DOI:
10.1039/C6RA27414E
(Paper)
RSC Adv., 2017,
7, 14649-14659
A glassy carbon electrode modified with γ-Ce2S3-decorated CNT nanocomposites for uric acid sensor development: a real sample analysis†
Received
27th November 2016
, Accepted 21st February 2017
First published on 6th March 2017
Abstract
γ-Ce2S3-decorated multi-walled carbon nanotube nanocomposite (Ce2S3-CNT NC) was synthesized by a wet chemical method in basic phase. Characterization of the resulting Ce2S3-CNT NCs was performed in detail by field emission scanning electron microscopy (FE-SEM) attached with energy dispersive spectroscopy (EDS), X-ray photoelectron spectroscopy (XPS), UV/vis spectroscopy, FT-IR spectroscopy, and X-ray diffraction (XRD). A glassy carbon electrode (GCE) was fabricated using the Ce2S3-CNT NCs and then it was utilized to develop a biological sensor for the detection of uric acid (UA) via a simple electrochemical approach. With the selectivity study, UA was selected as a target since it exhibited a fast response towards the Ce2S3-CNT NC-fabricated GCE sensor in the I–V method. The fabricated sensor also displayed an excellent sensitivity, very low detection limit, long-term stability, and reproducibility. In the diagnostic study, a linear calibration plot (r2 = 0.9972) was obtained for 0.1 nM to 1.0 mM aqueous UA solution, with the sensitivity value of 1.5822 μA nM−1 m−2 and an extremely low detection limit LOD = 60.0 ± 0.05 pM (S/N = 3). Potentials of the Ce2S3-CNT NCs in terms of biological sensing were also investigated via real serum and urine sample analyses. This approach is emerging as an effective technique in the development of an efficient biological sensor for the detection of biochemicals in broad scales.
1. Introduction
Uric acid (UA) is an important biomolecule that is often present in blood serum, urine, or biological fluids and is excreted from the human body.1,2 UA is a product of the metabolite of purine nucleotides, nucleic acids, and nucleoproteins.3 Due to its poor solubility in water (approximately 60 mg L−1), UA can easily accumulate in human body. Moreover, UA when present in excess in the body fluid can also solidify as a urate, which may cause gout or kidney stones.4 High uric acid concentration in the blood may lead to deterioration of the renal function and also causes several diseases such as gout, myocardial infarction, hyperuricemia, physiological disorders, and Lesch–Nyhan syndrome.5–7 Therefore, monitoring the uric acid level in the body fluid is sometimes considered as a key factor for the evaluation of a health condition.8 Therefore, detection of UA is essential for diagnosis as well as public health monitoring. Various enzyme-based sensors have already been reported for UA detection. However, enzymes can properly work only under appropriate conditions and hence these sensors cannot be used for a wide range of applications. On the other hand, non-enzymatic biosensors are more versatile.2 Electrochemical sensors always offer fast, profound, and cost-effective methods in UA detection and quantification.9,10 However, the electrochemical response of UA at a bare electrode is kinetically slow and often associated with high overpotential. Consequently, searching for new materials for the modification of electrodes to enhance the rate of electron transfer and reduce the overpotential is required.11 Carbonaceous materials such as CNT, graphene oxides, carbon black, fullerenes, etc. exhibit promising electrocatalytic properties.12–15 Liu et al. proposed a CNT composite-based sensor for electrically conductive strain sensing, conductive thermoplastic polyurethane–graphene nanocomposite-based organic vapor sensing, and a strain sensor based on conductive thermoplastic elastomer-graphene nanocomposites.16–18
Several redox mediators such as pristine graphene,1 gold nanocluster,3 Au–Pt bimetallic nanoclusters decorated on graphene oxide,19 mesoporous nickel oxide,3 polypyrrole films doped with ferrocyanide ions onto an iron substrate,20 reduced graphene oxide/zinc sulfide nanocomposite,21 calixarene-modified glassy carbon electrode,22 flexible graphene fibers,23 poly(glyoxal-bis(2-hydroxyanil))-fabricated GCE,24 CeO2 nanoparticle-fabricated glassy carbon paste electrode,25 iron nanoparticle-fabricated MWCNT enriched carbon paste electrode,26 carbon nanohorns/poly(glycine)-modified glassy carbon electrode27 etc. have been developed to date for the modification of the electrode in UA detection; however, all these attempts are either time consuming, require sophisticated instruments or are not cost-effective for routine analysis. Moreover, due to low-cost, rapid response, and higher sensitivity, electrochemical sensors are often considered more useful than any other methods for UA detection. For the real IT application of sensors, the challenge is due to advances in device fabrication, mechatronics, and communication technologies and limited availability of sophisticated sensor and actuator devices. It targets both everyday life industrial and mission-critical needs, which are either confined to a single target device or distributed within a network of units, by also taking advantage of seamless communication capabilities and address different application scenarios.28 These represent mostly relate to ecology or environment in which they are placed through active sensors and actuators, hence setting the IT technological bases for the cyber-physical framework. In some cases, sensors and actuators can be cybernetic or virtual when physical entity is not there but data-streams are generated and decisions are appropriately taken based on the information provided. For an environmentally intelligent sensor, table-top object manipulation is one of the most collective tasks, which combines the capabilities of robotic vision, image processing, object recognition, hand-arm manipulation, etc. However, the real indoor environment is much more convoluted than experimental scenarios. Sometimes the vision of a robot can hardly offer enough information for successfully executing some challenging tasks such as picking, placing or assembling some small targeting objects. In these circumstances, if two objects are too close to each other, it is difficult to correctly segment them; moreover, some occlusion cases often occur in the real indoor environment. Thus, a teleoperative demonstration method is an efficient way to overcome these problems. These demonstration techniques have already been implemented on industrial robots for several years.29
Recently, nanostructured transition metal sulfides have become more important in the research field due to their excellent electrochemical properties.30 Cerium sulfides show important roles as electrocatalysts among all other transition metal sulfides. To date, core–shell structured Ce2S3 on ZnO, Ce2S3 on SiO2, undoped and sodium-doped γ-Ce2S3 amorphous cerium sulphides, cerium sulfide/graphene nanocomposites, etc. have been prepared;31,32 however, these efforts have become unsuccessful in improving the electrochemical properties of cerium sulfides because of ineffective external interaction between cerium sulfides and carbonaceous substances. This ineffective communication among redox active entities and charge-transfer materials will only allow the fractional cerium sulfide molecules to join in the electron transfer process. Hence, unexpected electrochemical properties of cerium sulfides achieved slower charge-transfer than that of carbonaceous materials. To overcome these complications, herein, we proposed a facile wet-chemical method to prepare Ce2S3-CNT NCs in the form of interconnected three dimensional conductive links where Ce2S3 nanostructures are attached in and around CNT. Dispersed CNT solution plays a role as a precursor in these attachments, where γ-Ce2S3 nanoparticles are deposited in and around the CNT. An important benefit of these nanocomposites is that nanostructured cerium sulphides are firmly attached to the CNT and these two different entities are rigidly joined together. The as-grown nanocomposite Ce2S3-CNT NC exhibits unprecedented electrochemical performance. Since the improvement of the electrochemical properties of CNT, by making them nanocomposites, is cost-effective and quick relative to that of others reported in the literature,24 herein, we proposed an electrochemical sensor for the detection and quantification of UA in aqueous solutions (even at pico molar level) using Ce2S3-CNT NC-fabricated GCE. To the best of our knowledge, a Ce2S3-CNT NC-fabricated GCE sensor has not been reported to date, and herein, this sensor displays the lowest detection limit (LOD = 60.0 ± 0.05 pM) for the detection of UA than any other reported sensor.
