DOI:
10.1039/C6RA26183C
(Paper)
RSC Adv., 2017,
7, 10700-10706
Polymerization of polyhedral oligomeric silsequioxane (POSS) with perfluoro-monomers and a kinetic study†
Received
2nd November 2016
, Accepted 1st February 2017
First published on 8th February 2017
Abstract
The polymerization of diphenol polyhedral oligomeric silsequioxane (2OH-DDSQ) with a series of perfluoro-monomers including decafluorobiphenyl, decaflurobenzophenone and decafluorodiphenyl sulfone was studied to obtain the optimized reaction for the preparation of POSS-containing fluorinated poly(arylene ether)s. The kinetic behavior of the polymerizations was studied. All the reactants show high reactivity even at a low temperature (30 °C in DMAc). Results show that the polymerization rate increases with the increasing polarity of the perfluoro-monomers and the polymerization of 2OH-DDSQ with decafluorodiphenyl sulfone exhibits the highest rate constant at low temperatures of 30 °C, 40 °C and 45 °C. All of the reaction systems were slightly influenced by the reaction temperature due to the low activation energy. The thermal properties of the polymers were also studied.
Introduction
Fluoropolymers are very attractive for a broad range of advanced applications, because of their excellent optical and electronic properties, high thermal and chemical stabilities and low surface tension.1–3 However, fully fluorinated polymers such as polytetrafluoroethylene usually have high crystallinity which results in poor processability. Therefore, many partially fluorinated polymers with improved processability have been studied.4 Among them, poly(arylene ether)s containing perfluoro-monomers have shown relatively high fluorine content as well as easy processability which have been applied in low dielectric constant materials,5 optical waveguide devices6 and fluoride sensors.7
However, preparation of the poly(arylene ether)s from perfluoro-monomers which contain strong electro-withdrawing groups is difficult.8,9 The preparation of the traditional poly(arylene ether)s are usually following a nucleophilic aromatic substitution (SNAr) mechanism by a diphenol and an aromatic difluoride via a one-step procedure. In the procedure, a Meisenheimer complex is formed when the phenoxide added to the aromatic difluoride and the formation of the Meisenheimer complex is the rate determining step.10 Therefore, the electron-withdrawing groups of the aromatic difluoride which can stabilize the negative charge on the Meisenheimer complex will further activate the monomers and promote the overall reaction. For the perfluoro-monomers such as decafluorobiphenyl, not only the 2 para-fluorines but also the 4 ortho-fluorines of the monomers are activated for the polymerization because of the strong electro-withdrawing effect of the additional fluorine atoms and the polymerization with monomers of multiple sites may result in branched and crosslinked structures. To obtain linear chain structure polymers which possess better solubility and flexibility in adjustability of the chemical structure, side reactions should be suppressed. Consequently, considering the difference in the reactivity of para and ortho-fluorines, a mild reaction condition such as low temperature should be used to suppress the side reaction for obtaining linear chain structure polymers with high molecular weights.11
In this paper, a diphenol POSS with good nucleophilicity is used to copolymerize perfluoro-monomers with different polarities via a two-step nucleophilic substitution procedure to prepare high molecular weight poly(arylene ether)s free of any crosslinked structure.12 POSS is a class of nanoscale molecule with an inorganic silicon and oxygen framework covered by organic substituents.13 Incorporating POSS into a polymer materials can dramatically improve its mechanical properties as well as thermal stability,14,15 nonflammability,16,17 oxidative resistance18 etc. A lot of POSS-containing materials have been synthesized for porous materials,19,20 magnetic materials,21,22 optical materials,23,24 superhydrophobic materials,25,26 catalyst supports and biomedical applications, such as scaffolds for drug delivery, imaging reagents, and combinatorial drug development.27,28 With POSS incorporated, poly(arylene ether)s are expected to possess desirable properties of organic and inorganic compounds.19–42
To suppress the side reaction, in this approach, the diphenol is first converted to phenoxide at a high temperature and then further reacted with perfluoro-monomers at a low temperature. The effects of reaction temperature and time were investigated and the kinetic equation and activation energy were evaluated to study the effect of the monomer polarities on the reaction and summarize the optimal condition of the polymerization. Theoretically, polymers containing both POSS and fluorine are usually with wide application potency.43–47 Meantime, many functional groups can be easily introduced into the polymers because of the mild reaction conditions. The design and preparation of the polymers are already on the way.
