the storage modulus G′ values versus frequency were tested (Fig. 10). A higher G′ value was observed for the samples with more freezing and thaw cycles. This finding could be attributed to the presence of high crystallinity and the degree of crosslinking, which contribute to the strength of the network as the number of freezing and thawing cycles increased. However, the free hydroxyl of the PVA molecule will decrease as the degree of crosslinking increases, which may be unfavourable for the self-healing capacity of the hydrogels.
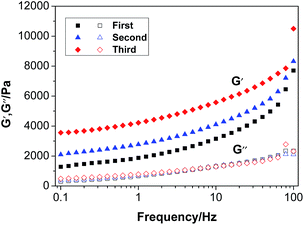 |
| Fig. 10 Frequency scanning of magnetic hydrogels. | |
3.6.4 Self-healing hydrogel. Rheological analyses were conducted to measure the self-healing process of the ms-PVA-2 hydrogel. After cutting the hydrogel into 8 pieces, the G′ and G′′ values of self-healing hydrogel versus frequency were almost the same as those of the original hydrogel (Fig. 11a), which qualitatively indicate the recovery of the inner structure of the hydrogel. Fig. 11b shows the hydrogel under a pulse deformation program. The strain initially increases from 5% to 200% at a certain time point and then returns to 5% after a certain period. The G′ and G′′ values are inverted under 1000% strain, which indicates the collapse of the gel network. G′ and G′′ return to their original values when placed under 5% strain. This recovery behavior could be repeatable for at least four cycles of varying strains, which indicates the rapid recovery of the inner network.38 All results support the self-healing ability of the dynamic hydrogels.
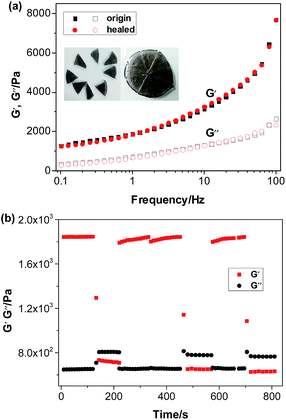 |
| Fig. 11 Frequency (a), strain (b) scanning of hydrogels. | |
3.7 Self-healing performance
Fe3O4 particles in the hydrogel do not affect the dynamic linkage because the hydrogel network is constructed through a reversible hydrogen bond. Thus, the magnetic hydrogel is self-healable, which is proven by the phenomenon shown in Fig. 12. A piece of the original hydrogels (1) was divided into two pieces using scissors (2) and placed together immediately to insure contact of the freshly created fracture surfaces (3). After 12 h, the self-healing hydrogel was stretched to break with a universal testing machine (4). The hydrogel do not even fracture at the wound (5). Thus, the ms-PVA hydrogels have excellent self-healing performance.
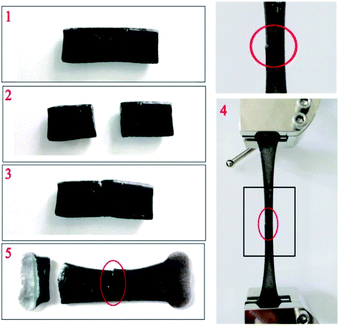 |
| Fig. 12 Self-healing process of magnetic hydrogel ((1) original sample; (2) cutting off; (3) healing; (4) stretching; (5) fracture). | |
3.8 Mechanical properties
3.8.1 Effect of freezing time. Previous studies have shown that the freezing time has an important influence on the self-healing properties of PVA hydrogel. Investigating the effect of freezing time (i.e., 2, 3 and 4 h) on the self-healing properties of ms-PVA-2 hydrogel is important. Fig. 13 shows that, with the increase in freezing time, the tensile strength of the hydrogel significantly increased from 0.2 MPa to 0.78 MPa, whereas the self-healing efficiency decreased from 100% to 9%. The longer the freezing time is, the higher the crosslinking degree of the hydrogel becomes, which leads to the improvement of the tensile strength of the hydrogel.39 However, less free hydroxyl groups in hydrogel were used for self-healing, which caused the decrease in self-healing efficiency.
