DOI:
10.1039/C7QM00107J
(Research Article)
Mater. Chem. Front., 2017,
1, 1823-1828
A tetraphenylethylene (TPE)-based supra-amphiphilic organoplatinum(II) metallacycle and its self-assembly behaviour†
Received
8th March 2017
, Accepted 9th May 2017
First published on 9th May 2017
Abstract
In recent years, fluorescent functional materials with aggregation-induced emission (AIE) properties have evolved to be one of the most attractive topics within chemistry and materials science. In particular, discrete TPE-based metallacycles or metallacages through coordination-driven self-assembly have proven to be novel scaffolds to construct various fluorescent materials with light-emitting characteristics. Herein, we report the preparation of a new family of discrete, TPE-based supra-amphiphilic metallacycles decorated with three PNIPAAM arms through combination of an exo-functionalization strategy and post-assembly polymerization. The obtained supra-amphiphilic organometallic species could spontaneously self-assemble into fluorescent nanoparticles in water. Moreover, by taking advantages of both AIE property and good biocompatibility of the obtained TPE-based supra-amphiphilic metallosupramolecular structure, its potential application in cell imaging was investigated.
Introduction
During the past few decades, coordination-driven self-assembly based on the dynamic metal–ligand interactions has emerged as a highly efficient strategy to build discrete metallosupramolecular architectures with well-defined shapes and sizes such as two-dimensional (2-D) polygons and three-dimensional (3-D) polyhedra.1 To date, a large number of delicate and elegant metallosupramolecular architectures have been successfully prepared, some of which have found wide applications in materials science and biotechnology.2 However, many discrete, well-defined metallosupramolecular structures to date have been built from rigid yet fairly inert building blocks, thus limiting their further applications. With the aim of constructing artificial functional assemblies through coordination-driven self-assembly, a great deal of effort has been devoted to the synthesis of functionalized building blocks (donors and acceptors) through rational design.3 Thus the introduction of a functional moiety into the precursors has evolved to be a facile yet efficient approach to the preparation of functionalized organometallic assemblies.4
Tetraphenylethylene (TPE) is an archetypical fluorogen with intriguing aggregation induced emission (AIE) properties.5 Such an AIE phenomenon is related to the restrictions of phenyl-ring rotation and the ethylentic C
C bond twist. Since Tang's pioneer work in the field of AIE,6 TPE has been widely used as the basis of chemical sensors, biological probes, etc.7 Recently, AIE-active molecules have been anchored onto discrete metallosupramolecular structures to construct light-emitting materials, which have presented intriguing optical properties.8 For example, Stang and co-workers reported the first TPE-based discrete metallacages, which featured a highly emissive behaviour in dilute solution.8a Very recently, we prepared a new family of tris-TPE metallacycles via coordination-driven self-assembly, which was able to aggregate into ordered nano-structures upon addition of linear heparin driven by multiple electrostatic interactions, thus allowing for the selective detection of heparin at the clinical dosage level.8b However, the most well-known TPE-based metallacycles or metallacages are soluble in organic solvents, which limits their further applications as biomaterials. Thus the development of TPE-based metallosupramolecular species with biocompatibility is indeed desirable.
Supra-amphiphiles that are formed on the basis of noncovalent interactions have gained much attention in recent years because of their diverse self-assembly behaviour in water and potential applications as biomaterials.9 Stimulated by the successful examples of supra-amphiphiles in the literature, herein, we present a facile approach to construct TPE-based supra-amphiphilic metallacycles with good biocompatibility. A combination of 120° TPE functionalized dipyridyl donors with 120° di-Pt(II) acceptors decorated with chain transfer agents (CTAs) resulted in the formation of a hexagonal organoplatinum(II) metallacycle driven by coordination interactions (Fig. 1). This exo-functionalization strategy allows good control over the number and position of TPE and CTA moieties on the periphery of the supramolecular metallacycle. Subsequently, post-assembly controlled radical polymerization of (N-isopropylacrylamide) (NIPAAM) by using the obtained metallacycle as an RAFT agent produced a new family of supra-amphiphilic metallacycles decorated with three polymeric arms. Due to the introduction of the TPE moiety, the metallacycle exhibited typical AIE characteristics. Moreover, benefiting from the amphiphilic properties, the obtained supra-amphiphilic star polymer could spontaneously self-assemble into ordered, fluorescent nanoparticles in water. The good biocompatibility of the obtained TPE-based supra-amphiphilic metallacycle allowed for investigation on its biological application in cell imaging.