Experimental section
Materials
The diphenol POSS (2OH-DDSQ) was synthesized in our laboratory according to the literature12 by hydrosilylation between 3,13-dihydro octaphenyl double-deckersilsesquioxane and eugenol. The perfluoro-monomers were abbreviated as DFPXs. Decafluorobiphenyl (DFP) and decaflurobenzophenone (DFPK) were purchased from Sigma-Aldrich and purified by recrystallization from 2-propanol prior to use. Decafluorodiphenyl sulfone (DFPS) was prepared according to reported methods.7 All other chemicals were obtained from commercial sources and used without further purification.
Methods
Thermogravimetric analysis (TGA) was performed to assess the thermal stability of membranes using a Perkin Elmer Pryis 1 TGA thermal analyzer under air atmosphere. Before performing the analysis, the samples were dried and kept inside the TGA furnace, which was isothermal at 100 °C for 20 min. Then, the samples were reheated up to 800 °C at 10 °C min−1, and the temperatures that resulted in 5% and 10% weight losses were recorded for each sample. Differential Scanning Calorimeter (DSC) measurements were performed using a Mettler Toledo DSC821e instrument at a heating rate of 20 °C min−1 from 40 °C to 250 °C for DDSQ-DFPXs under nitrogen atmosphere. IR spectra (KBr pellets or films) were conduct on a Nicolet Impact 410 Fourier Transform Infrared Spectrometer (FTIR) at room temperature (25 °C). The samples were scanned within the range of 400–3200 cm−1. 1H NMR and 19F NMR spectra were obtained using Brüker Advance 400 spectrometer (400 MHz). Gel permeation chromatograms were obtained using a Waters 410 instrument with dimethylformamide (DMF) as an eluant, at a flow rate of 1 mL min−1 using polystyrene as a standard. The crystallization behaviour of hybrid polymers were studied using a Rigaku D/max-2500 X-ray diffractometer with CuKα radiation (λ = 0.154 nm) as the X-ray source.
Polymer synthesis
As shown in Scheme 1, DDSQ-DFP was synthesized via a two-step nucleophilic aromatic substitution procedure. To a 25 mL three-necked flask equipped with a mechanical stirrer, a nitrogen inlet, and a Dean–Stark trap with a condenser was added 2OH-DDSQ (1.4822 g, 1 mmol), DFP (0.3341 g, 1 mmol), anhydrous K2CO3 (0.1658 g, 1.2 mmol), DMAc (10 mL) and toluene (4.5 mL). The system was allowed to reflux for 3 h, and then the toluene was removed. After removing toluene, the reaction mixture was cooled to 30 °C and then DFP was added. The temperature was maintained for 8 h before the polymerization completed. During the procedure, a small number of reaction solution was removed at various reaction times for 19F NMR analysis. The final viscous solution was then poured into 50 mL of ethanol. The polymer was refluxed in deionized water and ethanol several times to remove the salts and solvents and was dried at 120 °C for 24 h (90% yield). 1H NMR (400 MHz, DMSO-d6), δ (ppm): 0.14 (m), 0.63 (m), 1.56 (m), 2.42 (s), 3.68 (s), 7.37 (m). 19F NMR (400 MHz, DMSO-d6), δ (ppm): −139.3 (4F), −155.43 (4F). IR (KBr cm−1): 2927 (C–H stretching), 1643, 1507 (phenyl ring), 1304 (C–F stretching), 1071, 480 (Si–O stretching), 725 (Si–C stretching).
