DOI:
10.1039/C6RA20860F
(Paper)
RSC Adv., 2017,
7, 2913-2918
Highly efficient magnetic hyperthermia ablation of tumors using injectable polymethylmethacrylate–Fe3O4
Received
19th August 2016
, Accepted 28th November 2016
First published on 7th December 2016
Abstract
Magnetic hyperthermia is a promising minimally invasive technique for tumor therapy which has drawn much attention. However, the currently used magnetic materials have their limitations. In this study, we developed an injectable, liquid to solid phase transitional magnetic material, polymethylmethacrylate (PMMA)–Fe3O4 designed for highly efficient magnetic hyperthermia ablation of tumors. The PMMA–Fe3O4 was prepared by the incorporation of PMMA and the Fe3O4. The morphology characterization, the magnetic properties and the heating efficiency of PMMA–Fe3O4 were studied. The Fe3O4 particles were evenly distributed in the PMMA and the hysteresis curve of Fe3O4 and PMMA–Fe3O4 indicated that they were magnetic materials. When exposed to an alternating current magnetic field in vitro, the magnetic PMMA–Fe3O4 generated heat. The increased temperature of excised bovine liver was positively correlated to the iron content and time, which suggested that the temperature inside the tumor was controllable. The ablated liver tissue area for 0.1 ml 10% PMMA–Fe3O4 was 1.24 ± 0.28, 1.70 ± 0.57, 2.76 ± 0.31, 4.17 ± 1.07 cm3, respectively, at 60, 120, 180 and 240 s time points. In the in vivo animal experiments, a MB-231 breast cancer xenograft model was obtained in nude mice. In this tumor model, PMMA–Fe3O4 was injected precisely using guided ultrasound imaging. After the injection, the computer tomography images showed that it was well confined in the tumor tissues without any leakage. The tumors were completely ablated by a dose of 0.1 ml, 10% PMMA–Fe3O4 with 180 s exposure time in the magnetic field. Our results demonstrated that PMMA–Fe3O4 was an excellent magnetic material for the localized magnetic hyperthermia ablation of tumors.
1. Introduction
Cancer is a major public health problem in the world1 and the incidence of cancer has increased with the arrival of aging and augmented standards of living.2,3 For most cancer patients, surgical treatment is often the first choice, though surgical resection has limitations and potential complications.4 Thus, minimally invasive techniques such as radio-frequency ablation, microwave ablation and high intensity focused ultrasound (HIFU) has aroused more and more concerns.5 However, they have their limitations as well. For example, HIFU is affected by gas and bone, microwave ablation is not very suitable for deep tumor ablation, and the needle used for radio-frequency ablation is too big, which might cause potential safety problems.6,7
Magnetic hyperthermia is an adjuvant in cancer treatment that is used to improve the treatment effects.8 A temperature range of 41–43 °C is employed for most of the hyperthermia therapy.9 The physical principle of magnetic heating is owed to the Brownian and Neel relaxation,10 which is not affected by gas or bone and is suitable for both superficial and deep tumor ablation. Thus, the technique of magnetic hyperthermia develops very fast and has been applied in clinic already.11,12 The magnetic media is the key factor for magnetic hyperthermia, which includes magnetic nanoparticles, magnetic fluid and ferromagnetic thermoseeds.13,14 For magnetic fluid or magnetic nanoparticles, it is difficult to selectively accumulate in tumor tissue15,16 and can easily leak into the surrounding tissues or blood vessel, which might cause potential safety problems and decrease the therapy efficiency. Ferromagnetic thermoseeds need precision and are relatively big, complex calculating and controlling.17 Recently, Chunyan Xu18 reported a novel material, the calcium phosphate cement (CPC) containing Fe3O4, as an injectable and biodegradable media for the ablation of tumor. This material could form a solid implant inside the tumor to decrease the Fe3O4 leakage from the tumor to the surrounding tissues. However, the setting time19 for CPC is 30–60 minutes20 and the relatively long setting time might permit some of the Fe3O4 particles leak into the surrounding tissues before the liquid material transform to solid implant.