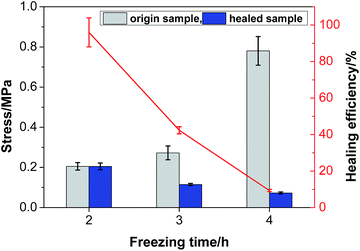 |
| Fig. 13 Effect of freeze time on self-healing properties of ms-PVA-2 hydrogel. | |
3.8.2 Effect of Fe3O4 particle content. A series of hydrogels with different Fe3O4 particle contents was prepared and the effect of Fe3O4 contents on the tensile strength and self-healing efficiency of the hydrogel was investigated. Fig. 14 illustrates that the tensile strength of the original samples was enhanced from 0.13 MPa to 0.32 MPa as the content of Fe3O4 particles increased from 2.5% to 10%. This observation indicated that the Fe3O4 particles in the PVA solution exhibited a nucleation effect to promote gel gelation, which results in the improvement of the initial strength of the hydrogel. When the content of Fe3O4 particles increased from 2.5% to 5%, the tensile strength after self-healing reached its maximum, and continuously increasing content of Fe3O4 particles diminished the self-healing efficiency. The Fe3O4 particles exerted an inhibiting effect on the binding of free hydroxyl groups through hydrogen bond when the content of Fe3O4 particles was more than 5%. Therefore, the ms-PVA-2 hydrogel exhibited the best self-healing strength and self-healing efficiency when the content of Fe3O4 particles was 5%.
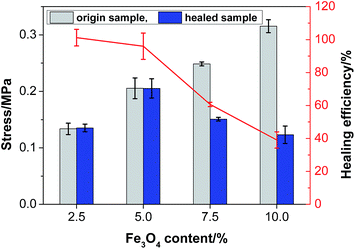 |
| Fig. 14 Effect of Fe3O4 content of self-healing properties of ms-PVA hydrogels. | |
3.8.3 Effect of the number of freezing and thawing. The effects of the number of freezing and thawing cycles on the self-healing properties of the ms-PVA-2 hydrogel were investigated. Fig. 15 shows that the self-healing efficiency of the hydrogels decreases drastically as the number of freezing and thawing cycles increases. The PVA hydrogel prepared using the freezing and thawing method became harder and more opaque with the increase in the number of freezing thawing cycles as a result of the increased crystallinity of PVA chains.40 Generally, greater hardness of the hydrogel means lesser chain mobility, which indicates that the molecular chains on the two surfaces cannot migrate or diffuse from one side to the other. Thus the self-healing process cannot develop effectively. This finding explains the decrease in the fracture stress of self-healing hydrogel with the increase in the number of freezing and thawing cycles.
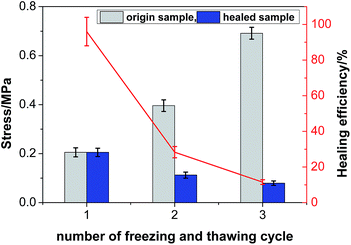 |
| Fig. 15 Effect of freezing and thawing times on self-healing properties of ms-PVA-2 hydrogel. | |
3.8.4 Healing time. The effect of healing time on the self-healing efficiency was evaluated because healing time is a crucial parameter for practical application. Fig. 16 shows that the self-healing efficiency increased rapidly in the initial stage within the first 60 min slowly increased and reached 100% after 12 h. The rapid step of self-healing efficiency in the beginning was due to the existence of a large number of free OH− on the fracture surface and excellent chain mobility.33 These results indicated the occurrence of a rapid and efficient autonomous self-healing process in such physically crosslinked ms-PVA hydrogel.
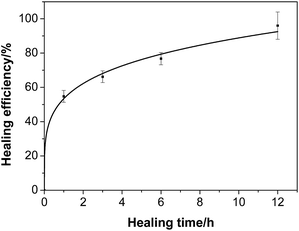 |
| Fig. 16 Effect of healing time on self-healing properties of ms-PVA-2 hydrogel. | |
3.8.5 Repeat self-healing properties. For self-healing materials, the repeat self-healing performance is important to the service life of the material. Fig. 17 shows the repeat self-healing performance of the ms-PVA-2 hydrogel. Repair times were set at 10, 30, and 60 min. The figure shows that the tensile strength after healing is high for the first healing in all three conditions. Healing efficiency reached 42%, 48% and 54%, respectively. The longer the repair time is, the higher the healing efficiency becomes. This finding can be attributed to increased uncrosslinked hydroxyl groups on the fractured hydrogel surface and improved molecular mobility. Afterward, the healing efficiency was reduced for later freezing and thawing cycles. The hydrogels lose their self-healing ability when they were repaired for the fourth time, they lose self-healing ability because of the decrease in the molecular mobility of the fracture surface. Therefore, PVA-124 hydrogels exhibited relatively good repeated self-healing performance.
