DOI:
10.1039/C7QM00210F
(Research Article)
Mater. Chem. Front., 2017,
1, 2317-2323
A glutamic acid-modified cellulose fibrous composite used for the adsorption of heavy metal ions from single and binary solutions†
Received
12th May 2017
, Accepted 10th August 2017
First published on 11th August 2017
Abstract
A new glutamic acid-modified cellulose was prepared and investigated as a bioadsorbent used for the simple and rapid removal of Cu2+ and Hg2+ from single and binary aqueous solutions. The effects of the initial concentration of heavy metal ions, pH of the solution and temperature on the adsorption capacity of the bioadsorbent were investigated. Equilibrium studies demonstrate that the adsorption of Cu2+ and Hg2+ follows the Langmuir model in a single aqueous solution system. Meanwhile, the adsorption kinetics were found to follow the pseudo-second-order model well. Competitive adsorption in the binary component system indicates the mutual inhibitation adsorption between the two metal ions. The FTIR and SEM–EDS results reveal that Cu2+ and Hg2+ are successfully adsorbed on the bioadsorbent by coordination and static interactions.
Introduction
Environmental pollution caused by heavy metal contamination is one of the worldwide problems to be concerned with in the treatment of wastewater. Pollutants can be a major threat to water resources, especially drinking water.1–6 For example, an excessive intake of heavy metal ions such as copper ions (Cu2+) can contribute to serious diseases such as Alzheimer's and haematological manifestations and thus, influence human health.7–9 Mercury ions (Hg2+) have very toxic effects even at a very low concentration.10–12 Hg(II) ions can be converted into the neurotoxin methylmercury, which is a serious environmental issue due to its bioaccumulation and magnification in aquatic food chains.13,14 Therefore, it is crucial to develop methods for the removal of heavy metal ions such as Cu2+ and Hg2+.15–18 Efforts have been made to remove heavy metal ions from wastewater, including membrane separation,19 chemical precipitation,20 electrochemical treatment21 and adsorption.22,23 Among them, adsorption is considered to be the most effective and efficient method due to its cost efficiency and flexibility in design and operation.24–26 Over the last few years, biopolymer-based adsorbents have attracted a lot of attention as one of the most promising alternatives used for the removal of Cu2+ and Hg2+, such as chitosan,27,28 lignin29 and cellulose.30–33 Cellulose is the most abundant polysaccharide and is comprised of particular groups that can be modified as a fibrous composite to improve the absorption capability towards copper ions and mercury ions.34–36 Oxidation catalyzed by TEMPO (2,2,6,6-tetramethylpiperidine-1-oxyl radical) is one of the typical methods, through which carboxyl groups are grafted to the surface of the cellulose fibers to enhance its adsorption ability.37–39 However, the adsorption efficiency is restricted and limited work has been performed in order to modify TEMPO oxidized cellulose (TOC). Conventional modifications such as in situ polymerization and grafting result in a low grafting efficiency. Recently, EDC (1-ethyl-3-(3-dimethylaminopropyl)carbodiimide), as a new catalyst, has provided a suitable approach to grafting functional groups with high efficiency.40–42
Herein, we describe a new glutamic acid-modified cellulose (GMC) fibrous composite used for copper ion and mercury ion adsorption (Scheme 1). Glutamic acid was chosen as the functional reagent due to its two carboxyl groups, which increase the binding sites for Cu2+ and Hg2+. The adsorption kinetics and isotherms as well the dual-component adsorption systems were investigated in an attempt to reveal the binding mechanism between GMC and the metal ions. The results indicated that the cellulose-based bioadsorbent could be used as a desirable adsorbent for environmental remediation.