 |
| Fig. 1 Schematic and molecular structure of 120° TPE containing the dipyridyl donor 1 and the 120° CTA-based di-Pt(II) acceptor 2. | |
Results and discussion
According to the “directional bonding” model and the “symmetry interaction” principle,1a the combination of three 120° dipyridyl donors with three 120° di-Pt(II) acceptors generates a new family of hexagons (Scheme 1).10 The 120° TPE containing dipyridyl donor 1 was prepared according to the procedure reported in previous literature work.8g The 120° CTA-based di-Pt(II) acceptor 2 was easily synthesized through an esterification reaction as shown in Schemes S1 and S2 (ESI†). Stirring the mixture of the building blocks of 1 and 2 in a stoichiometric ratio in dichloromethane at room temperature for 5 h resulted in the formation of [3+3] hexagon 3 containing TPE and CTA functional groups located at the alternative vertex of the metallacycle. The counter anion of 3 was exchanged from NO3− to PF6− by addition of a saturated aqueous solution of KPF6. Multinuclear NMR (1H and 31P) analysis of the reaction mixture revealed the formation of a hexagonal metallacycle with double functionalities (TPE and CTAs). For instance, the 31P{1H} NMR spectrum of metallacycle 3 displayed a sharp singlet at 16.70 ppm, which shifted upfield from that of the starting 120° di-Pt(II) acceptor 2 by approximately 4.78 ppm. This change, as well as the decrease in coupling of flanking 195Pt satellites ∼ΔJ ≈ −370.4 Hz for 3, is consistent with the electron back-donation from the platinum atom, providing further support to the formation of the supramolecular hexagon (Fig. S1, ESI†). In addition, in the 1H NMR spectrum of 3, the pyridine protons exhibited downfield shifts (α-HPy, 0.33 ppm; β-HPy, 0.45 ppm) resulting from the loss of electron density upon coordination of the pyridine N atom with the Pt(II) metal center. Analysis of electrospray ionization time of flight mass spectrometry (ESI-TOF-MS) provided further evidence for the formation of hexagon 3. The ESI-TOF-MS spectrum of 3 revealed three peaks at m/z = 1449.74, 1130.60, and 918.17, corresponding to [M − 4PF6−]4+, [M − 5PF6−]5+, and [M − 6PF6−]6+ species, respectively. All of these peaks were isotopically resolved, and they were in good agreement with their theoretical distributions (Fig. 2), which allowed for metallacycle 3 to be unambiguously established.
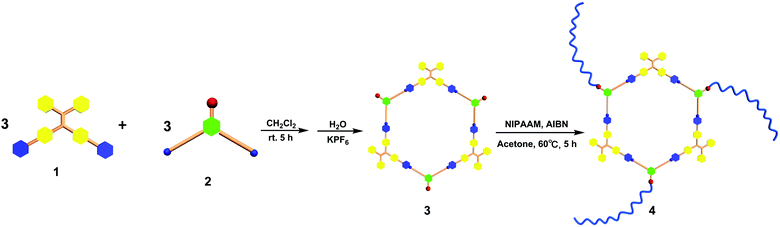 |
| Scheme 1 Graphical representation of the synthesis of TPE-based organoplatinum(II) metallacycle 3 and star supramolecular polymer 4. | |
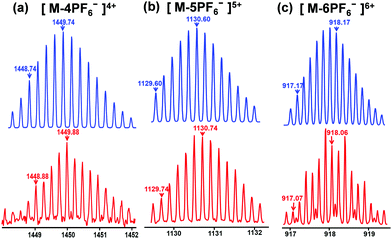 |
| Fig. 2 Calculated (top) and experimental (bottom) ESI-TOF-MS of metallacycle 3. | |
With the newly designed metallacycle 3 containing tris-CTAs and TPE groups in hand, subsequent post-assembly polymerization was carried out as shown in Scheme 1. RAFT polymerization of N-isopropylacrylamide (NIPAAM) with metallacycle 3 was performed in acetone in the presence of 2,2′-azobis (isobutyronitrile) (AIBN) as an initiator. After polymerization, polymer 4 was isolated by precipitation in diethyl ether, collected by filtration, and dried under vacuum. Multinuclear NMR (1H and 31P) spectroscopy and gel permeation chromatography (GPC) were employed to characterize the resultant polymer. As shown in Fig. S1 (ESI†), in the 1H NMR spectrum, the characteristics of the signals at δ = 4.02, 1.61–1.81, and 1.15 ppm are ascribed to the methine proton in the isopropyl group, the methylene protons, and the protons of the methyl groups of PNIPAAM, respectively. Notably, the signals corresponding to the pyridyl moieties remained almost unchanged, which indicated that the controlled RAFT polymerization did not destroy the metallacyclic scaffold. Moreover, the 31P{1H} NMR spectrum of polymer 4 displayed a singlet at 17.08 ppm, which was consistent with that of the original metallacycle, again demonstrating the retention of the metallacycle in the obtained star supramolecular polymer 4. From the FT-IR spectrum of the obtained polymer 4, the amide carbonyl stretching band and N–H bending vibration at 1640.21 and 1544.93 cm−1 were clearly observed, respectively. The GPC analysis indicated that the molecular weight (Mn) of polymer 4 was 12 kDa with a polydispersity index (PDI) of 1.20. It should be noted that the molecular weight of metallacycle 3 was found to be around 0.7 kDa obtained from the GPC investigation (Fig. S2, ESI†), which was much smaller than that of the star polymer. Thus, the GPC investigation indicated that the RAFT polymerization of NIPAAM by employing tris-CTA metallacycle as the RAFT agent allowed for the formation of star supramolecular polymer 4 containing a discrete metallacycle.