Bearing these limitations in mind, to develop an injectable magnetic media with a faster liquid to solid phase transformation time and without losing the other advantages of CPC is of importance. Polymethylmethacrylate (PMMA) is a material approved by FDA for clinical use. It has good compressive strength, safety and biocompatibility records, which has attracted wide publicity21 and has been used in orthopedic surgery and trauma for fixing arthroplasties.22,23 Moreover, PMMA possesses an ability to transform from liquid to solid state in a much shorter time than CPC (the setting time of PMMA is 12.5 ± 1 minute).24,25 We supposed the magnetic media made from PMMA might confine the Fe3O4 nanoparticles well in the tumor tissue after injection, which should further improve the treatment efficiency of hyperthermia ablation of tumor.
After a careful review of the literature, we found M. Kawashita has already tested the magnetic heating efficiency of the PMMA containing Fe3O4 in vitro. The temperature of PMMA–Fe3O4 could be increased rapidly to over 70 °C within a period of several tens of seconds25 and they thought PMMA–Fe3O4 could be used for high efficient cancer hyperthermia therapy. Unfortunately, they had not tested PMMA–Fe3O4 for cancer treatment neither in vitro cell experiments nor in vivo animal model experiments.
In this study, the injectable and fast liquid–solid phase transitional PMMA–Fe3O4 was prepared and its high efficiency for tumor hyperthermia therapy was studied in vivo.
2. Experimental section
2.1. Preparation of PMMA–Fe3O4
Fe3O4 powder (Chengdu Aike reagent, China) with an average diameter of 20–50 nm, PMMA powder and MMA liquid (Heraeus, Germany) were used. Four kinds of cement were prepared by mixing the PMMA, MMA liquid with the different proportion of iron oxide. The weight ratio of PMMA and MMA liquid was 2/3 (Table 1).26
Table 1 Composition of PMMA–Fe3O4
Cement |
Powder (wt%) |
Liquid (wt%) |
Fe3O4 |
PMMA |
PMMA-0 |
0 |
40 |
60 |
PMMA-5c |
5 |
38 |
57 |
PMMA-10c |
10 |
36 |
54 |
PMMA-15c |
15 |
34 |
51 |
2.2. Structure characteristics of PMMA–Fe3O4
The microstructure of PMMA–Fe3O4 was tested using scanning electron microscope (SEM, JEOC-7800F) and the element analysis was observed by an energy dispersive X-ray spectrometer (EDS, x-MaxN).
2.3. Magnetic properties of the Fe3O4 and PMMA–Fe3O4 powder
PMMA–Fe3O4 was prepared by PMMA and Fe3O4 nanoparticles. The magnetic properties of Fe3O4 and PMMA–Fe3O4 powder were tested by Physical Property Measurement System (PPMS-9) at room temperature. PPMS was developed by Quantum Design which can be used to measure the basic physical properties of materials.27
2.4. Injectability
The liquid PMMA–Fe3O4 was poured into a 2 ml syringe. Then, the PMMA–Fe3O4 was injected into the water to observe the progress of liquid–solid phase transformation.
2.5. Heating efficiency in excised bovine liver
PMMA–Fe3O4 with different iron contents were prepared into 6 mm diameter balls and dried for 24 h. They were embedded into the excised bovine liver pieces (2.5 cm × 2 cm × 2 cm) and exposed to the altering current magnetic field generated by a homemade magnetic hyperthermia machine (frequency 626 kHz, output current 28.6 A, coil diameter 3 cm)28 for 300 s at room temperature. The surface temperature of PMMA–Fe3O4 was acquired by a far-infrared thermometer (Fluke Ti32, Fluke Corporation, USA). The thermal images were analyzed using the Smart View 3.6 software. During treatment and measurement period, liver piece did not show significant shrinkage.