2. Experimental
2.1. Materials and methods
Cerium(III)chloride heptahydrate (CeCl3·7H2O), hydrated sodium sulphide (Na2S·9H2O), ethanol, disodium phosphate, monosodium phosphate, multi-walled CNT, creatinine, cystine, glycine, leucine, thionin acetate, tyrosine, uric acid, Nafion (5% ethanolic solution), and acetone were used without any further purification and were purchased from the Sigma-Aldrich Company. Ce2S3-CNT NCs was investigated via UV/vis spectroscopy (Evolution 300 UV/visible spectrophotometer, Thermo scientific). An FT-IR spectrum was obtained for the Ce2S3-CNT NCs using a spectrophotometer (NICOLET iS50 FTIR spectrometer, Thermo scientific.) in the range from 400 to 4000 cm−1. The XPS measurements were carried out to estimate the binding energies in eV for C, Ce, and S using a MgKα1 spectrometer (Thermo scientific, Kα 1066, USA) with an excitation radiation source (Al Kα, beam spot size = 300.0 μm, pass energy = 200.0 eV, and pressure ∼ 10−8 torr). The powdered XRD spectrum was obtained by an X-ray diffractometer (XRD, Thermo scientific, ARL X'TRA diffractometer) with Cu Kα1 radiation (λ = 1.5406 nm) using the generator voltage of 40 kV and current of 35 mA applied for the measurement. Morphologies of the Ce2S3-CNT NCs were investigated by FESEM (JEOL, JSM-7600F, Japan). Elemental analysis was carried out by EDS (JEOL, Japan). I–V measurements were performed by the Keithley, 6517A Electrometer, USA, at 25.0 °C.
2.2. Synthesis of the Ce2S3-CNT NCs
A simple wet-chemical method was used to synthesize Ce2S3-CNT NCs in an alkaline medium using cerium(III)chloride heptahydrate (CoCl3·7H2O), sodium sulphide (Na2S·9H2O), and CNT. In the synthesis process, 50.0 mL of CeCl3 solution (0.1 M) and 50.0 mL of Na2S solution (0.1 M) were separately prepared in deionized (DI) water. Then, 1.0 mg of CNT was added to 50.0 mL of cerium(III)chloride solution in a conical flask and heated up to 85 °C for 30 minutes under continuous stirring. Later, 50.0 mL of the Na2S solution was added dropwise to the abovementioned mixture under constant stirring. After 6 hours of continuous stirring at 85.0 °C, the reaction mixture was cooled down to room temperature and yielded black precipitate of the Ce2S3-CNT NCs. The black precipitate was washed by DI water and alcohol to remove any organic or inorganic impurities present and dried at room temperature. Then, the collected black powdered sample was dried at 65.0 °C using an oven for 6 h and finally the as-grown Ce2S3-CNT NCs were obtained.
The reaction scheme of Ce2S3-CNT NCs formation first depends on the slow release of Ce3+ and S2− ions within the solution and second on the precipitation of these ions as Ce2S3 in and around the CNT. Formation of Ce2S3 depends on the fact that the ionic product of Ce3+ and S2− ions is greater than the solubility product of Ce2S3. Ce3+ and S2− ions are provided by the hydrolysis reactions of CeCl3 and Na2S, respectively. The proposed mechanism may be described as follows:
CeCl3 gets ionized in water (eqn (i)) and dispersed in and around the CNT. Na2S also gets ionized (eqn (ii)) and S2− ions will also diffuse into the solution.
|
CeCl3 → Ce3+(aq) + 3Cl−(aq)
| (i) |
|
Na2S → 2Na+(aq) + S2−(aq)
| (ii) |
Effective collisions between Ce3+ and S2− ions cause nucleation followed by aggregation and finally the formation of Ce2S3-CNT NCs in the presence of dispersed CNTs (eqn (iii)).
|
CNT(disp) + 2Ce3+(aq) + 3S2−(aq) → Ce2S3-CNT(s)↓
| (iii) |
The overall reaction can be written as follows (eqn (iv)):
|
2CeCl3(aq) + 3Na2S(aq) + CNT(disp) → Ce2S3-CNT(s)↓ + 6Na+(aq) + 6Cl−(aq)
| (iv) |
Finally, the as-grown Ce2S3-CNT NCs were washed by DI water and alcohol to remove any impurities present and dried at 65.0 °C using an oven. As-obtained Ce2S3-CNT NCs were characterized in detail for crystallinity, morphology, structural, electrochemical properties etc. and later used as a fabricating material on the GCE for the UA sensor in the simple I–V technique. In the Ce2S3-CNT NCs growth mechanism, at the beginning, growth of the Ce2S3 nucleus occurs by itself with mutual aggregation. As described by the Ostwald ripening method, later, nanocrystals re-aggregate to form combined γ-Ce2S3 nanocrystals.33–36 In the presence of dispersed CNTs, γ-Ce2S3 nanocrystals crystallize and re-aggregate with one another and are deposited in and around CNT using van der Waals forces, which result in the porous morphology of the Ce2S3-CNT NCs, which is presented in Scheme 1.
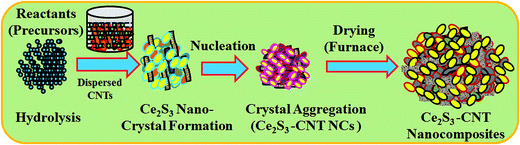 |
| Scheme 1 Probable mechanism of Ce2S3-CNT nanocomposites synthesis via the wet chemical process. | |
2.3. Fabrication of GCE using Ce2S3-CNT NCs and detection of UA
Fabrication of GCE was carried out by the as-grown Ce2S3-CNT NCs using 5% ethanolic Nafion solution as the conducting binder. It was then heated in an oven at 65 °C for 2 h to obtain a dry film on GCE. In the electrochemical cell, a working electrode (WE) was made of the Ce2S3-CNT NC-coated GCE, whereas the counter electrode was made by a platinum (Pt) wire and aqueous UA in the buffer solution was the electrolyte. To use as target analytes, aqueous UA solution (0.1 M) was diluted to different concentrations (from 1.0 mM to 0.01 nM) using deionized water. All I–V measurements were carried out in 5.0 mL of phosphate buffer solution (PBS; 0.1 M; pH 7.0). From the slope of the current vs. voltage plot, the sensitivity of the proposed UA sensor was estimated.37 Using an electrometer, I–V method was applied to UA solutions with Ce2S3-CNT NCs/GCE as the WE. Real samples were obtained from the medical center (KAU medical center). Initially, the rabbit serum was isolated from the blood. After dilution in PBS buffer, real serum and urine samples were analyzed via the fabricated Ce2S3-CNT NCs/GCE sensor using the I–V method under ambient conditions.