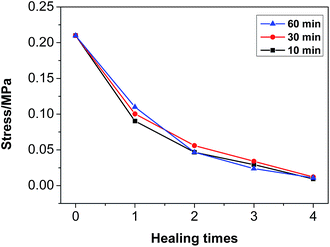 |
| Fig. 17 Repeat self-healing properties of ms-PVA-2 hydrogel. | |
4. Conclusions
In summary, this study proposed an easy and viable strategy to fabricate superparamagnetic self-healing bifunctional PVA composite hydrogels by simply adding magnetic iron oxide particles to physically crosslinked PVA hydrogel by freezing and thawing. The obtained ms-PVA hydrogels show a controllable magnetic property by adjusting the magnetic iron oxide content. Moreover, the addition of magnetic iron oxide particles largely affects the self-healing property of the ms-PVA hydrogel because of its influence on the crosslinked structure of the composite hydrogel. The results highlight the combined advantages of self-healing and magnetic hydrogels, which extend the application of the PVA hydrogel.
Acknowledgements
The authors greatly acknowledgement the financial support from the Natural Science Foundation of China (21364003, 51263004).
References
- J. Jagur-Grodzinski, Polym. Adv. Technol., 2010, 21, 27–47 CAS.
- M. Ahmad, S. M. Rai and A. Mahmood, Adv. Polym. Technol., 2016, 35, 121–128 CrossRef CAS.
- H.-J. Gwon, Y.-M. Lim, S.-J. An, M.-H. Youn, S.-H. Han, H.-N. Chang and Y.-C. Nho, Korean J. Chem. Eng., 2009, 26, 1686–1688 CrossRef CAS.
- B. S. Fazly Bazzaz, B. Khameneh, M.-m. Jalili-Behabadi, B. Malaekeh-Nikouei and S. A. Mohajeri, Cont. Lens Anterior Eye, 2014, 37, 149–152 CrossRef PubMed.
- M. Montiel-Herrera, A. Gandini, F. M. Goycoolea, N. E. Jacobsen, J. Lizardi-Mendoza, M. Recillas-Mota and W. M. Argüelles-Monal, Carbohydr. Polym., 2015, 128, 220–227 CrossRef CAS PubMed.
- M. Liu, Y. Ishida, Y. Ebina, T. Sasaki, T. Hikima, M. Takata and T. Aida, Nature, 2015, 517, 68–72 CrossRef CAS PubMed.
- F. Song, X. Li, Q. Wang, L. Liao and C. Zhang, J. Biomed. Nanotechnol., 2015, 11, 40–52 CrossRef CAS PubMed.
- Y. J. Che, D. P. Li, Y. L. Liu, Q. L. Ma, Y. B. Tan, Q. Y. Yue and F. J. Meng, RSC Adv., 2016, 6, 106035–106045 RSC.
- M. Criado, J. M. Rey, C. Mijangos and R. Hernandez, RSC Adv., 2016, 6, 105821–105826 RSC.
- C. J. Hansen, S. R. White, N. R. Sottos and J. A. Lewis, Adv. Funct. Mater., 2011, 21, 4320 CrossRef CAS.
- S. Basak, J. Nanda and A. Banerjee, Chem. Commun., 2014, 50, 2356–2359 RSC.
- K. Basu, A. Baral, S. Basak, A. Dehsorkhi, J. Nanda, D. Bhunia, S. Ghosh, V. Castelletto, I. W. Hamley and A. Banerjee, Chem. Commun., 2016, 52, 5045–5048 RSC.
- L. Zhou, B. He and F. Zhang, ACS Appl. Mater. Interfaces, 2011, 4, 192–199 Search PubMed.
- G. Gong, F. Zhang, Z. Cheng and L. Zhou, Int. J. Biol. Macromol., 2015, 81, 205–211 CrossRef CAS PubMed.
- A. Fernández-Ferreiro, M. González Barcia, M. Gil-Martínez, A. Vieites-Prado, I. Lema, B. Argibay, J. Blanco Méndez, M. J. Lamas and F. J. Otero-Espinar, Eur. J. Pharm. Biopharm., 2015, 94, 342–351 CrossRef PubMed.
- M. Häring, J. Schiller, J. Mayr, S. Grijalvo, R. Eritja and D. D. Díaz, Gels, 2015, 1, 135–161 CrossRef.
- G. R. Mahdavinia, H. Etemadi and F. Soleymani, Carbohydr. Polym., 2015, 128, 112–121 CrossRef CAS PubMed.
- N. Zhang, J. Lock, A. Sallee and H. Liu, ACS Appl. Mater. Interfaces, 2015, 7, 20987–20998 CAS.