According to the above experiments, the 10% PMMA (PMMA-10c) was the best choice for next experiment. 0.1 ml PMMA-10c was shaped into balls and dried for 24 h. They were implanted into the excised bovine liverpieces (2.5 cm × 2 cm × 2 cm) and exposed to the same altering current magnetic field for 60, 120, 180 and 240 s. Then, the excised bovine liver was split into 2 parts and the coagulation necrosis area was measured by ruler. Based on the following formula, the area was calculated.
where
S1 is the coagulation necrosis area (cm
2),
D1 and
D2 (cm) is the long and short diameter.
2.6. MB-231 human breast cancer xenograft in nude mice
Nine female nude mice (4 weeks old, 19.3 ± 0.8 g) were selected randomly. MB-231 breast cancer cells were collected by centrifugation, dispersed into DMEM cell culture medium and 1 × 105 cells were injected into the back of each nude mouse subcutaneously. After 4 weeks, the tumor volume reached to 0.453 ± 0.109 cm3 which were used to the next experiment. Animals were maintained in accordance with the guidelines of the Ministry of Science and Technology of Health Guide for Care and Use of Laboratory Animals, China, and approved by the institutional ethical committee (IEC) of Second Affiliated Hospital of Chongqing Medical University. Based on the following formula, the tumor volume was calculated.29where V1 is the tumor volume (cm3), D1 and D2 (cm) is the long and short diameter.
2.7. Nude mice MB-231 human breast cancer xenograft
The 9 nude mice were divided into two groups. For group 1, 6 nude mice were selected randomly and were anesthetized by pentobarbital (0.1–0.18 ml/mouse, 1%). 0.1 ml PMMA-10c was injected into the tumor tissues under the real time ultrasound induction (Esoate, L5-12 MHz). After that, CT images (Quantum FX) were acquired to observe the position of PMMA-10c. Then, the nude mice were exposed to the altering current magnetic field for 180 s and the surface temperature of tumor was obtained by infrared thermometer. To acquire the microstructure of ablated tumors, 3 nude mice were euthanized in 24 h after treatment. For group 2, 3 nude mice as control group had no treatment.
3. Results
3.1. Characteristic of PMMA–Fe3O4
As showed in the SEM images [Fig. 1(a)], the Fe3O4 nanoparticles were evenly distributed in PMMA. The element mapping of PMMA–Fe3O4 [Fig. 1(b)] showed that every element was well dispersed in PMMA and the corresponding molecular percentages for C, O and Fe were 48.64, 40.90, 10.46, respectively, [Fig. 1(c)].
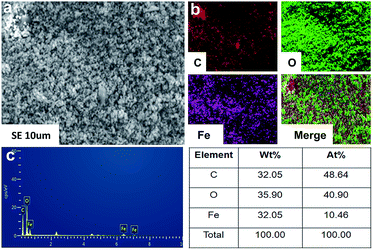 |
| Fig. 1 (a) SEM photographs; (b) and (c) corresponding EDS map scan images for the PMMA–Fe3O4. | |
3.2. Injectability
Fig. 2(a–f) illustrated that liquid PMMA–Fe3O4 could be injected from syringe (the inner diameter of needle: 1.2 mm). When contacted with water, PMMA–Fe3O4 would not be defeated and dispersed. The liquid–solid phase transformation happened after setting time.