3. Results and discussion
3.1. Optical and structural evaluation
We analyzed the UV/vis spectrum (300–600 nm) of the as-grown Ce2S3-CNT NCs to obtain the band-gap energy (Ebg) of Ce2S3-CNT NCs. The broad peak at 493 nm was attributed to the characteristic peak of the Ce2S3, which is shown in Fig. 1(a). Using the UV/vis spectral data in the Tauc's equation, the Ebg for the Ce2S3-CNT NCs was calculated.38–40 Tauc's equation (eqn (v)) can be represented aswhere n = 1/2 or 2 for a direct or indirect electronic transition, respectively. From the (αhν)2 vs. (hν) plot (Fig. 1(b)), the Ebg for the as-grown Ce2S3-CNT NCs was estimated as ∼2.31 eV, which is consistent with those of the previously reported Ce2S3-CNT NCs.
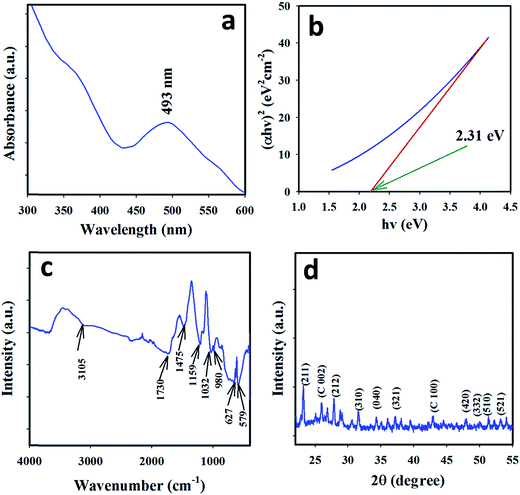 |
| Fig. 1 Optical and structural evaluation: (a) UV/vis spectrum, (b) Tauc's plot for the Ebg, (c) FTIR spectrum, and (d) XRD spectrum of the as-grown Ce2S3-CNT NCs. | |
The FTIR spectrum of the as-grown Ce2S3-CNT NCs is shown in Fig. 1(c). Broad peaks at 3105 and 1475 cm−1 are due to the stretching and bending mode of vibration of water, respectively.41 The stretching vibration of Ce2S3 gives a peak at 579 cm−1. Peaks at 1159 and 980 cm−1 can be attributed to C–O single bond vibrations. The peak at 1730 cm−1 might be due to C
O. The C
S and C–S vibrational peaks occur at 1032 cm−1 and 627 cm−1, respectively.42
The XRD pattern, as shown in Fig. 1(d), matched JCPDS # 50-0851 for the γ-Ce2S3 orthorhombic phase.23 Peaks at 25.8° and 42.7° indicate the presence of CNT.43–47 The more intense (211) peak at 23.2° implies that the (211) plane of the orthorhombic structure is slanted towards the experimental system. Overall, the whole XRD spectrum resembled that of the orthorhombic phase structure of γ-Ce2S3.
3.2. Morphological and elemental evaluation
The morphology and structure of the Ce2S3-CNT NCs were investigated by FESEM. Typical morphological information of the as-grown Ce2S3-CNT NCs is presented in Fig. 2(a)–(d). As-grown Ce2S3-CNT NCs exhibit interconnected networks of carbon nanotubes and Ce2S3 nanoparticles are adsorbed onto the CNT (white spots). This exceptional structure with self-assembled orthorhombic Ce2S3 nanoparticles provides a large surface area and increases the electron transport.
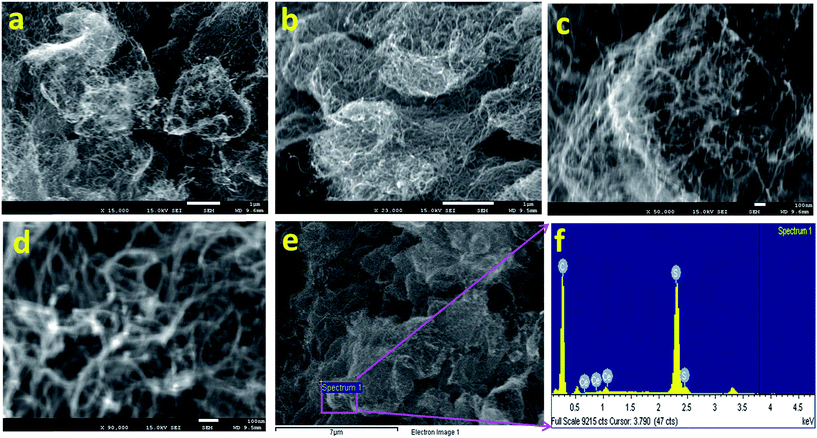 |
| Fig. 2 Morphological and elemental evaluation. (a–d) Low to high-resolution FE-SEM images and (e and f) EDS spectrum for the as-grown Ce2S3-CNT NCs. | |
The chemical compositions were determined by the EDS analysis (Fig. 2(e) and (f)) of the as-grown Ce2S3-CNT NCs, which confirmed the presence of C, S, and Ce. Composition (wt%) of carbon, sulphur, and cerium in the as-grown Ce2S3-CNT NCs was 84.20%, 15.57%, and 0.23%, respectively. The absence of any other extra peak(s) (Fig. 2(f)) confirms the purity of the Ce2S3-CNT NCs.
3.3. Evaluation of binding energy
XPS was used for further investigation of the purity and nanostructure of the Ce2S3-CNT NCs. From the full scan spectrum (Fig. 3(a)), it can be deduced that the surface of the Ce2S3-CNT NCs consisted of cerium, sulfur, carbon, and oxygen atoms. Oxygen atoms present in the Ce2S3-CNT NCs may attach to carbon atoms by a carbon–oxygen single or double covalent bond or as C
O.48 Obtained binding energy values of C1s and O1s of the as-grown Ce2S3-CNTNCs are 284.9 eV and 532.4 eV, respectively (Fig. 3(b) and (c)), which are very close to the reported values.49 The Ce3d spectra consist of three well-resolved peaks (Fig. 3(d)) located at 933.6, 923.4, and 918.7 eV, corresponding to Ce3d5/2, Ce3d3/2, and Ce3d1/2, respectively, which are also in good agreement with the binding energy of cerium in Ce2S3. As shown in Fig. 3(e), the two photoelectron signals at 164.2 and 169.4 eV can be attributed to S2p3/2 and S2p1/2, respectively, and the photoelectron signals at 228.4 eV, as shown in Fig. 3(f), can be attributed to S2s.50 Main peaks in the XPS spectra (Fig. 3(a)–(f)) are consistent with those of previously reported Ce2S3.