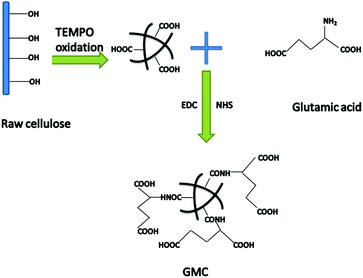 |
| Scheme 1 The synthetic route used to prepare the glutamic modified cellulose-based bioadsorbent (GMC). | |
Results and discussion
Synthesis and characterization of glutamic acid-modified cellulose (GMC)
Wood cellulose fibre was chosen as the base material. After pretreatment and TEMPO oxidation, the amine group (–NH2) of glutamic acid was reacted with the carboxyl group (–COOH) of the oxidised cellulose using the coupling agent EDC in the presence of an activation agent N-hydroxysuccinimide (NHS). In this work we used this technique to graft the oxidized cellulose suspensions with glutamic acid to introduce additional binding sites and the resulting composite material was characterized by SEM (Fig. 1). In addition, the degree of TEMPO oxidisation in cellulose was evaluated using an electric conductivity titration method (Fig. S1, ESI†). The COOH content was calculated to be 1.2 mmol g−1.
 |
| Fig. 1 SEM images of (a) TEMPO oxidised cellulose, (b) glutamic modified cellulose, (c) Cu(II)-loaded GMC and (d) Hg(II)-loaded GMC. | |
The broad peaks at around 3398 and 2902 cm−1 in the FTIR spectra are very common due to the O–H stretching vibrations and the stretching vibrations of aliphatic C–H (Fig. S2, ESI†), respectively. The peaks at 1164 and 1058 cm−1 are related to the saccharide structure and can be assigned to the C–O stretching vibrations. One carbonyl peak at 1739 cm−1 in the TEMPO-oxidized cellulose (TOC) suggests the successful oxidation modification. In the glutamic acid-modified cellulose, the CONH carbonyl group is observed between the two peaks but not clearly resolvable. These findings suggest the successful grafting of glutamic acid onto cellulose. Fig. 1 shows the typical SEM images of the four-types of cellulose fibres. It was observed that the cellulose fibers were loose and fibrillated after chemical treatment. Fig. 1b indicates that the oxidation reaction breaks up the cellulose wood pulp into smaller fibres. In addition, the fibres become even smaller after the glutamic acid-modification step. The coupling of glutamic acid induced by EDC/NHS was further confirmed by the presence of a high N content of 9.49 wt%, as shown in the EDS spectrum of GMC (Fig. 2a). Furthermore, when compared to unmodified cellulose, the adsorption capacity of GMC for Cu2+ and Hg2+ increased 20 times and 8 times, respectively, which also indicates the successful modification of cellulose (Fig. S3, ESI†).
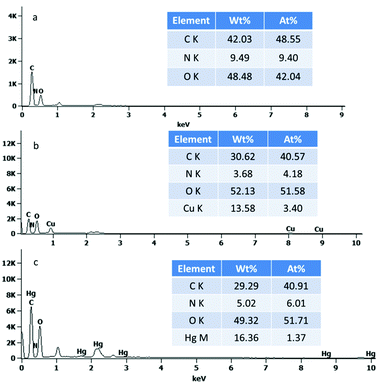 |
| Fig. 2 The EDS of (a) GMC, (b) Cu2+-loaded GMC and (c) Hg2+-loaded GMC. | |
Adsorption parameter effects on the adsorption of Cu2+ and Hg2+ ions
To take insight into the glutamic acid-modified cellulose (GMC) materials, the effects of different adsorption parameters on its performance were investigated. First, the effect of pH on the adsorption of Cu2+ and Hg2+ was studied over a pH range from 1.0 to 6.0. As seen in Fig. 3, the adsorption was seen to increase with an increase in pH and reaches a plateau at pH 5.0. At lower pH, protonation of the active sites on the adsorbent surface takes place, resulting in the electrostatic repulsions between the metal cations and the protonated groups, which prevents the adsorption of the metal ions, hence resulting in a lower Cu2+ ion uptake. At higher pH, the free carboxyl groups are available on the adsorbent for ion adsorption. Therefore, the Q values increase with pH,43 but the precipitation of Cu as Cu(OH)2 and Hg as Hg(OH)2 takes place at pH ≥ 6.44 Thus, pH 5.0 was selected as the optimum pH for our further studies.45
 |
| Fig. 3 The effect of pH on the adsorption of Cu2+ and Hg2+. Conditions: pH 1–6, GMC dosage was 2.0 g L−1, T = 25 °C and t = 30 min; [Cu2+] = [Hg2+] = 50 mg L−1. | |