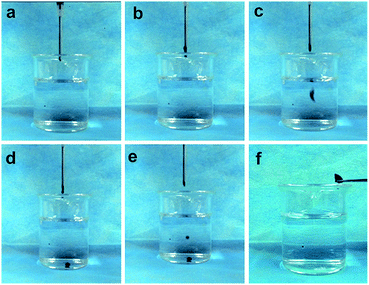 |
| Fig. 2 The process of liquid–solid phase transformation; (a) before the injection; (b–e) injecting liquid PMMA–Fe3O4 through a needle; (f) after the injection. The PMMA–Fe3O4 transformed into solid implant which could be taken out by a tweezers. | |
3.3. Magnetic properties
The hysteresis curve (Fig. 3) showed that the pure Fe3O4 nanoparticles and the PMMA–Fe3O4 were ferromagnetic. The saturation magnetization value (Ms) and the coercive force (Hc) for the pure Fe3O4 nanoparticles were −15.3 emu g−1 and 126.9 Oe. However, the PMMA–Fe3O4 had an Ms of −0.3 emu g−1 and an Hc of 64.5 Oe.
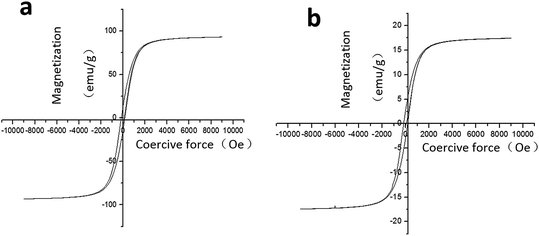 |
| Fig. 3 (a) The hysteresis curve of pure Fe3O4 nanoparticles; (b) the hysteresis curve of PMMA–Fe3O4. | |
3.4. Heating efficiency in vitro
Fig. 4(a) showed that the surface temperature of 0.1 ml PMMA-10c in excised bovine liver increased with the iron contents and time. But for the control group, the temperature of pure PMMA was not changing with the time. The corresponding time–temperature curve [Fig. 4(b)] showed that the surface temperature of PMMA-10c was 44.20 ± 1.13, 51.65 ± 1.62, 56.25 ± 1.2 °C, respectively, at the time point of 60, 180 and 300 s. While the surface temperature of 5% PMMA–Fe3O4 (PMMA-5c) and 15% PMMA–Fe3O4 (PMMA-15c) were 30.70 ± 1.153, 35.83 ± 0.87, 38.60 ± 1.51 °C and 59.25 ± 2.19, 73.15 ± 0.49, 79.70 ± 2.40 °C, at the time point of 60, 180 and 300 s. After being exposed to the altering current magnetic field, the heat generated by the PMMA-10c embedded inside the excised bovine liver changed the color of the surround tissue from a dark red color into a pale white color [Fig. 4(c)]. The coagulation necrosis areas for 0.1 ml PMMA-10c were 1.24 ± 0.23, 1.70 ± 0.57, 2.76 ± 0.31, 4.17 ± 1.07 cm2, respectively, at the time points of 60, 120, 180 and 240 s [Fig. 4(d)].
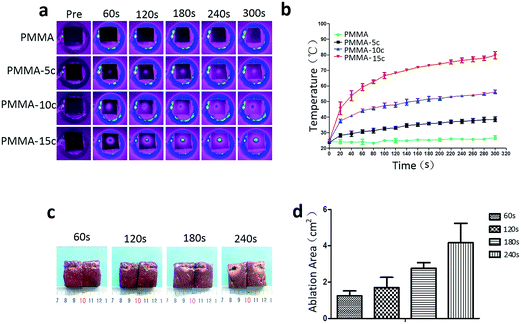 |
| Fig. 4 (a) The thermal images of the PMMA–Fe3O4 with different iron contents at different magnetic induction time point (the pure PMMA was taken as the control group); (b) the corresponding time–temperature curve of PMMA–Fe3O4; (c) macroscopic photos of excised bovine liver (the tissue with a pale white color around the PMMA-10c was the coagulation necrosis tissue); (d) the corresponding coagulation necrosis area. | |
3.5. Heating efficiency in vivo
The ultrasound images [Fig. 5(a)] showed that the echo of the tumor was hypoechoic. The hyperechoic needle was clearly visible in ultrasound imaging which guaranteed the precisely injection of PMMA–Fe3O4 into the tumor. After injection, the PMMA–Fe3O4 appeared hyperechoic inside the tumor tissue. The CT images [Fig. 5(b)] illustrated that PMMA-10c with high intensity inside the tumor. The thermal images [Fig. 5(c)] showed that the surface temperature of the tumor increased with the time when exposed to the altering current magnetic field and reached to 53.05 ± 3.18 °C at 180 s [Fig. 5(d)].