Because of the existence of the TPE moiety in star polymer 4 in hand, the AIE property of polymer 4 was then evaluated in a mixed THF/water solvent system. As shown in Fig. 3a, polymer 4 was non-emissive when it was dissolved in THF. With the addition of water, the emission of polymer 4 in the mixed solvents remained as faint as it had been in the original THF solution when the water fraction was below 70%. However, upon increasing the water content to 100%, marked emission enhancement was observed at 485 nm (Fig. 3a). Notably, with the increase in the water content from 0 to 100%, the emission intensity value increased approximately 30-fold compared to the original state (Fig. 3b). The fluorescence quantum efficiency of star polymer 4 was determined to be 3.2% in the aggregated state. As shown in Fig. S3 (ESI†), an obvious enhancement of the emission at 365 nm under an ultraviolet lamp was observed. These findings revealed that the star polymer 4 exhibits the typical AIE characteristics due to the introduction of the TPE group.
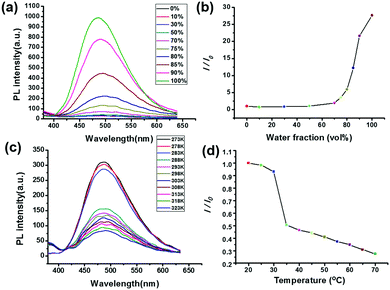 |
| Fig. 3 Fluorescence spectra of 4 in different water fractions with a concentration of 1.0 mg mL−1 (a), and relative fluorescence intensity (I/I0) of 4 in the THF/H2O system at 485 nm (b). PL spectra of an aqueous solution of 4 with an increase in temperature from 20 to 70 °C (c), and relative fluorescence intensity of 4 in water at different temperatures at 485 nm (d). | |
Benefiting from the amphiphilic property, polymer 4 can spontaneously self-assemble into ordered nanoparticles in water due to the existence of the hydrophobic TPE-based metallacycle aggregated in the core and hydrophilic PNIPAAM extended into the water at room temperature. The critical aggregation concentration (CAC) is approximately 0.008 mg mL−1 as monitored by the fluorescent probe method (Fig. S4, ESI†). The self-assembly behaviour of star polymer 4 was investigated by employing dynamic light scattering (DLS), transmission electron microscopy (TEM) and atomic force microscopy (AFM). With a concentration of 1.0 mg mL−1, the dynamic light scattering study displayed that the averaged hydrodynamic size of these nanoparticles was maintained at ca. 30.0 nm (Fig. 4a). Subsequently, an aqueous solution of polymer 4 with a concentration of 1.0 mg mL−1 was deposited on copper grids, followed by evaporation in air at room temperature and TEM investigation. In the TEM image as shown in Fig. 4b, nanoparticles with a size distribution of around 20 nm were observed. In addition, the formation of the spherical nanostructures was also demonstrated through the investigation using AFM, which revealed a size range of nanoparticles from 14 nm to 29 nm (Fig. S6, ESI†).
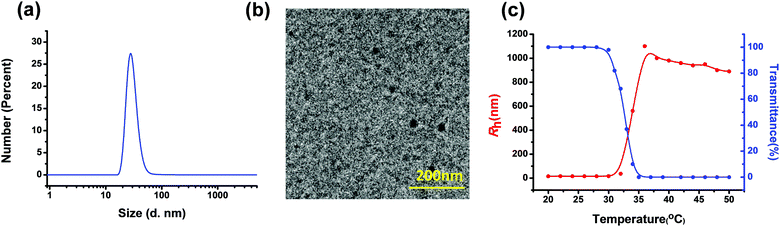 |
| Fig. 4 DLS data (a) and TEM image (b) of polymer 4 at 25 °C with a concentration of 1.0 mg mL−1. Transmittance (blue line) and hydrodynamic radius (red line) vs. temperature for polymer 4 (3.0 mg mL−1) in aqueous solution (c). | |
It is well known that PNIPAAM is a temperature-sensitive polymer, which can undergo a transition from a hydrated coil to dehydrated granules in water at a lower critical solution temperature (LCST) of about 32 °C.11 To study the LCST behaviour of polymer 4, an investigation on the change of transmittance at 500 nm was carried out by employing a temperature-controlled UV/vis spectrometer. According to the change of transmittance and the hydrodynamic size at different temperatures, a cloud point of 33 °C was determined, which is consistent with the LCST values of other PNIPAAM derivatives (Fig. 4c). In addition, variable temperature (VT) dynamic light scattering (DLS) was also performed to investigate the LCST behavior of polymer 4. As shown in Fig. 4c, a plot of the hydrodynamic radius (Rh) of polymer 4 in aqueous solution as a function of temperature, in the lower temperature region of 20 to 30 °C, the Rh values were relatively small and changed only slightly. When the temperature continued to increase, Rh increased gradually. But in the higher temperature ranges, the Rh values almost did not change. It should be noted that the critical temperature of phase transition obtained from the DLS investigation agreed with the result of transmittance measurements.