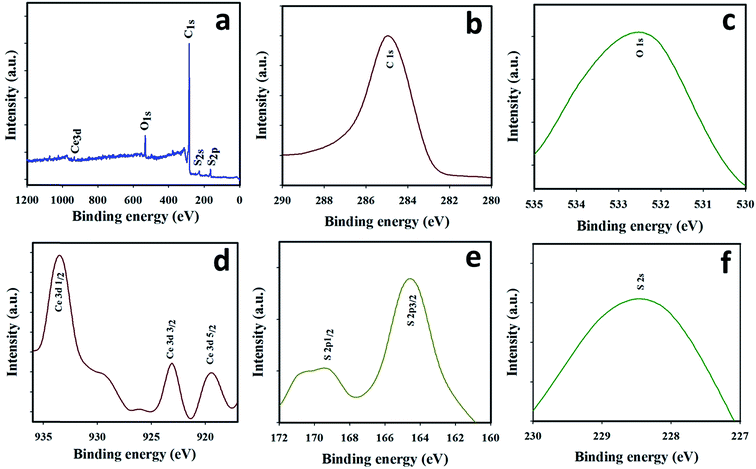 |
| Fig. 3 Binding energy evaluation: XPS spectrum of (a) as-prepared Ce2S3-CNT NCs, (b) C1s, (c) O1s, (d) Ce3d, (e) S2p, and (f) S2s orbital acquired using MgKα1 radiation. | |
4. Applications
4.1. Detection of UA using the Ce2S3-CNT NCs/GCE
UA in an aqueous solution was detected and the content was measured using the Ce2S3-CNT NC-fabricated GCE as the chemical sensor. Non-toxic nature, chemical stability, and electrochemical activity make the Ce2S3-CNT NCs one of the best sensing materials for UA. UA provided a remarkable response upon contact with the Ce2S3-CNT NCs in the I–V measurement. Fig. 4(a) shows the Ce2S3-CNT NCs/GCE electrode surface prepared in 5% ethanolic Nafion solution. The possible reduction scheme on the Ce2S3-CNT NCs/GCE is generalized in Fig. 4(b), where UA gets reduced by gaining electrons from the conduction band of the Ce2S3-CNT NCs/GCE sensor surface during the I–V measurements, which decreases the current intensity with the increasing concentration at room temperature.51–53 Fig. 4(c) shows the theoretical outcome, whereas Fig. 4(d) shows the practical I–V response with UA and without UA on the Ce2S3-CNT NCs/GCE working electrode at the delay time of 1.0 second in the electrometer, where a higher current response to the increasing voltage is clearly demonstrated.
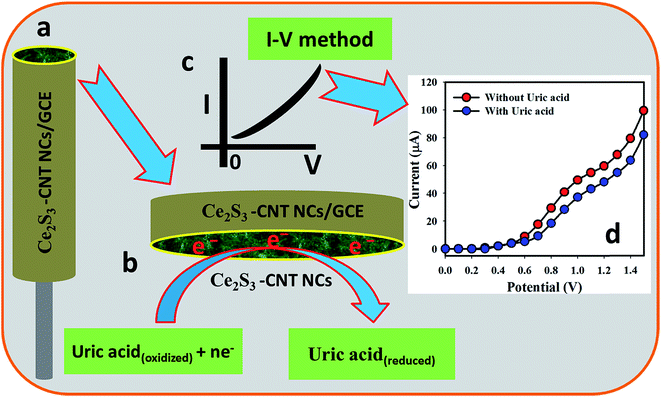 |
| Fig. 4 Schematic for the (a) Ce2S3-CNT NC-coated rod-shaped round-GCE electrode with conducting Nafion (5% ethanol) coating binders, (b) proposed detection mechanism of UA, where UA is reduced by removing conducting electrons from the Ce2S3-CNT NCs/GCE electrodes, (c) theoretical outcome of I–V measurement, and (d) observed I–V response by the Ce2S3-CNT NCs/GCE with the delay time of 1.0 s. | |
4.2. Optimization and application of the UA biosensor
The electrochemical behavior of the Ce2S3-CNT NCs depends on the pH value. The pH dependence of the Ce2S3-CNT NCs was examined in PBS with different pH values (5.7 to 8.0), as shown in Fig. 5(a). These results demonstrate that the Ce2S3-CNT NCs show good electrochemical performance at various pH values. It can be well observed that on changing the pH value of the PBS, the electrocatalytic activity of the Ce2S3 CNT NCs changed, which is reflected by a variety of current responses. During the pH optimization using PBS (without using UA), the highest current response was obtained at pH 7.0. Therefore, pH 7.0 was kept constant throughout this study during the UA detection using the Ce2S3-CNT NCs/GCE assembly.
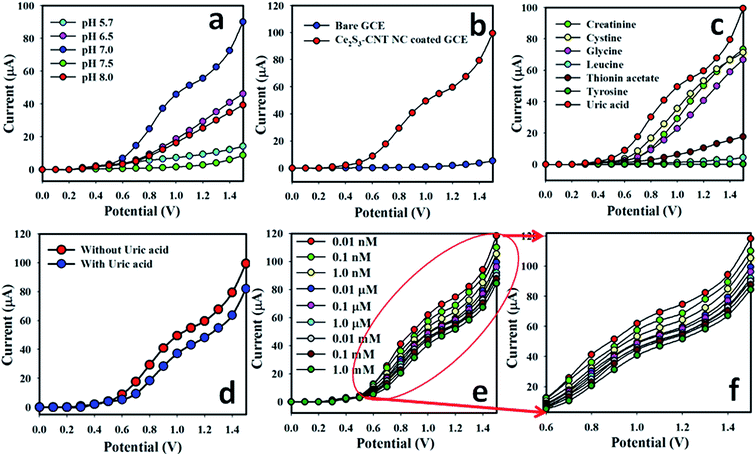 |
| Fig. 5 I–V responses with a delay time of 1.0 s for (a) pH optimization of the Ce2S3-CNT NC-coated GCE, (b) bare and Ce2S3-CNT NC-coated GCE in PBS with UA, (c) selectivity study with various interferences (7 analytes) (d) in the absence and presence of UA (1.0 μM; 25.0 μL) in 5.0 mL of PBS solution, (e) current variations for different concentrations (from 0.01 nM to 1.0 mM) of aqueous UA solution in the full voltage range, and (f) magnified view of concentration variation (+0.6 to +1.5 V). | |
Current intensities in PBS (pH = 7.0) without UA for the bare GCE (blue dotted) and Ce2S3-CNT NCs/GCE (red-dotted) are given in Fig. 5(b). With the Ce2S3-CNT NCs/GCE, the current intensity is much higher as compared to that with the bare GCE for UA detection, which demonstrates the excellent electrochemical properties of the Ce2S3-CNT NCs. Fig. 5(c) demonstrates the current responses to seven biological analytes, where (1.0 μM; 25.0 μL) UA solution (red-dotted) in PBS (pH = 7.0) gave the best response with the Ce2S3-CNT NCs/GCE surface. Fig. 5(d) represents I–V responses of the Ce2S3-CNT NCs/GCE without UA (blue-dotted) and with UA (red-dotted; 1.0 μM; 25.0 μL) in 5.0 mL of PBS solution. In the presence of UA in PBS, a noticeable decrease in the current response implies the UA sensing ability of the developed Ce2S3-CNT NCs/GCE sensor. UA solution (25.0 μL) of different concentrations (from 0.1 nM to 0.1 M) was sequentially injected into 5.0 mL of PBS from the stock solutions and variations of surface current were investigated after every injection using the Ce2S3-CNT NCs/GCE as the WE, which is given in Fig. 5(e). It clearly demonstrates that the current response gradually decreases with the increasing concentration of UA at room temperature for the Ce2S3-CNT NCs/GCE sensor. Aqueous solutions of UA (from 0.01 nM to 1.0 mM) were obtained to investigate the detection limit of the proposed sensor. Fig. 5(f) represents the magnification of the concentration variation plot (Fig. 5(e)) from +0.6 to +1.5 V.