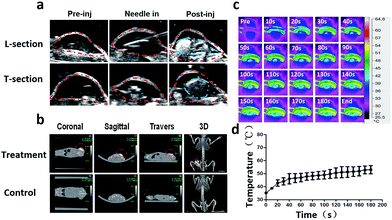 |
| Fig. 5 (a) 0.1 ml PMMA-10c was injected into the tumor under real time guidance of ultrasound imaging. The ultrasound images showed the procedures for injection at two different sections (L section: longitudinal section, T section: transverse section, Pre inj: pre injection, Post inj: post injection, the red dotted line: tumor, the blue dotted line: PMMA-10c); (b) CT images of PMMA-10c (red dotted line: PMMA–Fe3O4); (c) the thermal imaging of the tumor was exposed to the altering current magnetic field for 180 s after injection; (d) the corresponding time–temperature curve. | |
The macroscopic image [Fig. 6(a)] showed the tumor volume was changing with the time after the magnetic hyperthermia ablation in vivo. After the ablation of the tumor for 180 s, the color of the skin on the tumor turned into a pale color immediately. On the first day, the color of the skin on the tumor gradually turned from pale color into black color, and a boundary between the ablated tissue and the non-ablated tissue could be clearly observed. On the third day, the tumor tissue began to form a scab and on the tenth day, the scab began to desquamate. Until the fifteenth day, the scab separated from mice and fell down. Corresponding time–tumor volume curve was showed in [Fig. 6(b)], which revealed that tumor volume decreased with the time after treatment. While in the control group, the tumor volume had an upward tendency. Fig. 6(c) showed that the whole body weights of the mice didn't change with the time. The pathology images showed there was a significant difference between the ablated and non-ablated tumor tissue [Fig. 6(d)]. It could be observed from the tumor tissue that received PMMA-10c followed by ablation that disorder tissue with distinct destructed cells existed. On the other hand, an integrated nucleus and cytoplasm was found in the control group while no evident cell destruction discovered.
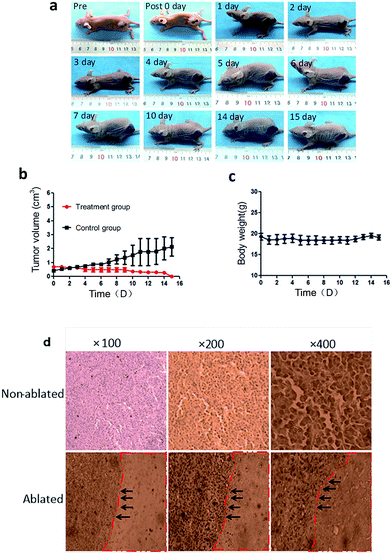 |
| Fig. 6 (a) The macroscopic images showed the change of tumor volume within 15 days after the magnetic hyperthermia ablation; (b) the time–tumor volume curve; (c) the time–body weight curve; (d) the HE staining of the non-ablated and ablated tumor tissue (red dotted line: the ablated tissue, black arrow: boundary between ablated tissue and non-ablated tissue). | |
4. Discussion
In this study, we prepared an injectable, fast liquid to solid phase transitional material, the PMMA–Fe3O4, and confirmed its high efficiency for the magnetic hyperthermia therapy of tumor in vivo.