Next, the influence of temperature on the optical properties of polymer 4 was investigated. The fluorescence intensity of polymer 4 decreased along with the increase in temperature from 20 to 70 °C. It was found that in the temperature region of 20 to 30 °C, the value of I/I0 decreased slightly (Fig. 3c and d). When the temperature was increased above 30 °C, a more sharp change occurred, indicating the existence of disaggregation of the polymer at high temperature. It is worthy to note that the temperature of the mutation point approached the LCST measured by the UV/vis spectrometer. Thus, the obtained results indicated that AIE is a very efficient approach to probe the thermal transition of PNIPAAM in water in this study. Below the LCST, polymer 4 aggregated to form ordered nanoparticles driven by the amide–water H-bonding and hydrophobic effect, thus allowing for the strong fluorescence intensity. However, above the LCST, polymer 4 existed in a dehydrated shrunken state because amide–water H-bonds were destroyed. Then the TPE units were probably wrapped by polymer chains, resulting in fluorescence quenching.
The supra-amphiphilic property of star polymer 4 prompted us to study its potential biological application. Cytotoxicity assessment of polymer 4 is a crucial parameter for its future application as a biological material. In order to evaluate the cytotoxicity of polymer 4, the cell viability of polymer 4 on Bone Mesenchymal Stem Cells (BMSCs) using the MTT colorimetric assay was examined. From the result of the MTT assay, no obvious decrease in cell viability was observed even when the concentration of polymer 4 was increased to 1.28 mg mL−1 (Fig. S7, ESI†). From the above results, it was confirmed that polymer 4 featured good biocompatibility, thus allowing for its potential application as a biological material. Therefore, cell imaging was further investigated by using confocal laser scanning microscopy (CLSM). As shown in Fig. 5, polymer 4 was taken up by BMSCs with a concentration of 1.0 mg mL−1 for 6 h. The red fluorescence signal as displayed in Fig. 5a was attributed to the BMSCs. Notably, from the CLSM analysis, the green fluorescence signal arising from polymer 4 was observed in the same regions as the blue fluorescence signal from the DAPI stain (Fig. 5b and d). This finding indicated that polymer 4 was mainly located in the nucleus. We hypothesize that polymer 4 tended to accumulate in the cell nucleus due to the possible coordination between the N7 atoms of purine bases on DNA and the Pt centers according to a previous study on the interaction of platinum complexes with DNA.12
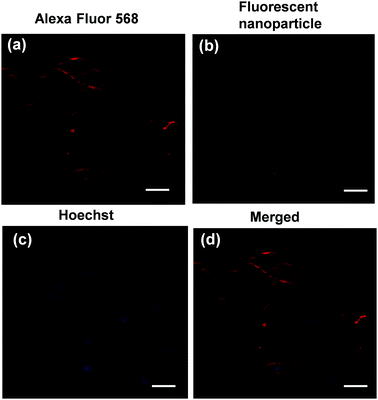 |
| Fig. 5 Confocal microscopy images of BMSCs incubated with the fluorescent nanoparticles of polymer 4 for 6 h. The cell cytoskeleton was stained for actin filament using Alexa Fluor 568 phalloidin (red). The cell nuclei were stained using DAPI (blue). Green fluorescence indicates the nanoparticles (scale bar = 50 μm). | |
Conclusions
In summary, we present a successful example of a TPE-based supra-amphiphilic metallacycle decorated with three PNIPAAM arms through combination of an exo-functionalization strategy and post-assembly polymerization. The obtained supra-amphiphilic metallosupramolecular architecture could spontaneously self-assemble into ordered nanoparticles in water with an obvious fluorescence emission even in dilute solutions due to the AIE effect. In particular, the obtained star polymer featured good biocompatibility and presented promising potential for application in the field of bioimaging. To the best of our knowledge, this study provides the first example of a TPE-based supra-amphiphilic metallacycle, thus providing a new route for producing functional metallosupramolecular architectures with potential application as biomaterials.
Experimental section
Full experimental details are provided in the ESI.† The most important information is summarized briefly below.