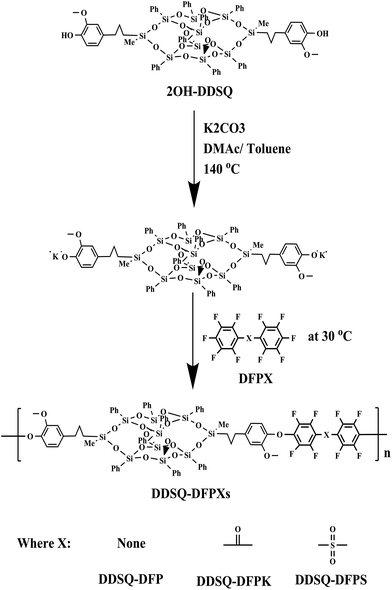 |
| Scheme 1 Reaction route for the preparation of DDSQ-DFPXs. | |
The other two polymers were prepared from the corresponding decafluorodiphenyl monomers and 2OH-DDSQ using the same procedure as described above with the reaction time and reaction temperature listed in Table 1. The molecular weights of the polymers were measured with GPC and listed in Table 1.
Table 1 The preparation and characterization of DDSQ-DFPXs
DDSQ-DFPXs |
Time (h) |
Mn (kDa) |
PDI |
Conversion values (%) |
Tg (°C) |
Td5 (°C) |
Residue (%) |
DDSQ-DFP |
8 |
25.3 |
2.13 |
92.7 |
158 |
417 |
32.9 |
DDSQ-DFPK |
6 |
21.6 |
2.25 |
91.5 |
144 |
408 |
31.9 |
DDSQ-DFPS |
4.5 |
24.8 |
2.31 |
92.6 |
151 |
358 |
31.2 |
DDSQ-DFPK (87% yield). 1H NMR (400 MHz, DMSO-d6), δ (ppm): 0.12 (m), 0.64 (m), 1.55 (m), 2.36 (s), 3.55 (s), 7.376 (m). 19F NMR (400 MHz, DMSO-d6),δ (ppm): −143.3 (4F), −155.4 (4F). IR (KBr cm−1): 2925 (C–H stretching), 1639, 1490 (phenyl ring), 1302 (C–F stretching), 1069, 475 (Si–O stretching), 720 (Si–C stretching).
DDSQ-DFPS (89% yield). 1H NMR (400 MHz, DMSO-d6), δ (ppm): 0.11 (m), 0.65 (m), 1.51 (m), 2.35 (s), 3.77 (s), 7.37 (m). 19F NMR (400 MHz, DMSO-d6), δ (ppm): −138.7 (4F), −154.5 (4F). IR (KBr cm−1): 2920 (C–H stretching), 1635, 1483 (phenyl ring), 1298 (C–F stretching), 1069, 478 (Si–O stretching), 720 (Si–C stretching).
Results and discussion
Polymerization of 2OH-DDSQ with DFPXs
The polymerization of DFPXs and 2OH-DDSQ was carried out in a two-step nucleophilic aromatic substitution procedure. In this approach, the 2OH-DDSQ was first converted to phenoxide and then further reacted with DFPXs at a low temperature of 30 °C. The reactions are displayed in Scheme 1 and analyzed by 19F NMR in Fig. 1. It can be seen that the produced polymers have clean 19F NMR spectra and the two major peaks are assigned to the fluorine atoms on the main chain as indicated in the Fig. 1. The only three tiny peaks correspond to the fluorine atoms on the end group. This means that the side reactions in the ortho, and meta fluorine atoms are strongly suppressed under the mild condition. Actually the reaction at a temperature of 60 °C for DFP and 2OH-DDSQ could yield crosslinked gels in only 15 minutes and the temperatures for the other reactions to get crosslinked gels are even lower.