The results showed that the Fe3O4 nanoparticles could be uniformly distributed inside the PMMA, which was thought to be the underneath reason for the relatively high compressive strength of PMMA–Fe3O4.25 When the PMMA–Fe3O4 contacted with water, it could keep integrated and form a solid implant after the setting time. The setting time of PMMA was found to be significantly shorter than the reported setting time of CPC. The PMMA–Fe3O4 could transform from liquid to solid rapidly. Thus, Fe3O4 nanoparticles were quickly trapped inside the implant, which means PMMA–Fe3O4 could well confine the Fe3O4 nanoparticles inside the tumor decreasing the risk of leaking to the surrounding tissue or blood vessel. The hysteresis curve of Fe3O4 powder and PMMA-10c powder showed that both of them were ferromagnetic, while the Fe3O4 had a much higher Ms value and Oe value. As we know, the heat-generating ability of ferromagnetic materials is positively correlated to the magnitude of the coercive force of ferromagnetic materials and the alternating current magnetic field strength.30 Briefly, if the coercive force of the Fe3O4 is bigger than the applied magnetic field, then no heat would be generated by Fe3O4. The heating efficiency in vitro showed that the surface temperature and coagulation necrosis area of tissue were positively correlated to the time and the iron contents, which suggested that temperature and ablated area could be controlled to further improve its efficiency and safety. After heating, the PMMA-10c could still keep its integrity, which was different from the other reported magnetic materials which had a melting phenomenon after heating. Thus, PMMA-10c might be able to be repeated used for the magnetic ablation of residue tumor or reoccurrence tumor. Furthermore, the PMMA-10c was visible under real time ultrasound imaging which guaranteed the precisely injection of PMMA-10c inside the tumor, which further improved its efficiency for the completely ablation of tumor.
Interestingly, we found the ablated area of tumor was larger than the ablated area of the excised bovine liver using the same magnetic field at the same time. The reason might be that the tumor tissue was more sensitive to heat than the normal liver tissue.
For the bench to bed translation of this novel material, the safety issue of PMMA-10c should be concerned. The PMMA-10c was composed of PMMA and Fe3O4 nanoparticles. PMMA has been reported as a safe and biocompatible bone cement which has been used in clinic more than 50 years, especially for percutaneous vertebroplasty (PVP) and percutaneous kyphoplasty (PKP).31,32 On the other hand, Fe3O4 nanoparticles, as a magnetic resonance imaging (MRI) contrast agent, have been authorized by the FDA for clinical application.33 They are biodegradable in vivo and can be assimilated by physiological metabolism.34 Thus, we think the PMMA-10c should inherit their good safety properties. While more studies on safety, such as long-term toxicity study, need to be performed in future.
The efficiency of the injectable and phase transitional PMMA–Fe3O4 was proved in vivo in this study for the hyperthermia therapy of tumor. However, there are some limitations in this study as well, which need to be further studied. Firstly, only the surface temperature of the skin on the tumor was measured by a far-infrared thermometer, which should be lower than the temperature inside the tumor. The MR thermometry or other non-invasive methods might be useful for the measurement of the internal temperature of the tumor. Secondly, like the other minimally invasive techniques, the magnetic hyperthermia technique will also face the residual tumor problem if the tumor is too large or the magnetic materials are not homogeneously distributed inside the tumor. To combine the magnetic hyperthermia with chemotherapy might be a very good choice since the increased temperature should double the efficiency of chemotherapy to prevent the tumor from reoccurrence.35
5. Conclusion
The injectable and fast liquid–solid phase transitional PMMA–Fe3O4 was prepared and its high efficiency for tumor hyperthermia therapy was confirmed in vivo.
Acknowledgements
We acknowledge the financial supports from 973 program (No. 2014CB744500), the National Science Foundation for Distinguished Young Scholars (81425014), National Nature Science Foundation of China (No. 81227801, 81270021, 31630026, 81271598, 81571688), Chongqing Fund for Distinguished Young Scholars (cstc2013jcyjjq10004), Program for New Century Excellent Talents in University (NCET-13-1067).