Self-assembly of supramolecular metallacycle 3 from 120° TPE containing dipyridyl donor 1 and 120° CTA-based di-Pt(II) acceptor 2
The dipyridyl donor ligand 1 (3.11 mg, 6.39 μmol) and the organoplatinum(II) acceptor 2 (9.41 mg, 6.39 μmol) were weighed accurately into a glass vial. To the vial was added 5.0 mL dichloromethane, and the reaction solution was then stirred at room temperature for 5 h to yield a homogeneous yellow solution. Then the addition of a saturated aqueous solution of KPF6 into the bottle with continuous stirring (10 minutes) precipitated the product. The reaction mixture was centrifuged, washed several times with water, and dried. Yellow solid product 3 was obtained by removing the solvent under vacuum. Yield: 11.2 mg, 90%. Mp: >285 °C, decomposed. 1H NMR (acetone-d6, 400 MHz): δ 8.92–8.93 (d, 4H, J = 6Hz), 8.06–8.07 (d, 4H, J = 6.4 Hz), 7.81 (s, 4H), 7.29–7.32 (t, 4H, J = 5.2 Hz), 7.20 (s, 6H) 7.12–7.14 (t, 4H, J = 3.6 Hz), 7.06 (s, 1H), 6.90 (s, 2H), 3.40–3.43 (t, 2H, J = 7.2 Hz), 1.82–196 (m, 35H), 1.20–1.28 (m, 65H), 0.86–0.87 (m, 7H); 31P NMR (acetone-d6, 161.9 MHz): δ 16.70 (s, JPt–P = 2430.0 Hz); IR (neat): 2922, 2851, 2119, 1748, 1612, 1567, 1489, 1330, 1142, 816, 734; ESI-TOF-MS of 4: calcd for [M − 6 PF6−]6+: 918.17, found: 918.06; calcd for [M − 5 PF6−]5+: 1130.60, found: 1330.74, calcd for [M − 4 PF6−]4+: 1449.74, found: 1449.88.
Synthesis of star supramolecular polymer 4
Supramolecular metallacycle 3 (40 mg, 6.1 μmol), AIBN (0.48 mg, 3.0 μmol), N-isopropylacrylamide (350 mg, 3.0 mmol), and 1.0 mL of acetone were added into a 10.0 mL flask equipped with a magnetic stirring bar. After being degassed by freeze–pump–thaw cycles three times, the mixed solution was immediately transferred to a preheated oil bath at 60 °C to initiate the polymerization. After 5 h, the polymerization was quenched by liquid N2, and the resultant mixture was precipitated in diethyl ether. The precipitate was dissolved in acetone and then precipitated again in the presence of diethyl ether. The above dissolution–precipitation cycle was repeated three times. The final product was dried under vacuum, as a yellow solid (210 mg, Mn,NMR = 15 kDa, Mn,GPC = 12 kDa, PDI = 1.20). 1H NMR (acetone-d6, 400 MHz): 6.98–7.77 (br s, –NH), 4.02 (br s, –NCH), 1.15–2.04 (br m, backbone), 0.83–0.88(s, –CH3 end-group); 31P NMR (acetone-d6, 161.9 MHz): δ 17.08 (s, JPt–P = 2436.7 Hz); IR (neat): 3289, 2973, 2367, 1640, 1544, 1460, 1387, 1367, 1171, 1131 cm−1.
Acknowledgements
Hai-Bo Yang thanks the NSFC/China (No. 21625202), the 973 Program (No. 2015CB856600), and STCSM (16XD1401000) for financial support. G. C. acknowledges the support of NSFC/China (No. 91527305, 21474020, 91227203, and 51322306).
Notes and references
-
(a) P. J. Stang and B. Olenyuk, Acc. Chem. Res., 1997, 30, 502–518 CrossRef CAS;
(b) M. Fujita, M. Tominaga, A. Hori and B. Therrien, Acc. Chem. Res., 2005, 38, 369–378 CrossRef CAS PubMed;
(c) D. Fiedler, D. H. Leung, R. G. Bergman and K. N. Raymond, Acc. Chem. Res., 2005, 38, 349–358 CrossRef CAS PubMed;
(d) A. M. Spokoyny, D. Kim, A. Sumrein and C. A. Mirkin, Chem. Soc. Rev., 2009, 38, 1218–1227 RSC;
(e) G. R. Newkome and C. Shreiner, Chem. Rev., 2010, 110, 6338–6442 CrossRef CAS PubMed;
(f) T. R. Cook and P. J. Stang, Chem. Rev., 2015, 115, 7001–7045 CrossRef CAS PubMed;
(g) M. Han, D. M. Engelhard and G. H. Clever, Chem. Soc. Rev., 2014, 43, 1848–1860 RSC;
(h) A. H. Clever and M. Shionoya, Coord. Chem. Rev., 2010, 254, 2391–2402 CrossRef;
(i) W. Wang, Y.-X. Wang, L.-J. Chen and H.-B. Yang, Chem. Soc. Rev., 2016, 45, 2656–2693 RSC.