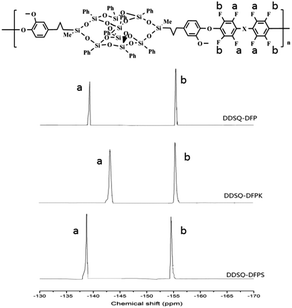 |
| Fig. 1 19F NMR spectra of the DDSQ-DFPXs in DMSO-d6. | |
To investigate the reaction process of the DDSQ-DFPXs, as an example, Fig. 2 shows the 19F NMR spectra of DDSQ-DFP at different reaction times. As shown in Fig. 2, as the reaction time increased, two new peaks appeared (−139.3 (a), −155.4 (b) ppm) and increased in intensity which were attributed to the ortho and meta fluorine atoms on the main chain of the polymer. Meanwhile, three other peaks decreased (−138.5 (ppm), −150.1 (ppm), −160.9 (ppm)) in density, which were attributed to the ortho, para and meta fluorine atoms of the DFP end units in the polymer. From the Fig. 2 it can be seen that at the reaction time of 8 h, the peaks of ortho, and meta-fluorine atoms can barely be seen. At the end this period about 92.7% of DFP was converted to the di-substituted compound. Moreover, when the reaction time gets longer, the solution becomes highly viscous and a gel-like substance formed. However, the isolated polymer from the solution was found to be soluble in DMAc and there is no extra peaks in the 19F NMR spectra indicating no branched or crosslinked structure in the gel-like substance. The solubility of the DDSQ-DFP becomes poor and precipitate from the solution when the molecule weight gets high. From the Table 1, it can be seen that the molecular weight of DDSQ-DFPXs are relatively high compared with the other POSS-containing polymers though the numbers of the repeating units in the polymers are all less than 15.
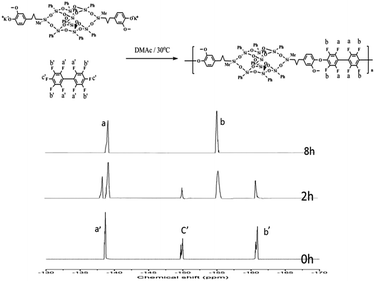 |
| Fig. 2 19F NMR spectra of DDSQ-DFP at different reaction times. | |
The polymers were also identified by FTIR and 1H NMR spectroscopy (Fig. 3). The FTIR spectra of the DDSQ-DFPXs show characteristic absorption bands around 1650 cm−1 and 1304 cm−1, corresponding to aryl carbonyl groups and carbon–fluorine bonds. Fig. 3 shows the 1H NMR of DDSQ-DFPXs. Signals of proton at 0.13, 0.65–2.4 and 3.56 ppm are assigned to the protons of methyl, methylene, and methoxy. The peaks at 6.2–8.5 are assigned to the protons of aromatic rings.
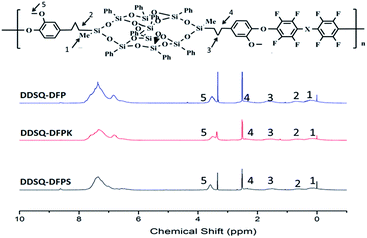 |
| Fig. 3 1H NMR spectra of DDSQ-DFPXs in DMSO-d6. | |
Kinetic study of the reaction
As a two-step nucleophilic aromatic substitution procedure polymerization, the diphenol was first converted to phenoxide and then further reacted with DFPXs at a low temperature. The first procedure is not directly participating in the polymerization. And the second procedure can be explained by the Scheme 2, following the nucleophilic aromatic substitution (SNAr) mechanism. Typically, the formation of Meisenheimer complex is the rate-determining step.
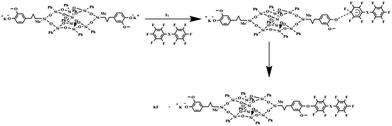 |
| Scheme 2 Reaction mechanism for the polymerization. | |
The rate expression Rp for polymerization in Scheme 2 can be described as the following eqn (1):
|
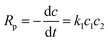 | (1) |
c1: molar concentration of DFPXs;
c2: molar concentration of the phenoxide;
k1: the reaction rate constant of polymerization and the rate constant for the formation of the Meisenheimer. It was assumed that the molar concentrations of DFPXs and the phenoxide were equal and described as
c. The above equation can be simplified as follow:
|
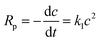 | (2) |
indicating a second-order reaction.