References
- R. L. Siegel, K. D. Miller and A. Jemal, Ca-Cancer J. Clin., 2016, 65, 5–29 CrossRef PubMed.
- A. Jemal, F. Bray, M. M. Center, J. Ferlay, E. Ward and D. Forman, Ca-Cancer J. Clin., 2011, 61, 69–90 CrossRef PubMed.
- R. Siegel, D. Naishadham and A. Jemal, Ca-Cancer J. Clin., 2013, 63, 11–30 CrossRef PubMed.
- D. Li, J. Kang, B. J. Golas, V. W. Yeung and D. C. Madoff, Cancer Biol. Med., 2014, 11, 217–236 Search PubMed.
- R. W. Habash, R. Bansal, D. Krewski and H. T. Alhafid, Crit. Rev. Bioeng., 2006, 34, 491–542 Search PubMed.
- Y. X. Yi, Y. F. Zhang, Q. Wei, L. Zhao, J. B. Han, Y. Song, Y. Ding, G. L. Lu, J. M. Liu, H. Y. Ding, F. Dai and X. J. Tang, Chin. J. Cancer Res., 2014, 26, 112–118 Search PubMed.
- C. J. Gannon, C. R. Patra, R. Bhattacharya, P. Mukherjee and S. A. Curley, J. Nanobiotechnol., 2008, 6, 1–9 CrossRef PubMed.
- J. Van der Zee, Ann. Oncol., 2002, 13, 1173–1184 CrossRef CAS PubMed.
- S. Garca-Jimeno, R. Ortega-Palacios, M. Cepeda-Rubio, A. Vera, L. Leija and J. Estelrich, Prog. Electromagn. Res., 2012, 128, 229–248 CrossRef.
- M. Mohamed, G. Borchard and O. Jordan, J. Drug Delivery Sci. Technol., 2012, 22, 393–408 CrossRef CAS.
- M. Johannsen, U. Gneveckow, L. Eckelt, A. Feussner, N. Waldofner, R. Scholz, S. Deger, P. Wust, S. A. Loening and A. Jordan, Int. J. Hyperthermia, 2005, 21, 637–647 CrossRef CAS PubMed.
- B. Thiesen and A. Jordan, Int. J. Hyperthermia, 2008, 24, 467–474 CrossRef CAS PubMed.
- S. Laurent, S. Dutz, U. O. Hafeli and M. Mahmoudi, Adv. Colloid Interface Sci., 2011, 166, 8–23 CrossRef CAS PubMed.
- J. Rehman, J. Landman, R. D. Tucker, D. G. Bostwick, C. P. Sundaram and R. V. Clayman, J. Endourol., 2002, 16, 523–531 CrossRef PubMed.
- M. Latorre and C. Rinaldi, P. R. Health Sci. J., 2009, 28, 227–238 Search PubMed.
- M. Babic, M. Schmiedtova, R. Poledne, V. Herynek and D. Horak, J. Biomed. Mater. Res., Part B, 2015, 103, 1141–1148 CrossRef CAS PubMed.
- A. H. El-Sayed, A. A. Aly, N. I. EI-Sayed, M. M. Mekawy and A. A. EI-Gendy, J. Mater. Sci.: Mater. Med., 2007, 18, 523–528 CrossRef CAS PubMed.
- C. Y. Xu, Y. Y. Zheng, W. Gao, J. X. Xu, G. Q. Zuo, Y. Chen, M. Z. Zhao, J. B. Li, J. L. Song, N. Zhang, Z. G. Wang, H. Y. Zhao and Z. C. Mei, ACS Appl. Mater. Interfaces, 2015, 7, 13866–13875 CAS.
- M. H. Zhang, K. Sisomphon, T. S. Ng and D. J. Sun, Construct. Build. Mater., 2010, 24, 1700–1707 CrossRef.
- D. T. Felson and J. J. Anderson, Lancet, 1987, 329, 902–906 CrossRef.