-
(a) M. Fujita, J. Yazaki and K. Ogura, J. Am. Chem. Soc., 1990, 112, 5645–5647 CrossRef CAS;
(b) M. Fujita, D. Oguro, M. Miyazawa, H. Oka, K. Yamaguchi and K. Ogura, Nature, 1995, 378, 469–471 CrossRef CAS;
(c) P. J. Stang and D. H. Cao, J. Am. Chem. Soc., 1994, 116, 4981–4982 CrossRef CAS;
(d) B. Olenyuk, J. A. Whiteford, A. Fechtenkotter and P. J. Stang, Nature, 1999, 398, 796–799 CrossRef CAS PubMed;
(e) A. M. Brown, M. V. Ovchinnikov, C. L. Stern and C. A. Mirkin, J. Am. Chem. Soc., 2004, 126, 14316–14317 CrossRef CAS PubMed;
(f) P. Wang, C. N. Moorefield and G. R. Newkome, Angew. Chem., Int. Ed., 2005, 44, 1679–1683 CrossRef CAS PubMed;
(g) V. M. Dong, D. Fiedler, B. Carl, R. G. Bergman and K. N. Raymond, J. Am. Chem. Soc., 2006, 128, 14464–14465 CrossRef CAS PubMed;
(h) S. Hiraoka, M. Goda and M. Shionoya, J. Am. Chem. Soc., 2009, 131, 4592–4593 CrossRef CAS PubMed;
(i) B. Roy, A. K. Ghosh, S. Srivastava, P. D'Silva and P. S. Mukherjee, J. Am. Chem. Soc., 2015, 137, 11916–11919 CrossRef CAS PubMed;
(j) M. Yamashina, M. M. Sartin, Y. Sei, M. Akita, S. Takeuchi, T. Tahara and M. Yoshizawa, J. Am. Chem. Soc., 2015, 137, 9266–9269 CrossRef CAS PubMed;
(k) M. Han, R. Michel, B. He, Y.-S. Chen, D. Stalke, M. John and G. H. Clever, Angew. Chem., 2013, 125, 1358–1362 CrossRef;
(l) W. J. Ramsay, F. J. Rizzuto, T. K. Ronson, K. Capriceand and J. R. Nitschke, J. Am. Chem. Soc., 2016, 138, 7264–7267 CrossRef CAS PubMed;
(m) D. Fujita, Y. Ueda, S. Sato, H. Yokoyama, N. Mizuno, T. Kumasaka and M. Fujita, Chem. – Eur. J., 2016, 1, 91–101 CAS.
-
(a) B. H. Northrop, H.-B. Yang and P. J. Stang, Chem. Commun., 2008, 5896–5908 RSC;
(b) T. R. Cook, V. Vajpayee, M. H. Lee, P. J. Stang and K. Chi, Acc. Chem. Res., 2013, 46, 2464–2474 CrossRef CAS PubMed;
(c) M. L. Saha, X. Yan and P. J. Stang, Acc. Chem. Res., 2016, 49, 2527–2539 CrossRef CAS PubMed;
(d) L. Xu, Y.-X. Wang, L.-J. Chen and H.-B. Yang, Chem. Soc. Rev., 2015, 44, 2148–2167 RSC;
(e) A. J. McConnell, C. S. Wood, P. P. Neelakandan and J. R. Nitschke, Chem. Rev., 2015, 115, 7729–7793 CrossRef CAS PubMed;
(f) S. Shanmugaraju and P. S. Mukherjee, Chem. – Eur. J., 2015, 21, 6656–6666 CrossRef CAS PubMed;
(g) L. Xu, L.-J. Chen and H.-B. Yang, Chem. Commun., 2014, 50, 5156–5170 RSC;
(h) L. Xu, Y.-X. Wang and H.-B. Yang, Dalton Trans., 2015, 44, 867–890 RSC.
-
(a) L.-J. Chen, G.-Z. Zhao, B. Jiang, B. Sun, M. Wang, L. Xu, J. He, Z. Abliz, H. Tan, X. Li and H.-B. Yang, J. Am. Chem. Soc., 2014, 136, 5993–6001 CrossRef CAS PubMed;
(b) B. Jiang, J. Zhang, J.-Q. Ma, W. Zheng, L.-J. Chen, B. Sun, C. Li, B.-W. Hu, H. Tan, X. Li and H.-B. Yang, J. Am. Chem. Soc., 2016, 138, 738–741 CrossRef CAS PubMed;
(c) B. Sun, M. Wang, Z. Lou, M. Huang, C. Xu, X. Li, L.-J. Chen, Y. Yu, G. L. Davis, B. Xu, H.-B. Yang and X. Li, J. Am. Chem. Soc., 2015, 137, 1556–1564 CrossRef CAS PubMed;
(d) Z.-Y. Li, Y. Zhang, C.-W. Zhang, L.-J. Chen, C. Wang, H. Tan, Y. Yu, X. Li and H.-B. Yang, J. Am. Chem. Soc., 2014, 136, 8577–8589 CrossRef CAS PubMed;
(e) W. Wang, Y. Zhang, B. Sun, L.-J. Chen, X.-D. Xu, M. Wang, X. Li, Y. Yu, W. Jiang and H.-B. Yang, Chem. Sci., 2014, 5, 4554–4560 RSC;
(f) J.-K. Ou-Yang, Y.-Y. Zhang, M.-L. He, J.-T. Li, X. Li, X.-L. Zhao, C.-H. Wang, Y. Yu, D.-X. Wang, L. Xu and H.-B. Yang, Org. Lett., 2014, 16, 664–667 CrossRef CAS PubMed;
(g) G.-Z. Zhao, L.-J. Chen, W. Wang, J. Zhang, G. Yang, D. Wang, Y. Yu and H.-B. Yang, Chem. – Eur. J., 2013, 19, 10094–10100 CrossRef CAS PubMed;
(h) L.-J. Chen, B. Jiang and H.-B. Yang, Org. Chem. Front., 2016, 3, 579–587 RSC;
(i) B. Jiang, L.-J. Chen, Y. Zhang, H.-W. Tan, L. Xu and H.-B. Yang, Chin. Chem. Lett., 2016, 27, 607–612 CrossRef CAS.