After integration, the equation was shown as
|
 | (3) |
with
c0, the initial concentration of the DFPXs and phenoxide and
c, the concentration of the DFPXs and phenoxide at time
t. There is an equation between
c and
c0 following
c =
c0 (1 −
p) and
p is the conversion at reaction time
t. So the
eqn (2) can be further described as the following
eqn (4):
|
 | (4) |
By comparing the integral intensity of the fluorine atoms in the 19F NMR spectra, the conversion at different reaction times can be calculated following p = (F0 − Ft)/F0 with F0 the initial concentration of para-fluorine and Ft the concentration of para-fluorine at time t. The relation of the 1/(1 − p) with the reaction time t in the related three reaction systems are presented in the Fig. 5. It can be found that the experimental data can fit the equation when the conversion is less than 83% reflecting the SNAr mechanism of the reaction. According to the eqn (1) and Fig. 4, the reaction rate constant at 30 °C can be calculated due to the slope of the curve. The values of the reaction rate constants are shown to be 0.271, 0.307, 0.483 L mol−1 min−1 which increase with the increasing of polarity of the DFPXs.
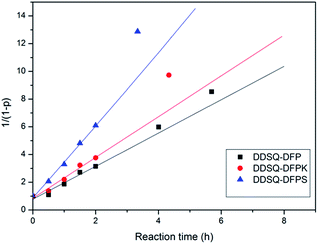 |
| Fig. 4 Dependence of 1/(1 − p) on the reaction time for the DDSQ-DFPXs produced in DMAc at 30 °C. | |
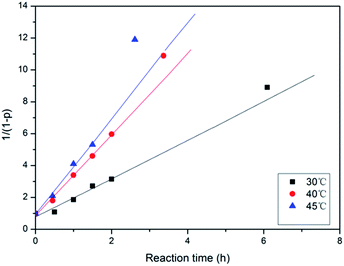 |
| Fig. 5 Dependence of 1/(1 − p) on the reaction time for the DDSQ-DFP produced in DMAc at 30 °C, 40 °C and 45 °C. | |
Activation energy of the polymerization
Since the present reaction was conducted at a temperature of 30 °C, for the calculation of the activation energy and investigation of the temperature effect on the reaction system, the reaction was also investigated at various temperatures. Kinetic parameters of the reaction systems are listed in Table 2. As an example, Fig. 6 shows the variation of 1/(1 − p) with the reaction time for the DDSQ-DFP produced in DMAc at 30 °C, 40 °C and 45 °C. It can be seen that increasing the temperature from 30 °C to 45 °C results in an about 1.5-fold increase in the rate constant reflecting a low activation energy. The activation energy Ea values are calculated following the Arrhenius equation: |
 | (5) |
T: temperature in K; R: universal gas constant with the value of 8.314 J mol−1 K−1; A: pre-exponential factor. The equation can be transformed into the following eqn (6): |
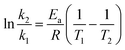 | (6) |
k1: rate constant at T1; k2: rate constant at T2.