- S. Nouda, S. Tomita, A. Kin, K. Kawahara and M. Kinoshita, Spine, 2009, 34, 2613–2618 CrossRef PubMed.
- M. Jager and A. Wilke, J. Biomater. Sci., Polym. Ed., 2003, 14, 1283–1298 CrossRef CAS PubMed.
- A. J. Evans, M. E. Jensen, K. E. Kip, A. J. DeNardo, G. J. Lawler, G. A. Negin, K. B. Remley, S. M. Boutin and S. A. Dunnagan, Radiology, 2003, 226, 366–372 CrossRef PubMed.
- W. F. Mousa, M. Kobayashi, S. Shinzato, M. Kamimura, M. Neo, S. Yoshihara and T. Nakamura, Biomaterials, 2000, 21, 2137–2146 CrossRef CAS PubMed.
- M. Kawashita, K. Kawamura and Z. Li, Acta Biomater., 2010, 6, 3187–3192 CrossRef CAS PubMed.
- K. Goto, J. Tamura, S. Shinzato, S. Fujibayashi, M. Hashimoto, M. Kawashita, T. Kokubo and T. Nakamura, Biomaterials, 2005, 26, 6496–6505 CrossRef CAS PubMed.
- M. Starowicz, P. Starowicz, J. Zukrowski, J. Przewoznik, A. Lemanski, C. Kapusta and J. Banas, J. Nanopart. Res., 2011, 13, 7167–7176 CrossRef CAS PubMed.
- Y. Chen, L. Jiang, R. Wang, M. Lu, Q. Zhang, Y. Zhou, Z. G. Wang, G. M. Lu, P. Liang, H. T. Ran, H. R. Chen and Y. Y. Zheng, Adv. Mater., 2014, 26, 7468–7473 CrossRef CAS PubMed.
- S. Naito, A. Eschenbach, R. Giavazzi and I. Fidler, Cancer Res., 1986, 46, 4109–4115 CAS.
- T. Atsumi, B. Jeyadevan, Y. Sato and K. Tohji, J. Magn. Magn. Mater., 2007, 310, 2841–2843 CrossRef CAS.
- K. Goto, J. Tamura, S. Shinzato, S. Fujibayashi, M. Hashimoto, M. Kawashita, T. Kokubo and T. Nakamura, Biomaterials, 2005, 26, 6496–6505 CrossRef CAS PubMed.
- P. Bourrinet, H. H. Bengele, B. Bonnemain, A. Dencausse, J. M. Idee, P. M. Jacobs and J. M. Lewis, Invest. Radiol., 2006, 41, 313–324 CrossRef CAS PubMed.
- M. Levy, N. Luciani, D. Alloyeau, V. Deveaux, C. Pechoux, S. Chat, G. Wang, N. Vats, F. Gendron, C. Factor, S. Lotersztajn, A. Luciani, C. Wilhelm and F. Gazeau, Biomaterials, 2011, 32, 3988–3999 CrossRef CAS PubMed.
- L. Lartigue, D. Alloyeau, J. Kolosnjaj-Tabi, Y. Javed, P. Guardia, A. Riedinger, C. Pechoux, T. Pellegrino, C. Wilhelm and F. Gazeau, ACS Nano, 2013, 7, 3939–3952 CrossRef CAS PubMed.
- R. D. Issels, L. H. Lindner, P. Wust, P. Reichardt, B. C. Schem, S. Abdel Rahman, S. Daugaard, C. Salat, C. M. Wendtner, Z. Vujaskovic, R. Wessalowski, K. W. Jauch, H. R. Durr, F. Ploner, A. B. Melnyk, U. Mansnann, W. Hiddemann, J. Y. Blay and P. Hohenberger, Lancet Oncol., 2010, 11, 561–570 CrossRef CAS PubMed.
|
This journal is © The Royal Society of Chemistry 2017 |