The calibration plot current vs. concentration (at +0.8 V) in the full concentration range is given in Fig. 6(a). A very high sensitivity value (1.5822 μA nM−1 m−2) was calculated from the calibration plot at +0.8 V. The LDR of the proposed sensor was obtained from 0.1 nM to 1.0 mM (r2 = 0.9972) and the LOD was calculated as 60.0 ± 0.05 pM [3 × noise (N)/slope (S)]. Fig. 6(b) represents the repeatability of the I–V responses with the as-grown Ce2S3-CNT NC-coated GCE using 25.0 μL of 0.1 μM UA solutions with nine different working electrodes in Run 1 – Run 9 under identical conditions. Almost the same current response in nine repeated experiments confirmed the excellent repeatability of the sensor (RSD = 3.52%, n = 9). This small % RSD may be due to the mass variation of the coating materials Ce2S3-CNT NCs in the working electrodes. When the same working electrode was used in different solutions of the same concentration, even under identical conditions, current response slightly decreased. This is because after each run, the number of the active sites of the Ce2S3-CNT NCs slightly decreases.
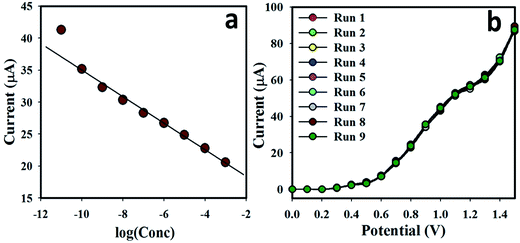 |
| Fig. 6 (a) Calibration plot of Ce2S3-CNT NC-fabricated GCE surfaces. Potential range from 0.0 to +1.5 V and (b) repeatability using different WE (25.0 μL; 0.1 μM UA). | |
4.3. Application to real samples
To confirm the validity of the I–V method, the Ce2S3-CNT NCs/GCE was used to quantify UA in two real aqueous UA solutions. For this purpose, we used the standard addition method to check the precision of the UA detection in aqueous samples (human urine and rabbit serum; Diluted 10 times in the buffer). A fixed amount (∼25.0 μL) of real water sample was mixed and analyzed in PBS (5.0 mL) by the Ce2S3-CNT NCs/GCE working electrodes. Table 1 shows the results, which demonstrated that the amount of UA in the rabbit serum is higher than that in the urine sample. Based on the results, therefore, we can conclude that the I–V method is suitable, consistent, and appropriate in real sample analysis with the Ce2S3-CNT NCs/GCE system.
Table 1 Quantification of uric acid in real samples
Real samples |
Measured current (μA) |
Respective concentration (nM) |
Human urine (10 times diluted) |
60.4 |
∼1.50 ± 0.02 |
Rabbit serum (10 times diluted) |
78.3 |
∼2.12 ± 0.02 |
The resistance of the fabricated nanocomposites sensor decreases with the increasing electron communication, characteristics that are important features of the nanomaterial at RTP and vice versa.54–58 During the reduction of UA, the number of electrons in the conduction band decreases and hence it increases the resistance of the Ce2S3-CNT NCs. Reduction of UA on the Ce2S3-CNT NCs surface is the main phenomenon involved in this proposed UA sensor. Due to its mesoporous structure, Ce2S3-CNT NCs have a large surface area that may be responsible for this sensitive reduction at room temperature. The rate of the UA reduction in Ce2S3-CNT NCs was higher than that of other analytes, even under identical conditions, as shown in Fig. 5(b). When UA is reduced on the Ce2S3-CNT NCs, it removes electrons from the conduction band of the nanocomposites, which increases the resistance of the coating material Ce2S3-CNT NCs.59
Current response in the I–V method during uric acid detection largely depends on the dimensions, morphology, and nanoporosity of the nanocomposites. When Ce2S3-CNT NCs surface is exposed to the oxidizing UA, a surface-mediated reduction reaction takes place. Removal of the conducting electrons from the Ce2S3-CNT NCs/GCE decreases the surface conductance of the electrode. This removal of electrons quickly decreases the conductance of the Ce2S3-CNT NCs coating. Massive deposition of the γ-Ce2S3 NPs on the CNT (white-spots in Fig. 2(a) and (b)) with porous morphology will increase the reducing power of the Ce2S3-CNT NCs. The Ce2S3-CNT NCs/GCE sensor requires approximately 10 s to achieve a constant current in the I–V measurements. It was practical to measure the response time, and the I–t plot is presented in the ESI (ESM, Ψ; Fig. S1†). This excellent sensitivity and high electrochemical performance of the Ce2S3-CNT NCs is due to the mesoporous surface that enhances the reduction of UA. The Ce2S3-CNT NCs/GCE sensor is more sensitive and has a lower detection limit than other sensors already reported for UA detection,1–3,16–20,60 as given in Table 2. Having a large surface area, the Ce2S3-CNT NCs offered a positive nanoenvironment during the detection and quantification of UA. The Ce2S3-CNT NCs/GCE sensor has also shown better reliability and stability. Despite these developments, there are still numerous important apprehensions that must be investigated before the commercial production of this sensor.