-
(a) J. Shi, N. Chang, C. Li, J. Mei, C. Deng, X. Luo, Z. Liu, Z. Bo, Y. Q. Dong and B. Z. Tang, Chem. Commun., 2012, 48, 10675–10677 RSC;
(b) G. Liang, J. W. Y. Lam, W. Qin, J. Li, N. Xie and B. Z. Tang, Chem. Commun., 2014, 50, 1725–1727 RSC;
(c) N. Song, D.-X. Chen, Y.-C. Qiu, X.-Y. Yang, B. Xu, W. Tian and Y.-W. Yang, Chem. Commun., 2014, 50, 8231–8234 RSC;
(d) J. Wu, S. Sun, X. Feng, J. Shi, X.-Y. Hu and L. Wang, Chem. Commun., 2014, 50, 9122–9125 RSC;
(e) X. Wang, J. Hu, G. Zhang and S. Liu, J. Am. Chem. Soc., 2014, 136, 9890–9893 CrossRef CAS PubMed;
(f) Y. Lin, G. Chen, L. Zhao, W. Z. Yuan, Y. Zhang and B. Z. Tang, J. Mater. Chem. C, 2015, 3, 112–120 RSC.
-
(a) J. Luo, Z. Xie, J. W. Y. Lam, L. Cheng, H. Chen, C. Qiu, H. S. Kwork, X. Zhan, Y. Liu, D. Zhu and B. Z. Tang, Chem. Commun., 2001, 1740–1741 RSC;
(b) Y. Hong and J. W. Y. Lam, Chem. Commun., 2009, 4332–4353 RSC;
(c) H. Tong, Y. Hong, Y. Dong, M. Haeussler, J. W. Y. Lam, Z. Li, Z. Guo and B. Z. Tang, Chem. Commun., 2006, 3705–3707 RSC;
(d) M. Li, J. W. Y. Lam, F. Mahtab, S. Chen, W. Zhang, Y. Hong, J. Xiong, Q. Zheng and B. Z. Tang, J. Mater. Chem. B, 2013, 1, 676–684 RSC;
(e) P. Wang, X. Yan and F. Huang, Chem. Commun., 2014, 50, 5017–5019 RSC;
(f) G. Yu, G. Tang and F. Huang, J. Mater. Chem. C, 2014, 2, 6609–6617 RSC;
(g) S. Li, Y. Shang, E. Zhao, R. T. K. Kwok, J. W. Y. Lam, Y. Song and B. Z. Tang, J. Mater. Chem. C, 2015, 3, 3445–3451 RSC;
(h) M. Wang, X. Gu, G. Zhang, D. Zhang and D. Zhu, Anal. Chem., 2009, 81, 4444–4449 CrossRef CAS PubMed;
(i) X. Gu, G. Zhang, Z. Wang, W. Liu, L. Xiao and D. Zhang, Analyst, 2013, 138, 2427–2431 RSC.
-
(a) J. Mei, N. L. C. Leung, R. T. K. Kwok, J. W. Y. Lam and B. Z. Tang, Chem. Rev., 2015, 115, 11718–11940 CrossRef CAS PubMed;
(b) K. Li and B. Liu, Chem. Soc. Rev., 2014, 43, 6570–6597 RSC;
(c) Y. Yuan, R. T. Kwok, B. Z. Tang and B. Liu, J. Am. Chem. Soc., 2014, 136, 2546–2554 CrossRef CAS PubMed;
(d) Y. Yuan, C. Zhang, S. Xu and B. Liu, Chem. Sci., 2016, 7, 1862–1866 RSC;
(e) J. Liang, B. Tang and B. Liu, Chem. Soc. Rev., 2015, 44, 2798–2811 RSC;
(f) J. Mei, Y. Hong, J. W. Y. Lam, A. Qin, Y. Tang and B. Z. Tang, Adv. Mater., 2014, 26, 5429–5479 CrossRef CAS PubMed;
(g) Y. Liu, C. Deng, L. Tang, A. Qin, R. Hu, J. Z. Sun and B. Z. Tang, J. Am. Chem. Soc., 2011, 133, 660–663 CrossRef CAS PubMed;
(h) Z. Zhao, B. He, H. Nie, B. Chen, P. Lu, A. Qin and B. Z. Tang, Chem. Commun., 2014, 50, 1131–1133 RSC.