- F.-Y. Chou, C.-M. Shih, M.-C. Tsai, W.-Y. Chiu and S. J. Lue, Polymer, 2012, 53, 2839–2846 CrossRef CAS.
- Y. Zhang, B. Yang, X. Zhang, L. Xu, L. Tao, S. Li and Y. Wei, Chem.
Commun., 2012, 48, 9305–9307 RSC.
- S. R. White, N. Sottos, P. Geubelle, J. Moore, M. R. Kessler, S. Sriram, E. Brown and S. Viswanathan, Nature, 2001, 409, 794–797 CrossRef CAS PubMed.
- D. L. Taylor and M. In Het Panhuis, Adv. Mater., 2016, 28, 9060–9093 CrossRef CAS PubMed.
- Y. Zhang, B. Yang, L. Xu, X. Zhang, L. Tao and Y. Wei, Acta Phys.-Chim. Sin., 2013, 71, 485–492 CrossRef CAS.
- Z. Wei, J. H. Yang, X. J. Du, F. Xu, M. Zrinyi, Y. Osada, F. Li and Y. M. Chen, Macromol. Rapid Commun., 2013, 34, 1464–1470 CrossRef CAS PubMed.
- G. Deng, F. Li, H. Yu, F. Liu, C. Liu, W. Sun, H. Jiang and Y. Chen, ACS Macro Lett., 2012, 1, 275–279 CrossRef CAS.
- Y. Xu, Q. Wu, Y. Sun, H. Bai and G. Shi, ACS Nano, 2010, 4, 7358–7362 CrossRef CAS PubMed.
- A. Phadke, C. Zhang, B. Arman, C.-C. Hsu, R. A. Mashelkar, A. K. Lele, M. J. Tauber, G. Arya and S. Varghese, Proc. Natl. Acad. Sci. U. S. A., 2012, 109, 4383–4388 CrossRef CAS PubMed.
- M. M. C. Bastings, S. Koudstaal, R. E. Kieltyka, Y. Nakano, A. C. H. Pape, D. A. M. Feyen, F. J. van Slochteren, P. A. Doevendans, J. P. G. Sluijter, E. W. Meijer, S. A. J. Chamuleau and P. Y. W. Dankers, Adv. Healthcare Mater., 2014, 3, 70–78 CrossRef CAS PubMed.
- X. Wang, F. Liu, X. Zheng and J. Sun, Angew. Chem., Int. Ed., 2011, 50, 11378–11381 CrossRef CAS PubMed.
- Q. Wang, J. L. Mynar, M. Yoshida, E. Lee, M. Lee, K. Okuro, K. Kinbara and T. Aida, Nature, 2010, 463, 339–343 CrossRef CAS PubMed.
- U. Gulyuz and O. Okay, Soft Matter, 2013, 9, 10287–10293 RSC.
- G. Jiang, C. Liu, X. Liu, G. Zhang, M. Yang, Q. Chen and F. Liu, J. Macromol. Sci., Part A: Pure Appl. Chem., 2010, 47, 335–342 CrossRef CAS.
- H. Zhang, H. Xia and Y. Zhao, ACS Macro Lett., 2012, 1, 1233–1236 CrossRef CAS.
- Y. Zhou, T. Fan, R. Yang, H. Sun and Q. Xia, J. Pharm. Sci., 2007, 16, 187 Search PubMed.
- H. Hong, H. Liao, S. Chen and H. Zhang, Mater. Lett., 2014, 122, 227–229 CrossRef CAS.
- J. S. Gonzalez, C. E. Hoppe, D. Muraca, F. H. Sanchez and V. A. Alvarez, Colloid Polym. Sci., 2011, 289, 1839–1846 CAS.
- S. Li, H.-Y. Lu, Y. Shen and C.-F. Chen, Macromol. Chem. Phys., 2013, 214, 1596–1601 CrossRef CAS.
- P. Sahoo, R. Sankolli, H.-Y. Lee, S. R. Raghavan and P. Dastidar, Chem.–Eur. J., 2012, 18, 8057–8063 CrossRef CAS PubMed.
- N. A. Peppas and S. R. Stauffer, J. Controlled Release, 1991, 16, 305–310 CrossRef CAS.
- M. Lee, H. Bae, S. Lee, N.-o. Chung, H. Lee, S. Choi, S. Hwang and J. Lee, Macromol. Res., 2011, 19, 130–136 CrossRef CAS.
|
This journal is © The Royal Society of Chemistry 2017 |