Table 2 Analytical performance comparison of different detection methods for uric acid using various nanocomposites or nanomaterials on conventional electrodes
Electrode fabrication |
Technique/method |
Linear dynamic range (LDR) |
Lower detection limit (LOD) |
Sensitivity |
pH |
Ref. |
Pristine graphene |
CV + amperometry |
6.00–1330 μM |
4.82 μM |
0.1029 μA μM−1 |
7.0 |
1 |
Mesoporous NiO |
CV |
Up to 0.374 mM |
0.005 μM |
0.7562 μA μM−1 cm−2 |
— |
2 |
Gold nanocluster |
Fluorescence |
7.0 × 10−7 to 8.0 × 10−5 M |
1.2 × 10−7 M |
— |
7.0 |
3 |
Au–Pt bimetallic nanoclusters |
CV |
0.125 μM to 8.28 × 10−2 M |
4.07 × 10−2 μM |
— |
7.0 |
16 |
Polypyrrole films/Fe-substrate |
CV |
100–5000 μM |
23.0 μM |
0.0046 μA μM−1 |
7.0 |
17 |
rGO/ZnS nanocomposites |
DPV |
1.0–500 μM |
0.4 μM |
— |
7.0 |
18 |
Calixarene film |
CV |
0.4–40 μM |
0.08 μM |
— |
— |
19 |
Flexible graphene fibers |
CV |
10–26 μM |
0.2 μM |
— |
7.0 |
20 |
Silver nanoprism |
Colorimetry |
1.0–40 μM |
0.7 μM |
— |
— |
60 |
Ce2S3/CNT NCs/Nafion/GCE |
I–V |
0.1 nM to 1.0 mM |
60.0 ± 0.05 pM |
1.5822 μA nM−1 m−2 |
7.0 |
This work |
5. Conclusions
In conclusion, we successfully fabricated GCE by the as-grown Ce2S3-CNT NCs with 5% ethanolic Nafion as the conducting coating binder for the first time to act as a selective chemical sensor for the detection of uric acid. Facile Ce2S3-CNT NCs were prepared by a wet-chemical method at low temperature, which is considered as the simplest, convenient, and economical method for the metal-sulphide nanocomposites preparation. The UA biological sensor was studied with Ce2S3-CNT NCs/Nafion/GCE by the simple I–V technique at room conditions. The analytical parameters were thoroughly investigated in terms of sensitivity, limit of detection, and storage ability as well as reproducibility. Significant research activities including synthesis, structural and optical characterization, and chemical-sensing application of the Ce2S3-CNT NCs towards the UA have been included in this study. Crystallinity, morphology, optical properties, band-gap, and binding energies were investigated by XRD, FESEM, FTIR, UV/vis spectroscopy, and XPS methods, respectively. The proposed Ce2S3-CNT NCs/GCE-based UA sensor exhibits the higher-sensitivity (1.5822 μA nM−1 m−2) and very low detection limit (LOD = 60.0 ± 0.05 pM) with excellent linear response (r2 = 0.9972) in a wide range of concentrations (from 0.1 nM to 1.0 mM) within a short response time. This noble approach is a well-organized and reliable technique of an effective biological sensor development for the detection of biological samples as well as biomolecules in the health care field.
Acknowledgements
This project was funded by the Center of Excellence for Advanced Materials Research (CEAMR), King Abdulaziz University, Jeddah, under grant no. (CEAMR-SG-3-438).
References
- S. Qi, B. Zhao, H. Tang and X. Jiang, Determination of ascorbic acid, dopamine, and uric acid by a novel electrochemical sensor based on pristine graphene, Electrochim. Acta, 2015, 161, 395–402 CrossRef CAS.
- W. Huang, Y. Cao, Y. Chen, Y. Zhou and Q. Huang, 3-D periodic mesoporous nickel oxide for nonenzymatic uric acid sensors with improved sensitivity, Appl. Surf. Sci., 2015, 359, 221–226 CrossRef CAS.
- P. Xu, R. Li, Y. Tu and J. Yan, A gold nanocluster-based sensor for sensitive uric acid detection, Talanta, 2015, 144, 704–709 CrossRef CAS PubMed.
- P. E. Erden and E. Kilic, A review of enzymatic uric acid biosensors based on amperometric detection, Talanta, 2013, 107, 312–323 CrossRef CAS PubMed.
- L. Luo, F. Li, L. Zhu, Y. Ding, Z. Zhang, D. Deng and B. Lu, Simultaneous determination of epinephrine and uric acid at ordered mesoporous carbon modified glassy carbon electrode, Anal. Methods, 2012, 4, 2417–2422 RSC.
- K. Jindal, M. Tomar and V. Gupta, Nitrogen-doped zinc oxide thin films biosensor for determination of uric acid, Analyst, 2013, 138, 4353–4362 RSC.
- J. P. Dong, Y. Y. Hu, S. M. Zhu, J. Q. Xu and Y. J. Xu, A highly selective and sensitive dopamine and uric acid biosensor fabricated with functionalized ordered mesoporous carbon and hydrophobic ionic liquid, Anal. Bioanal. Chem., 2010, 396, 1755–1762 CrossRef CAS PubMed.
- W. R. Liu, Modern Clinical Laboratory Diagnostics, Chemical Industry Press, Beijing, 2009 Search PubMed.
- N. Maleki, A. Safavi, E. Farjami and F. Tajabadi, Palladium nanoparticle decorated carbon ionic liquid electrode for highly efficient electrocatalytic oxidation and determination of hydrazine, Anal. Chim. Acta, 2008, 611, 151 CrossRef CAS PubMed.
- U. P. Azad and V. Ganesan, Determination of hydrazine by polyNi(II) complex modified electrodes with a wide linear calibration range, Electrochim. Acta, 2011, 56, 5766 CrossRef CAS.
- F. Li, B. Zhang, S. Dong and E. Wang, A novel method of electrodepositing highly dispersed nano palladium particles on glassy carbon electrode, Electrochim. Acta, 1997, 42, 2563 CrossRef CAS.
- J. Gu, X. Yang, C. Li and K. Kou, Synthesis of Cyanate Ester Microcapsules via Solvent Evaporation Technique and Its Application in Epoxy Resins as a Healing Agent, Ind. Eng. Chem. Res., 2016, 55, 10941–10946 CrossRef CAS.
- J. Gu, C. Liang, J. Dang, W. Dong and Q. Zhang, Ideal dielectric thermally conductive bismaleimide nanocomposites filled with polyhedral oligomeric silsesquioxane functionalized nanosized boron nitride, RSC Adv., 2016, 6, 35809–35814 RSC.
- J. Gu, X. Meng, Y. Tang, Y. Li, Q. Zhuang and J. Kong, Hexagonal boron nitride/polymethyl–vinyl siloxane rubber dielectric thermally conductive composites with ideal thermal stabilities, Composites, Part A, 2017, 92, 27–32 CrossRef CAS.
- J. Gu, X. Yang, Z. Lv, N. Li, C. Liang and Q. Zhang, Functionalized graphite nanoplatelets/epoxy resin nanocomposites with high thermal conductivity, Int. J. Heat Mass Transfer, 2016, 92, 15–22 CrossRef CAS.
- H. Liu, J. Gao, W. Huang, K. Dai, G. Zheng, C. Liu, C. Shen, X. Yan, J. Guo and Z. Guo, Electrically conductive strain sensing polyurethane nanocomposites with synergistic carbon nanotubes and graphene bifillers, Nanoscale, 2016, 8, 12977–12989 RSC.