Table 2 Kinetic parameters of the reaction systems; rate constant k and activation energy Ea
DDSQ-PAEKs |
T (°C) |
k (L mol−1 min−1) |
Ea (kJ mol−1) |
DDSQ-DFP |
30 |
0.271 |
32.14 |
40 |
0.414 |
45 |
0.503 |
DDSQ-DFPK |
30 |
0.307 |
27.52 |
40 |
0.431 |
45 |
0.510 |
DDSQ-DFPS |
30 |
0.483 |
25.11 |
40 |
0.643 |
45 |
0.739 |
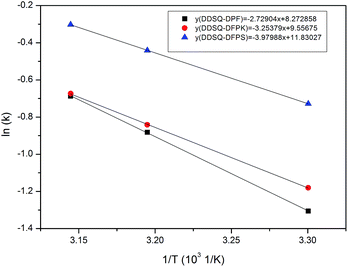 |
| Fig. 6 Dependence of ln k on the reaction temperature for the DDSQ-DFPXs produced in DMAc at 30 °C, 40 °C and 45 °C. | |
The variation of ln
k with 1/T for the DDSQ-DFPXs are shown in Fig. 6 and according to the eqn (6) the activation energy values can be calculated from the slopes indicated in the figure. The corresponding R-squares for DDSQ-DFP, DDSQ-DFPK and DDSQ-DFPS are 0.99235, 0.98753, 0.9889 which indicate the high correlation between ln
k and the reaction temperature. The reactions between 2OH-DDSQ and perfluro-monomers are all shown to have low activation energy with the Ea below 35 kJ mol−1.
As a nucleophilic aromatic substitution (SNAr) reaction, the nucleophilicity of the diphenol and the stability of the Meisenheimer complex are important for low activation barriers leading to relatively low activation energy. The phenoxide of the 2OH-POSS has a high nucleophilicity because of the electron-donating effect of the ortho-substituted methoxy and meantime, the electron-withdrawing groups of the perfluoro-monomer further stabilize the Meisenheimer complex. As explained the above two main reasons lead to a relatively low activation energy. It can be seen that the activation energy Ea decreases with the increasing polarity of the DFPXs. This is because the stronger electro-withdrawing effect gets a stabler Meisenheimer complex.
Thermal properties of DDSQ-DFPXs
The thermal properties of the DDSQ-DFPXs were evaluated by DSC and TGA, and the data are listed in Table 1 and the curves are shown in Fig. 7 and 8. No melting endotherms are observed in DSC traces for the DDSQ-DFPXs (Fig. 7), which suggests their amorphous nature. Among the polymers, DDSQ-DFP shows the highest Tg value 158 °C because of the effect of the rigid biphenylene polymer backbone which hinders the movement of the chain segment. With the increasing of polarity of the DFPXs, DDSQ-DFPS exhibits a higher Tg than DDSQ-DFPK, reflecting a higher intermolecular force in the polymer. Meanwhile, the polymers don't show very high Tgs. Though the rigid molecular structure of POSS can lead a higher Tg, both the greatly increased free volume of DDSQ-DFPXs and the flexible aliphatic chains possessed by 2OH-DDSQ results in the easier movement of the chain segment.
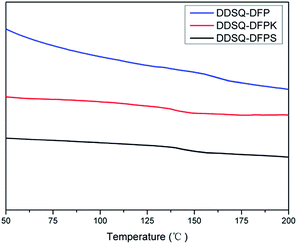 |
| Fig. 7 DSC curves of the DDSQ-DFPXs. | |
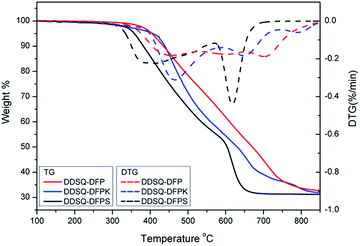 |
| Fig. 8 TGA curves and the derivative curves of the DDSQ-DFPXs. | |
Seen from the TGA data Table 1 and Fig. 8, the temperatures at 5% weight loss (Td5) are in the range of 358–417 °C in air for the DDSQ-DFPXs, indicating a fairly high thermal stability of the polymers. It can be seen from the derivative curves in Fig. 8 that all the polymers display two steps in degradation progress. The first step from 335 °C to 570 °C originated from the degradation of active alkyl chain and the second step from 570 °C to 760 °C originated from the degradation of frameworks, such as the aromatic ring and POSS cage. The DDSQ-DFPXs display high residuals of degradations which are all above 30%. The high yields of degradation residues are ascribed to the ceramic formation from POSS moiety during the thermal decomposition.