Subsequently, the relationship between temperature and the adsorption capacity was investigated. As seen in Fig. 4, temperature variation had a positive effect. The adsorption capacity of Cu2+ and Hg2+ increased upon increasing the temperature from 25 to 35 °C and an equilibrium was reached with a further increase in temperature. With a temperature increase, the polymer structure opens and more active sites become accessible to the metal ions. Therefore, the adsorption capacity was observed to increase.34
 |
| Fig. 4 The effects of temperature on the adsorption of Cu2+ and Hg2+. Conditions: pH = 5, GMC dosage was 2.0 g L−1, and t = 30 min; [Cu2+] = [Hg2+] = 100 mg L−1. | |
The kinetics of single metal solutions
The adsorption was investigated as a function of time from 0 to 120 min in batch experiments with 50 mg L−1 Cu2+ and 50 mg L−1 Hg2+, respectively at pH 5.0 and 25 °C to collect data for the kinetic studies. The adsorption capacity for both Cu2+ and Hg2+ was observed to increase with time and an equilibrium for Cu2+ adsorption was attained after 20 min with a maximum adsorption capacity of 23.1 mg g−1, while an equilibrium for Hg2+ adsorption was reached after 10 min with a maximum adsorption capacity 22.9 mg g−1 (Fig. 5). Based on the above results, three adsorption kinetics models including the pseudo-first-order kinetic model (Fig. S4, S5 and eqn (S1), ESI†), the pseudo-second-order kinetic model (Fig. S6, S7 and eqn (S2), ESI†) and intraparticle diffusion kinetic model (Fig. S8 and eqn (S3), ESI†) were exploited. The linear fitting results of the two models are displayed and the kinetic model parameters calculated from the slope and intercept of each linear plot are summarized in Table 1. The correlation coefficients (R2) for the pseudo-second-order kinetic model are higher than those for the pseudo-first-order kinetic model and the Qe values calculated from the pseudo-second-order kinetic model are very close to the experimental ones, demonstrating that the pseudo-second order kinetic model matches the kinetic model of GMC. In general, the pseudo-first-order and the pseudo-second-order models hint the physical adsorption and chemical adsorption dominate, respectively. These results suggest that the overall rates of the adsorption of GMC are controlled by chemical adsorption.
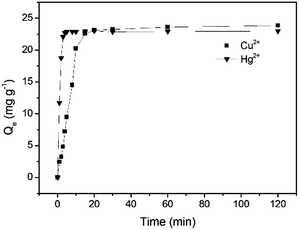 |
| Fig. 5 Cu2+ and Hg2+ adsorption on GMC with respect to time at pH 5.0, 25 °C and [Cu2+] = [Hg2+] = 50 mg L−1. | |
Table 1 The kinetic parameters for Cu2+ and Hg2+ absorption under different time durations
Parameter |
Pseduo-first-order model |
C
0/metal (mg g−1) |
Q
e (exp) (mg g−1) |
Q
e (cal) (mg g−1) |
k
1 (min−1) |
R
2
|
h
1 (mg g−1 min−1) |
Cu2+ 50 |
23.86 |
10.969 |
0.047 |
0.728 |
0.516 |
Hg2+ 50 |
23.15 |
1.587 |
0.017 |
0.098 |
0.027 |
Parameter |
Pseduo-second-order model |
C
0/metal (mg g−1) |
Q
e (exp) (mg g−1) |
Q
e (cal) (mg g−1) |
k
2 × 104 (mg g−1 min−1) |
R
2
|
h
2
|
Cu2+ 50 |
23.86 |
25.61 |
59.129 |
0.981 |
3.875 |
Hg2+ 50 |
23.15 |
23.02 |
2194.274 |
0.999 |
116.28 |
The intraparticle diffusion kinetic model was widely applied to study the process to predict the rate-controlling step. The curve fitting plots of the intraparticle diffusion model are displayed in Fig. S8 (ESI†). The intraparticle diffusion plots contained two regions: the sharper stage and the second stage. The sharper stage indicated the external surface adsorption or instantaneous adsorption due to intraparticle diffusion. In the second stage, the intraparticle diffusion rate decreased due to the reduction of the functional groups bearing negative charges on the surface and the reduction of the ion concentration in solution. However, the intraparticle diffusion rate constant (kd1) for the first sharper portion was higher than that (kd2) for the second portion and C1 was smaller than C2 (Table S1, ESI†), which indicates the rate of Cu2+ and Hg2+ removal were higher than at the beginning. None of plots pass through the origin, which indicates intraparticle diffusion was not the only rate-controlling step in the adsorption process.