-
(a) X. Yan, T. R. Cook, P. Wang, F. Huang and P. J. Stang, Nat. Chem., 2015, 7, 342–348 CrossRef CAS PubMed;
(b) L.-J. Chen, Y.-Y. Ren, N.-W. Wu, B. Sun, J.-Q. Ma, L. Zhang, H. Tan, M. Liu, X. Li and H.-B. Yang, J. Am. Chem. Soc., 2015, 137, 11725–11735 CrossRef CAS PubMed;
(c) M. Zhang, S. Li, X. Yan, Z. Zhou, M. L. Saha, Y.-C. Wang and P. J. Stang, Proc. Natl. Acad. Sci. U. S. A., 2016, 113, 11100–11105 CrossRef CAS PubMed;
(d) Y. Tian, X. Yan, M. L. Saha, Z. Niu and P. J. Stang, J. Am. Chem. Soc., 2016, 138, 12033–12036 CrossRef CAS PubMed;
(e) Z. Zhou, X. Yan, M. L. Saha, M. Zhang, M. Wang, X. Li and P. J. Stang, J. Am. Chem. Soc., 2016, 138, 13131–13134 CrossRef CAS PubMed;
(f) X. Yan, H. Wang, C. E. Hauke, T. R. Cook, M. Wang, M. L. Saha, Z. Zhou, M. Zhang, X. Li, F. Huang and P. J. Stang, J. Am. Chem. Soc., 2015, 137, 15276–15286 CrossRef CAS PubMed;
(g) X. Yan, M. Wang, T. R. Cook, M. Zhang, M. L. Saha, Z. Zhou, X. Li, F. Huang and P. J. Stang, J. Am. Chem. Soc., 2016, 138, 4580–4588 CrossRef CAS PubMed.
-
(a) C. Wang, Z. Wang and X. Zhang, Acc. Chem. Res., 2012, 45, 608–618 CrossRef CAS PubMed;
(b) K. Liu, C. Wang, Z. Li and X. Zhang, Angew. Chem., Int. Ed., 2011, 50, 4952–4956 CrossRef CAS PubMed;
(c) Y. J. Jeon, P. K. Bharadwaj, S. W. Choi, J. W. Lee and K. Kim, Angew. Chem., Int. Ed., 2002, 41, 4474–4476 CrossRef CAS;
(d) Y. P. Wang, H. P. Xu and X. Zhang, Adv. Mater., 2009, 21, 2849–2864 CrossRef CAS;
(e) W. Tao, Y. Liu, B. Jiang, S. Yu, W. Huang, Y. Zhou and D. Yan, J. Am. Chem. Soc., 2012, 134, 762–764 CrossRef CAS PubMed;
(f) G. Yu, K. Jie and F. Huang, Chem. Rev., 2015, 115, 7240–7303 CrossRef CAS PubMed.
-
(a) N.-W. Wu, L.-J. Chen, C. Wang, Y.-Y. Ren, X. Li, L. Xu and H.-B. Yang, Chem. Commun., 2014, 50, 4231–4233 RSC;
(b) J. Zhang, R. Marega, L.-J. Chen, N.-W. Wu, X.-D. Xu, D. C. Muddiman, D. Bonifazi and H.-B. Yang, Chem. – Asian J., 2014, 9, 2928–2936 CrossRef CAS PubMed;
(c) W. Zheng, L.-J. Chen, G. Yang, B. Sun, X. Wang, B. Jiang, G.-Q. Yin, L. Zhang, X. Li, M. Liu, G. Chen and H.-B. Yang, J. Am. Chem. Soc., 2016, 138, 4927–4937 CrossRef CAS PubMed;
(d) S. Chen, L.-J. Chen, H.-B. Yang, H. Tian and W. Zhu, J. Am. Chem. Soc., 2012, 134, 13596–13599 CrossRef CAS PubMed.
-
(a) H. G. Schild, Prog. Polym. Sci., 1992, 17, 163–249 CrossRef CAS;
(b) H. Feil, Y. H. Bae, J. Feijen and S. W. Kim, J. Membr. Sci., 1991, 64, 283–294 CrossRef CAS;
(c) A. Gustafsson, H. Wennerstrom and F. Tjerneld, Fluid Phase Equilib., 1986, 29, 365–371 CrossRef CAS;
(d) S. B. La, T. Okano and K. Kataoka, J. Pharm. Sci., 1996, 85, 85–90 CrossRef CAS PubMed;
(e) A. Matsuyama and F. Tanaka, J. Chem. Phys., 1991, 94, 781–786 CrossRef CAS;
(f) C. Wu and S. Zhou, Macromolecules, 1995, 28, 5388–5390 CrossRef CAS;
(g) Y. Okada and F. Tanaka, Macromolecules, 2005, 38, 4465–4471 CrossRef CAS.
-
(a) D. Wang and S. J. Lippard, Nat. Rev. Drug Discovery, 2005, 4, 307–320 CrossRef CAS PubMed;
(b) G. Yu, T. R. Cook, Y. Li, X. Yan, D. Wu, L. Shao, J. Shen, G. Tang, F. Huang, X. Chen and P. J. Stang, Proc. Natl. Acad. Sci. U. S. A., 2016, 113, 13720–13725 CrossRef CAS PubMed.
Footnotes |
† Electronic supplementary information (ESI) available: Details of synthesis and characterization of the compounds, and supplementary experimental data and figures. See DOI: 10.1039/c7qm00107j |
‡ W. Zheng and G. Yang contributed equally. |
|
This journal is © the Partner Organisations 2017 |