Conclusion
The polymerization of 2OH-DDSQ with DFPXs can be conducted at a low temperature of 30 °C in DMAc via a two-step procedure obtaining polymers with high molecular weights and well-defined linear chain structures. The reactants exhibit high reactivity allowing the polymerization to complete in a few hours. The reaction rate increases with the increasing of polarity of the DFPXs and it is found that the reaction rate is not greatly affected with the increasing temperature. The activation energy of the polymerization is shown to be very low and the values decreases with the polarity of the DFPXs. With the well-controlled reaction time, all the produced polymers have great fundamental properties for future application such as solubility and thermal properties. The polymers with POSS and fluorine incorporated in have great application potency.
References
- J. M. Desimone, Z. Guan and C. S. Elsbernd, Science, 1992, 257, 945–947 CAS.
- J. W. Martin, M. M. Smithwick and B. M. Braune, Environ. Sci. Technol., 2004, 38, 373–380 CrossRef CAS PubMed.
- T. R. Dargaville, G. A. George and D. J. T. Hill, Prog. Polym. Sci., 2003, 28, 1355–1376 CrossRef CAS.
- R. Souzy and B. Ameduri, Prog. Polym. Sci., 2005, 30, 644–687 CrossRef CAS.
- J. Ding and M. Day, Macromolecules, 2006, 39, 6054–6062 CrossRef CAS.
- K. Aljoumaa, Y. Qi and J. Ding, Macromolecules, 2009, 42, 9275–9288 CrossRef CAS.
- J. Ding, X. Du and M. Day, Macromolecules, 2007, 40, 3145–3153 CrossRef CAS.
- J. Jiang, C. L. Callender and J. P. Noad, Opt. Eng., 2007, 46, 074601 CrossRef.
- Y. Qi, J. Jiang and C. L. Callender, Appl. Opt., 2006, 45, 7480–7487 CrossRef CAS PubMed.
- A. Jutand and A. Mosleh, Organometallics, 1995, 14, 1810–1817 CrossRef CAS.
- J. Ding, Y. Qi and M. Day, Macromol. Chem. Phys., 2005, 206, 2396–2407 CrossRef CAS.
- W. Zhang, J. Xu and X. Li, J. Polym. Sci., Part A: Polym. Chem., 2014, 52, 780–788 CrossRef CAS.
- W. Zhang and A. H. E. Müller, Prog. Polym. Sci., 2013, 38, 1121–1162 CrossRef CAS.
- H. Y. Xu, S. W. Kuo, J. S. Lee and F. C. Chang, Macromolecules, 2002, 35, 8788–8793 CrossRef CAS.
- H. Y. Xu, B. H. Yang, J. F. Wang, S. Y. Guang and C. Li, Macromolecules, 2005, 38, 10455–10460 CrossRef CAS.
- Q. He, L. Song, Y. Hu and S. J. Zhou, Mater. Sci., 2009, 44, 1308–1316 CrossRef CAS.
- W. C. Zhang, X. M. Li and R. J. Yang, J. Appl. Polym. Sci., 2012, 124, 1848–1857 CrossRef CAS.
- B. H. Augustine, W. C. Hughes, K. J. Zimmermann, A. J. Figueiredo, X. W. Guo, C. C. Chusuei and J. S. Maidment, Langmuir, 2007, 23, 4346–4350 CrossRef CAS PubMed.
- Y. J. Lee, J. M. Huang and S. W. Kuo, Polymer, 2005, 46, 10056–10065 CrossRef CAS.
- S. S. Chen, X. Li, Y. Li and J. Q. Sun, ACS Nano, 2015, 9, 4070–4076 CrossRef CAS PubMed.
- J. C. Huang, C. B. He and Y. Xiao, Polymer, 2003, 44, 4491–4499 CrossRef CAS.
- Y. Ni, S. X. Zheng and K. M. Nie, Polymer, 2004, 45, 5557–5568 CrossRef CAS.