Adsorption isotherms
The adsorption capacity (Qe) and the equilibrium concentration of Cu2+ and Hg2+ have a certain mathematical relationship (Fig. 6), which can be presented by the isothermal adsorption model, respectively. It was observed that the amount of metal ions captured by GMC increased with an increase in the concentration and ultimately reached a saturated value. In order to analyse the distribution of Cu2+ and Hg2+ in the adsorbent and solution, the Langmuir model (Fig. S9, S10 and eqn (S4), ESI†) and Freundlich model (Fig. S11, S12 and eqn (S5), ESI†) were employed. The Langmuir isotherm model and Freundlich isotherm model curves obtained for Cu2+ and Hg2+ adsorption on GMC are shown in Fig. S8–S11 (ESI†). In addition, the results were summarized in Table 2. The correlation coefficients of the Langmuir isotherm model are higher than that of the Freundlich isotherm model, indicating that the Langmuir isotherm model matches the isothermal adsorption process of GMC and the absorption of GMC was a monolayer molecule adsorption process. The RL values of GMC for both metal ions are considerably smaller than 1.0, indicating the favorable adsorption for both metal ions under the investigated conditions.
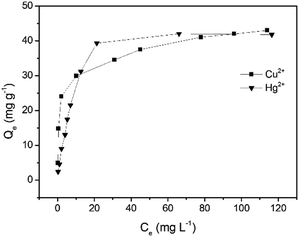 |
| Fig. 6 The Langmuir adsorption isotherms obtained for the monocomponent adsorption of Cu2+ and Hg2+ on GMC at pH = 5, 2.0 g L−1 GMC at 30 min. | |
Table 2 The Langmuir and Freundlich parameters obtained for Cu2+ and Hg2+ adsorption
Langmuir |
Q
m (mg g−1) |
K
L × 103 (L mg−1) |
R
L
|
R
2
|
RMSE |
Cu2+ |
43.309 |
285.909 |
0.017–0.412 |
0.996 |
4.736 |
Hg2+ |
44.883 |
147.694 |
0.033–0.576 |
0.996 |
2.404 |
Freundlich |
K
F ((mg g−1) (mg L−1)−1/n) |
n
|
R
2
|
RMSE |
Cu2+ |
13.450 |
3.68 |
0.831 |
4.380 |
Hg2+ |
6.288 |
2.011 |
0.910 |
9.656 |
In order to investigate the competitive adsorption, a dual-component adsorption system comprised of Cu2+–Hg2+ was studied. As seen in Fig. 7, at a fixed concentration of Cu2+ or Hg2+, the adsorption capacity for Hg2+ or Cu2+ decreased. Qmix/Q0 was employed to understand the competitive adsorption process, where Qmix is the adsorption capacity in the mixed solution and Q0 is the adsorption capacity in a single metal ion solution.46 The value of Qmix/Q0 was less than 1, indicating that the two metal ions have mutual inhabitation, which can be attributed to the radius, electronegativity and softness of the metal ions in the complex adsorption process.