- H. Liu, W. Huang, X. Yang, K. Dai, G. Zheng, C. Liu, C. Shen, X. Yan, J. Guo and Z. Guo, Organic vapor sensing behaviors of conductive thermoplastic polyurethane–graphene nanocomposites, J. Mater. Chem. C, 2016, 4, 4459–4469 RSC.
- H. Liu, Y. Li, K. Dai, G. Zheng, C. Liu, C. Shen, X. Yan, J. Guo and Z. Guo, Electrically conductive thermoplastic elastomer nanocomposites at ultralow graphene loading levels for strain sensor applications, J. Mater. Chem. C, 2016, 4, 157–166 RSC.
- Y. Liu, P. She, J. Gong, W. Wu, S. Xu, J. Li, K. Zhao and A. Deng, A novel sensor based on electrodeposited Au–Pt bimetallic nano-clusters decorated on graphene oxide (GO)–electrochemically reduced GO for sensitive detection of dopamine and uric acid, Sens. Actuators, B, 2015, 221, 1542–1553 CrossRef CAS.
- D. Oukil, L. Benhaddad, R. Aitout, L. Makhloufi, F. Pillier and B. Saidani, Electrochemical synthesis of polypyrrole films doped by ferrocyanide ions onto iron substrate: application in the electroanalytical determination of uric acid, Sens. Actuators, B, 2014, 204, 203–210 CrossRef CAS.
- Y. J. Yang, One-pot synthesis of reduced graphene oxide/zinc sulfide nanocomposite at room temperature for simultaneous determination of ascorbic acid, dopamine and uric acid, Sens. Actuators, B, 2015, 221, 750–759 CrossRef CAS.
- F. Wang, C. L. Chi, B. Yu and B. Ye, Simultaneous voltammetric determination of dopamine and uric acid based on Langmuir–Blodgett film of calixarene modified glassy carbon electrode, Sens. Actuators, B, 2015, 221, 1586–1593 CrossRef CAS.
- W. Cai, J. Lai, T. Lai, H. Xie and J. Ye, Controlled functionalization of flexible graphene fibers for the simultaneous determination of ascorbic acid, dopamine and uric acid, Sens. Actuators, B, 2016, 224, 225–232 CrossRef CAS.
- E. Ergün, S. Kart, D. K. Zeybek and B. Zeybek, Simultaneous electrochemical determination of ascorbic acid and uric acid using poly(glyoxal-bis(2-hydroxyanil)) modified glassy carbon electrode, Sens. Actuators, B, 2016, 224, 55–64 CrossRef.
- Y. Temerk and H. Ibrahim, A new sensor based on In doped CeO2 nanoparticles modified glassy carbon paste electrode for sensitive determination of uric acid in biological fluids, Sens. Actuators, B, 2016, 224, 868–877 CrossRef CAS.
- A. K. Bhakta, R. J. Mascarenhas, O. J. D'Souza, A. K. Satpati, S. Detriche, Z. Mekhalif and J. Dalhalle, Iron nanoparticles decorated multi-wall carbon nanotubes modified carbon paste electrode as an electrochemical sensor for the simultaneous determination of uric acid in the presence of ascorbic acid, dopamine and L-tyrosine, Mater. Sci. Eng. C, 2015, 57, 328–337 CrossRef CAS PubMed.
- G. Zhang, P. He, W. Feng, S. Ding, J. Chen, L. Li, H. He, S. Zhang and F. Dong, Carbon nanohorns/poly(glycine) modified glassy carbon electrode: preparation, characterization and simultaneous electrochemical determination of uric acid, dopamine and ascorbic acid, J. Electroanal. Chem., 2016, 760, 24–31 CrossRef CAS.
- C. Alippi, A unique timely moment for embedding intelligence in applications, CAAI Transactions on Intelligence Technology, 2016, 1, 1–3 CrossRef.
- H. Jin, Q. Chen, Z. Chen, Y. Hu and J. Zhang, Multi-LeapMotion sensor based demonstration for robotic refine tabletop object manipulation task, CAAI Transactions on Intelligence Technology, 2016, 1, 104–113 CrossRef.
- J. C. Xing, Y. L. Zhu, Q. W. Zhou, X. D. Zheng and Q. J. Jiao, Fabrication and shape evolution of CoS2 octahedrons for application in super capacitors, Electrochim. Acta, 2014, 136, 550–556 CrossRef CAS.
- W. X. Mao, W. Zhang, Z. X. Chi, R. W. Lu, A. M. Cao and L. J. Wan, Core–shell structured Ce2S3@ZnO and its potential as a pigment, J. Mater. Chem. A, 2015, 3, 2176 CAS.
- S. Yu, D. Wang, Y. Liu, Z. Li, X. Zhang, X. Yang, Y. Wang, X. Wang and H. Su, Preparations and characterizations of γ-Ce2S3@SiO2 pigments from precoated CeO2 with improved thermal and acid stabilities, RSC Adv., 2014, 4, 23653 RSC.
- M. M. Rahman, J. Ahmed and A. M. Asiri, Development of Creatine sensor based on antimony-doped tin oxide (ATO) nanoparticles, Sens. Actuators, B, 2017, 242, 167–175 CrossRef CAS.
- M. M. Hussain, M. M. Rahman, A. M. Asiri and M. R. Awual, Non-enzymatic simultaneous detection of L-glutamic acid and uric acid using mesoporous Co3O4 nanosheets, RSC Adv., 2016, 6, 80511–80521 RSC.
- M. M. Rahman, J. Ahmed, A. M. Asiri, I. A. Siddiquey and M. A. Hasnat, Development of ultra-sensitive hydrazine sensor based on facile CoS2-CNT nanocomposites, RSC Adv., 2016, 6, 90470–90479 RSC.
- M. M. Rahman, J. Ahmed, A. M. Asiri, M. A. Hasnat and I. A. Siddiquey, Development of 4-methoxyphenol chemical sensor based on NiS2-CNT nanocomposites, J. Taiwan Inst. Chem. Eng., 2016, 64, 157–165 CrossRef CAS.
- C. Karuppiaha, S. Palanisamya, S. M. Chena, S. K. Ramarajb and P. Periakaruppan, A novel and sensitive amperometric hydrazine sensor based on gold nanoparticles decorated graphite nanosheets modified screen printed carbon electrode, Electrochim. Acta, 2014, 139, 157–164 CrossRef.
- M. M. Rahman, A. Jamal, S. B. Khan and M. Faisal, Highly Sensitive Ethanol Chemical Sensor Based on Ni-doped SnO2 Nanostructure Materials, Biosens. Bioelectron., 2011, 28, 127–134 CrossRef CAS PubMed.