- W. A. Zhang and H. E. Axel Mueller, Prog. Polym. Sci., 2013, 38, 1121–1162 CrossRef CAS.
- Y. D. Zhang, S. H. Lee and M. Yoonessi, Polymer, 2006, 47, 2984–2996 CrossRef CAS.
- S. Srinivasan, S. S. Chhatre and J. M. Mabry, Polymer, 2011, 52, 3209–3218 CrossRef CAS.
- H. Maciejewski, J. Karasiewicz and M. Dutkiewicz, Silicon, 2015, 7, 201–209 CrossRef CAS.
- R. Y. Kannan, H. J. Salacinski and M. Odlyha, Biomaterials, 2006, 27, 1971–1979 CrossRef CAS PubMed.
- J. Wu, Q. Ge and P. T. Mather, Macromolecules, 2010, 43, 7637–7649 CrossRef CAS.
- G. Li, L. Wang and H. Ni, J. Inorg. Organomet. Polym., 2001, 11, 123–154 CrossRef CAS.
- D. B. Cordes, P. D. Lickiss and F. Rataboul, Chem. Rev., 2010, 110, 2081–2173 CrossRef CAS PubMed.
- F. Laoutid, L. Bonnaud and M. Alexandre, Mater. Sci. Eng., R, 2009, 63, 100–125 CrossRef.
- S. H. Phillips, T. S. Haddad and S. J. Tomczak, Curr. Opin. Solid State Mater. Sci., 2004, 8, 21–29 CrossRef CAS.
- S. W. Kuo and F. C. Chang, Prog. Polym. Sci., 2011, 36, 1649–1696 CrossRef CAS.
- H. Xu, S. W. Kuo and J. S. Lee, Macromolecules, 2002, 35, 8788–8793 CrossRef CAS.
- J. Wu and P. T. Mather, Polym. Rev., 2009, 49, 25–63 CrossRef CAS.
- Y. Ni, S. Zheng and K. Nie, Polymer, 2004, 45, 5557–5568 CrossRef CAS.
- B. X. Fu, B. S. Hsiao and S. Pagola, Polymer, 2001, 42, 599–611 CrossRef CAS.
- C. M. Leu, Y. T. Chang and K. H. Wei, Macromolecules, 2003, 36, 9122–9127 CrossRef CAS.
- E. T. Kopesky, T. S. Haddad and R. E. Cohen, Macromolecules, 2004, 37, 8992–9004 CrossRef CAS.
- L. Matejka, A. Strachota and J. Pleštil, Macromolecules, 2004, 37, 9449–9456 CrossRef CAS.
- Y. Ni, S. Zheng and K. Nie, Polymer, 2004, 45, 5557–5568 CrossRef CAS.
- L. Zheng, S. Hong and G. Cardoen, Macromolecules, 2004, 37, 8606–8611 CrossRef CAS.
- Y. S. Ye, W. Y. Chen and Y. Z. Wang, J. Polym. Sci., Part A: Polym. Chem., 2006, 44, 5391–5402 CrossRef CAS.
- Y. Z. Wang, W. Y. Chen and C. C. Yang, J. Polym. Sci., Part B: Polym. Phys., 2007, 45, 502–510 CrossRef CAS.
- A. G. Kannan, N. R. Choudhury and N. Dutta, ACS Appl. Mater. Interfaces, 2009, 1, 336–347 CAS.
- N. Kitamura, T. Hiraoka and K. Tanaka, Bioorg. Med. Chem., 2012, 20, 4668–4674 CrossRef CAS PubMed.
- X. Qiang, X. Ma and Z. Li, Colloid Polym. Sci., 2014, 292, 1531–1544 CAS.
Footnote |
† Electronic supplementary information (ESI) available. See DOI: 10.1039/c6ra26183c |
|
This journal is © The Royal Society of Chemistry 2017 |
Click here to see how this site uses Cookies. View our privacy policy here.