 |
| Fig. 7 (a) The adsorption isotherms obtained for Cu2+ in single and binary (Cu2+ + 50 mg L−1 Hg2+) systems. (b) The adsorption isotherms obtained for Hg2+ (Hg2+ + 50 mg L−1 Cu2+) in single and binary systems. | |
Desorption and recycling experiments
The recyclability of GMC and the adsorption rate of metal ions are important in environmental protection (Fig. 8). The regeneration of GMC was performed by immersing the adsorbent into HNO3 solution for 100 min. After five desorption cycles, the recyclable adsorption behavior of GMC towards Hg2+ and Cu2+ was similar and the adsorption rates were only slightly decreased. The removal efficiency of GMC towards Cu2+ after one and five cycles was 95.1% and 88.0%, respectively, whilst 85.1% and 80.2% towards Hg2+ under the same conditions. The excellent reusability indicates that GMC could be a potential absorbent in practical applications.
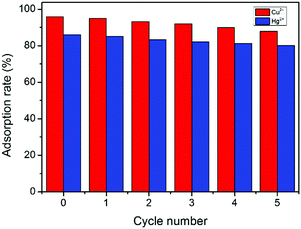 |
| Fig. 8 The adsoption rate (%) of metal ions by the GMC bioadsorbent at different regeneration cycles. Conditions: pH 1–6, GMC dosage = 2.0 g L−1, T = 25 °C and t = 30 min; [Cu2+] = [Hg2+] =50 mg L−1. | |
Adsorption mechanism
Evidence to support the adsorption of Cu2+ and Hg2+ were attained from the FTIR spectra of the Cu2+-loaded GMC and Hg2+-loaded GMC. The adsorption of Cu2+ and Hg2+ on the bioadsorbent was indicated by the shifting of the position of some basic peaks and the presence of additional peaks in the FTIR spectra (Fig. S2, ESI†). An obvious change in the FTIR spectra was found at the peak at 1385 cm−1, which disappeared after Cu2+ and Hg2+ adsorption. Therefore, metal ions adsorption on the adsorbent affected the chemical bonds with the N atoms. The intensity of the C
O in the band of 1733 and 1621 cm−1 was attenuated and its shape was shifted due to the carboxylic acid combining with the heavy metal ions, which suggested GMC was influenced by ion-exchange. It can be proposed that the bioadsorbent adsorption action was controlled by physical and chemical adsorption. Therefore, the main adsorption mechanism associated with the adsorption behavior was via coordination and electrostatic interactions. Similarly, the SEM images of the Cu(II)-loaded GMC (Fig. 1c) and Hg(II)-loaded GMC (Fig. 1d) have different surface morphologies from the precursor GMC (Fig. 1b). Furthermore, the EDS results of the Cu(II)-loaded GMC and Hg(II)-loaded GMC indicated the presence of peaks corresponding to Cu in the spectrum (Fig. 2b) and Hg in the spectrum (Fig. 2c), supporting the adsorption of Cu2+ and Hg2+ onto the adsorbent.
Conclusions
In conclusion, we have developed a new glutamic acid modified cellulose fibrous composite for Cu2+ and Hg2+ adsorption. Both the adsorption isothermal experiments and kinetic studies were employed to understand the adsorption process. Best fitting using a Langmuir isotherm model indicates that the absorption of Cu2+ and Hg2+ was a monolayer molecule adsorption process. The adsorption reaction follows a pseudo-second-order model, indicating the overall rates of adsorption on GMC are controlled by chemical adsorption. The competitive adsorption in a binary component system indicates the mutual inhabitation adsorption between the two metal ions. Since our GMC based system is simply prepared with excellent adsorption capacity, we believe that it will become the adsorbent of choice for industrial applications. For future industrial applications, the economic effects are very important. Therefore, cheap modifying agents need to be further evaluated. Also, the potential of cellulose-based adsorbents in practical applications will further be investigated in a continuous flow fixed-bed column in our future work.
Experimental
Materials
All chemical reagents and solvents were analytical grade and purchased from commercial suppliers. Pulp wood was purchased from Sinopharm. Mercury acetate, copper sulfate pentahydrate, NaBr, Na2CO3, NaHCO3, NaOH, 1-ethyl-3-(3-dimethylaminopropyl) carbodiimide hydrochloride (EDC), N-hydroxysuccinimide (NHS) and 2,2,6,6,-tetramethyl-1-(pyperidinyloxy) radical (TEMPO) were purchased from Aladdin.