- X. Zhang, X. Yan, Q. He, H. Wei, J. Long, J. Guo, H. Gu, J. Yu, J. Liu, D. Ding, L. Sun, S. Wei and Z. Guo, Electrically Conductive Polypropylene Nanocomposites with Negative Permittivity at Low Carbon Nanotube Loading Levels, ACS Appl. Mater. Interfaces, 2015, 7(11), 6125–6138 CAS.
- H. Wei, D. Ding, X. Yan, J. Guo, L. Shao, H. Chen, L. Sun, H. A. Colorado, S. Wei and Z. Guo, Tungsten Trioxide/Zinc Tungstate Bilayers: Electrochromic Behaviors, Energy Storage and Electron Transfer, Electrochim. Acta, 2014, 132, 58–66 CrossRef CAS.
- R. Akbarzadeh, H. Dehghani and F. Behnoudnia, Sodium thiosulfate-assisted synthesis of NiS2 nanostructure by using nickel(II)–Salen precursor: optical and magnetic properties, Dalton Trans., 2014, 43, 16745 RSC.
- S. Pan, J. Zhu and X. Liu, Preparation, electrochemical properties, and adsorption kinetics of Ni3S2/graphene nanocomposites using alkyl dithiocarbonatio complexes of nickel(II) as single-source precursors, New J. Chem., 2013, 37, 654 RSC.
- H. Liu, M. Dong, W. Huang, J. Gao, K. Dai, J. Guo, G. Zheng, C. Liu, C. Shen and Z. Guo, Lightweight conductive graphene/thermoplastic polyurethane foams with ultrahigh compressibility for piezoresistive sensing, J. Mater. Chem. C, 2017, 5, 73–83 RSC.
- M. M. Hussain, M. M. Rahman and A. M. Asiri, Sensitive L-leucine sensor based on a glassy carbon electrode modified with SrO nanorods, Microchim. Acta, 2016, 183, 3265–3273 CrossRef CAS.
- H. Chen, T. Liu, J. Ren, H. He, Y. Cao, N. Wang and Z. Guo, Synergistic carbon nanotube aerogel – Pt nanocomposites toward enhanced energy conversion in dye-sensitized solar cells, J. Mater. Chem. A, 2016, 4, 3238–3244 CAS.
- M. M. Rahman, B. M. Abu-Zied, M. M. Hasan, A. M. Asiri and M. A. Hasnat, Fabrication of selective 4-amino phenol sensor based on H-ZSM-5 zeolites deposited silver electrodes, RSC Adv., 2016, 6, 48435–48444 RSC.
- S. Guo, J. Liu, S. Qiu, Y. Wang, X. Yan, N. Wu, S. Wang and Z. Guo, Enhancing Electrochemical
Performances of TiO2 Porous Microspheres through Hybridizing with FeTiO3 and Nanocarbon, Electrochim. Acta, 2016, 190, 556–565 CrossRef CAS.
- E. Bi, H. Chen, X. Yang, W. Peng, M. Gratzel and L. Han, A quasi core–shell nitrogen-doped graphene/cobalt sulfide conductive catalyst for highly efficient dye-sensitized solar cells, Energy Environ. Sci., 2014, 7, 2637 CAS.
- P. K. Chu and L. Li, Characterization of amorphous and nanocrystalline carbon films, Mater. Chem. Phys., 2006, 96, 253–277 CrossRef CAS.
- Z. Li, W. Li, H. Xue, W. Kang, X. Yang, M. Sun, Y. Tang and C. S. Lee, Facile fabrication and electrochemical properties of high-quality reduced graphene oxide/cobalt sulfide composite as anode material for lithium-ion batteries, RSC Adv., 2014, 4, 37180 RSC.
- M. Zhang, B. Chen, H. Tang, G. Tang, C. Li, L. Chen, H. Zhang and Q. Zhang, Hydrothermal synthesis and tribological properties of FeS2 (pyrite)/reduced graphene oxide heterojunction, RSC Adv., 2015, 5, 1417–1423 RSC.
- M. M. Rahman, S. B. Khan, M. Faisal, A. M. Asiri and M. A. Tariq, Detection of aprepitant drug based on low-dimensional un-doped iron oxide nanoparticles prepared by solution method, Electrochim. Acta, 2012, 75, 164–170 CrossRef CAS.
- X. Chen, Z. Wang, X. Wang, J. Wan, J. Liu and Y. Qian, Single-Source Approach to Cubic FeS2 Crystallites and Their Optical and Electrochemical Properties, Inorg. Chem., 2005, 44, 951–954 CrossRef CAS PubMed.
- M. M. Rahman and A. M. Asiri, Fabrication of highly sensitive ethanol sensor based on doped nanostructure materials using tiny chips, RSC Adv., 2015, 5, 63252–63263 RSC.
- D. Y. Wang, M. Gong, H. L. Chou, C. J. Pan, H. A. Chen, Y. Wu, M. C. Lin, M. Guan, J. Yang, C. W. Chen, Y. L. Wang, B. J. Hwang, C. C. Chen and H. Dai, Highly Active and Stable Hybrid Catalyst of Cobalt-Doped FeS2 Nanosheets–Carbon Nanotubes for Hydrogen Evolution Reaction, J. Am. Chem. Soc., 2015, 137, 1587–1592 CrossRef CAS PubMed.
- M. M. Rahman, A. Jamal, S. B. Khan, M. Faisal and A. M. Asiri, Highly Sensitive Methanol Chemical Sensor Based on Undoped Silver Oxide Nanoparticles Prepared by a Solution Method, Microchim. Acta, 2012, 178, 99–106 CrossRef CAS.
- D. A. Rice, S. J. Hibble, M. J. Almond, K. A. H. Mohammad and S. P. Pearse, Novel low-temperature route to known (MnS and FeS2) and new (CrS3, MoS4 and WS5) transition-metal sulfides, J. Mater. Chem., 1992, 2, 895–896 RSC.
- M. M. Rahman, S. B. Khan, A. M. Asiri and A. G. Al-Sehemi, Chemical sensor development based on polycrystalline gold electrode embedded low-dimensional Ag2O nanoparticles, Electrochim. Acta, 2013, 112, 422–430 CrossRef CAS.
- L. M. F. Dantas, A. P. dos Reis, S. M. C. N. Tanaka, J. H. Zagal, Y. Y. Chen and A. A. Tanaka, Electrocatalytic oxidation of hydrazine in alkaline media promoted by iron tetrapyridinoporphyrazine adsorbed on graphite surface, J. Braz. Chem. Soc., 2008, 19, 720–726 CrossRef CAS.
- D. Wu, H. F. Lu, H. Xie, J. Wu, C. M. Wang and Q. L. Zhang, Uricase-stimulated etching of silver nanoprisms for highly selective and sensitive colorimetric detection of uric acid in human serum, Sens. Actuators, B, 2015, 221, 1433–1440 CrossRef CAS.
Footnote |
† Electronic supplementary information (ESI) available. See DOI: 10.1039/c6ra27414e |
|
This journal is © The Royal Society of Chemistry 2017 |