Preparation of the GMC fibre composites
Pulp wood (20 g) was first added to an aqueous solution of sodium hydroxide (500 mL, 15% w/v) at 60 °C. The resulting mixture was stirred for 2 h, washed thoroughly with deionized water and neutralized with HCl. A mixture of the pretreated cellulose (5 g), TEMPO (0.08 g), NaBr (0.5 g) and Na2CO3/NaHCO3 buffer (pH = 10.8) were stirred under magnetic stirring at 600 rpm and then, an aqueous solution of sodium hydroxide (0.5 mol L−1) was added to keep the pH at 10–11. Ethanol was added to keep the pH constant.
The resulting mixture was centrifuged (10
000 rpm, 20 min) and washed with deionized water and ethanol. The precipitate was dried in a vacuum oven (40 °C) for 24 h to give the TEMPO oxidized cellulose (TOC1). The TOC1 was added to an aqueous solution of sodium chlorite (200 mL, 15% w/v, pH = 4.8) and oxidized, and then centrifuged, washed and dried to afford TOC2. TOC2 (2 g) was dissolved in water (400 mL), then EDC and NHS (1.1–1.5 eq. to carboxyl groups of microcrystalline cellulose) were added to the mixture. The pH of the resulting mixture was adjusted to 7.5–8 with an aqueous solution of NaOH and HCl and the reaction was kept at r.t. until deionized water was added to remove redundant EDC/NHS and side products. Glutamic acid (2 equiv. of COOH on TOC2) was added and reacted with the mixture at r.t. for 24 h. When the reaction was completed, the redundant glycine was washed off. The products were centrifuged and freeze-dried.
Characterization of the glutamic modified cellulose (GMC)
FTIR spectra were recorded on the Nicolet iS-50 spectrometer at room temperature. SEM images were recorded using a JEOL JSM-7500F. Electric conductivity titration was recorded on a Shanghai Shengci DDS-IIA conductivity meter.
The adsorption of metal ions
For the adsorption experiments, copper sulfate pentahydrate and mercury acetate were used. 50 mg of GMC was added into 25 mL of the heavy metal ion solution for 30 min except for the study of contact time. The amount of residual copper ions in the solution was traced using a Shimadzu AA-680 atomic absorption spectrophotometer and the amount of residual mercury ions in the solution was traced using a Beijing Titan AFS-933 atomic fluorescence spectrometry.
The effect of contact time (0 to 120 min), pH (1 to 6) and temperature (25 to 65 °C) were also investigated with 2 g L−1 of dry GMC and the initial concentration of metals was 50, 50 and 100 mg L−1, respectively. The adsorbed amounts of the heavy metal ions (qt, mg g−1) were determined according to eqn (1):
|  | (1) |
where
C0 is the initial solution concentration (mg L
−1) and
Ct is the solution concentration at time
t,
V is the volume of solution (L) and
W is the weight of dry GMC (g).
Recyclability
The recyclability of GMC was recorded at 25 °C. 50 mg of GMC was added into 25 mL of 50 mg L−1 Cu2+ and Hg2+ metal ion solutions for 30 min, respectively. Then, the GMC was removed from the solutions. The desorption and regeneration of the GMC adsorbed with metal ions were performed by immersing the GMC into 100 mL of 1.0 mol L−1 HNO3 solution under agitation at 25 °C for 100 min. The regenerated GMC was used for another adsorption in the subsequent cycles.
Conflicts of interest
There are no conflicts to declare.
Acknowledgements
This work was supported by the National key Research and Development Program (No. 2016YFA0200300), NSFC for Distinguished Young Scholars (21325625), NSFC (51379077, 21607044), the Fundamental Research Funds for the Central Universities (2016MS108) and Natural Science Foundation of Hebei Province (B2017502069).
Notes and references
- Y. Yu, J. G. Shapter, R. Popelka-Filcoff, J. W. Bennett and A. V. Ellis, J. Hazard. Mater., 2014, 273, 174–182 CrossRef CAS PubMed.
- I. E. M. Carpio, J. D. Mangadlao, H. N. Nguyen, R. C. Advincula and D. F. Rodrigues, Carbon, 2014, 77, 289–301 CrossRef.
- X. Xin, G. Huang, X. Liu, C. An, Y. Yao, H. Weger, P. Zhang and X. Chen, Environ. Pollut., 2017, 226, 12–20 CrossRef CAS PubMed.
- X. Zeng, Y. Zhu, C. Chen, Y. Tong, Y. Li, G. Huang, S. Nie and X. Wang, J. Cleaner Prod., 2017, 154, 61–82 CrossRef.
- Y. Pi, B. Chen, M. Bao, F. Fan, Q. Cai, L. Ze and B. Zhang, Bioresour. Technol., 2017, 232, 263–269 CrossRef CAS PubMed.
- L. L. Deng, C. X. Zhao, Y. Ma, S. S. Chen and G. Xu, Anal. Methods, 2013, 5, 3709–3713 RSC.
- S. G. Kaler, Nat. Rev. Neurol., 2011, 7, 15–29 CrossRef CAS PubMed.
- Q. Zou, X. Li, J. Zhang, J. Zhou, B. Sun and H. Tian, Chem. Commun., 2012, 48, 2095–2097 RSC.
- M. Li, H. Ge, R. L. Arrowsmith, V. Mirabello, S. W. Botchway, W. Zhu, S. I. Pascu and T. D. James, Chem. Commun., 2014, 50, 11806–11809 RSC.
- P. Holmes, K. James and L. Levy, Sci. Total Environ., 2009, 408, 171–182 CrossRef CAS PubMed.
- Y. Gong, Y. Liu, Z. Xiong and D. Zhao, Environ. Sci. Technol., 2014, 48, 3986–3994 CrossRef CAS PubMed.
- K. H. Nam, S. Gomez-Salazar and L. L. Tavlarides, Ind. Eng. Chem. Res., 2003, 42, 1955–1964 CrossRef CAS.
- L. I. Sweet and J. T. Zelikoff, J. Toxicol. Environ. Health, Part B, 2001, 4, 161–205 CAS.
- N. Saman, K. Johari, S. S. Tien and H. Mat, Clean: Soil, Air, Water, 2014, 42, 1541–1548 CrossRef CAS.
- V. Gupta, P. Carrott, R. Singh, M. Chaudhary and S. Kushwaha, Bioresour. Technol., 2016, 216, 1066–1076 CrossRef PubMed.
- R. Li, L. Liu and F. Yang, J. Hazard. Mater., 2014, 280, 20–30 CrossRef CAS PubMed.
- J. Tang, H. Lv, Y. Gong and Y. Huang, Bioresour. Technol., 2015, 196, 355–363 CrossRef CAS PubMed.
- S. Hokkanen, A. Bhatnagar and M. Sillanpää, Water Res., 2016, 91, 156–173 CrossRef CAS PubMed.
- F. Fu and Q. Wang, J. Environ. Manage., 2011, 92, 407–418 CrossRef CAS PubMed.
- N. Meunier, P. Drogui, C. Montané, R. Hausler, G. Mercier and J.-F. Blais, J. Hazard. Mater., 2006, 137, 581–590 CrossRef CAS PubMed.
- S. Y. Lim, W. Shen and Z. Gao, Chem. Soc. Rev., 2015, 44, 362–381 RSC.
- S. Kumari and G. S. Chauhan, ACS Appl. Mater. Interfaces, 2014, 6, 5908–5917 CAS.
- Y. Pan, P. Cai, M. Farmahini-Farahani, Y. Li, X. Hou and H. Xiao, Appl. Surf. Sci., 2016, 385, 333–340 CrossRef CAS.
- Y. Zhang, Y. Liu, X. Wang, Z. Sun, J. Ma, T. Wu, F. Xing and J. Gao, Carbohydr. Polym., 2014, 101, 392–400 CrossRef CAS PubMed.
- R. Sitko, E. Turek, B. Zawisza, E. Malicka, E. Talik, J. Heimann, A. Gagor, B. Feist and R. Wrzalik, Dalton Trans., 2013, 42, 5682–5689 RSC.
- M.-W. Wan, C.-C. Kan, B. D. Rogel and M. L. P. Dalida, Carbohydr. Polym., 2010, 80, 891–899 CrossRef CAS.
- S. R. Popuri, Y. Vijaya, V. M. Boddu and K. Abburi, Bioresour. Technol., 2009, 100, 194–199 CrossRef CAS PubMed.
- X. Liang, J. Duan, Q. Xu, X. Wei, A. Lu and L. Zhang, Chem. Eng. J., 2017, 317, 766–776 CrossRef CAS.
- A. Kriaa, N. Hamdi and E. Srasra, Desalination, 2010, 250, 179–187 CrossRef CAS.
- Q.-Q. Zhong, Q.-Y. Yue, Q. Li, B.-Y. Gao and X. Xu, Carbohydr. Polym., 2014, 111, 788–796 CrossRef CAS PubMed.
- H. Chen, G. Dai, J. Zhao, A. Zhong, J. Wu and H. Yan, J. Hazard. Mater., 2010, 177, 228–236 CrossRef CAS PubMed.
- Y. Li, H. Xiao, M. Chen, Z. Song and Y. Zhao, J. Mater. Sci., 2014, 49, 6696–6704 CrossRef CAS.
- Z. Jiang, Y. Fang, Y. Ma, M. Liu, R. Liu, H. Guo, A. Lu and L. Zhang, J. Phys. Chem. B, 2017, 121, 1793–1801 CrossRef CAS PubMed.
- K. Chauhan, G. S. Chauhan and J.-H. Ahn, Bioresour. Technol., 2009, 100, 3599–3603 CrossRef CAS PubMed.
- R. Batmaz, N. Mohammed, M. Zaman, G. Minhas, R. M. Berry and K. C. Tam, Cellulose, 2014, 21, 1655–1665 CrossRef CAS.
- Y. Tian, M. Wu, R. Liu, Y. Li, D. Wang, J. Tan, R. Wu and Y. Huang, Carbohydr. Polym., 2011, 83, 743–748 CrossRef CAS.
- A. Isogai, T. Saito and H. Fukuzumi, Nanoscale, 2011, 3, 71–85 RSC.
- T. Saito, S. Kimura, Y. Nishiyama and A. Isogai, Biomacromolecules, 2007, 8, 2485–2491 CrossRef CAS PubMed.
- R. Hiraoki, Y. Ono, T. Saito and A. Isogai, Biomacromolecules, 2015, 16, 675–681 CrossRef CAS PubMed.
- S. Barazzouk and C. Daneault, Cellulose, 2011, 18, 643–653 CrossRef CAS.
- R. Yang, K. B. Aubrecht, H. Ma, R. Wang, R. B. Grubbs, B. S. Hsiao and B. Chu, Polymer, 2014, 55, 1167–1176 CrossRef CAS.
- C. Wang, Q. Yan, H.-B. Liu, X.-H. Zhou and S.-J. Xiao, Langmuir, 2011, 27, 12058–12068 CrossRef CAS PubMed.
- R. Ansari and F. Raofie, J. Chem., 2006, 3, 35–43 CAS.
- S. Deng, R. Bai and J. P. Chen, Langmuir, 2003, 19, 5058–5064 CrossRef CAS.
- G. Z. Kyzas and E. A. Deliyanni, Molecules, 2013, 18, 6193–6214 CrossRef CAS PubMed.
- Y. Zhu, J. Hu and J. Wang, J. Hazard. Mater., 2012, 221, 155–161 CrossRef PubMed.
Footnote |
† Electronic supplementary information (ESI) available. See DOI: 10.1039/c7qm00210f |
|
This journal is © the Partner Organisations 2017 |